DOI:
10.1039/D4SC01877J
(Edge Article)
Chem. Sci., 2024,
15, 8530-8535
A robust aluminum-octacarboxylate framework with scu topology for selective capture of sulfur dioxide†
Received
19th March 2024
, Accepted 30th April 2024
First published on 1st May 2024
Abstract
The high structural diversity and porosity of metal–organic frameworks (MOFs) promote their applications in selective gas adsorption. The development of robust MOFs that are stable against corrosive SO2 remains a daunting challenge. Here, we report a highly robust aluminum-based MOF (HIAM-330) built on a 4-connected Al3(OH)2(COO)4 cluster and 8-connected octacarboxylate ligand with a (4,8)-connected scu topology. It exhibits a fully reversible SO2 uptake of 12.1 mmol g−1 at 298 K and 1 bar. It is capable of selective capture of SO2 over other gases (CO2, CH4, and N2) with high adsorption selectivities of 60, 330, and 3537 for equimolar mixtures of SO2/CO2, SO2/CH4, and SO2/N2, respectively, at 298 K and 1 bar. Breakthrough measurements verified the capability of HIAM-330 for selective capture of SO2 (2500 ppm) over CO2 or N2. High-resolution synchrotron X-ray powder diffraction of SO2 loaded HIAM-330 revealed the binding domains of adsorbed SO2 molecules and host–guest interactions.
Introduction
By 2050, fossil fuels are predicted to account for 77% of global energy production, with coal emerging as the primary source in the global energy supply matrix.1 Exhaust gases from pulverized coal combustion consist of 10–12% CO2 and 500–3000 ppm SO2;2 the latter is a toxic and corrosive gas that will pose significant threats to human health and the environment.3,4 While current flue gas desulfurization (FGD) technologies can remove most SO2 from flue gases,5–7 they generate a tremendous amount of solid waste and suffer from high energy input. Achieving complete removal of trace SO2 proves challenging, as it necessitates a capture system with low selectivity for both dinitrogen and carbon dioxide coupled with remarkable material stability to withstand the highly corrosive nature of SO2.8,9
Selective capture of SO2 by dry, regenerable adsorbents under ambient conditions has attracted growing interest and is perceived as a promising strategy for eliminating trace SO2.10 This new method offers advantages such as lower energy consumption and minimized solid waste compared with the FGD strategy. Additionally, the recovered SO2 can be used to produce valuable chemicals such as sulfuric acid. While porous materials such as zeolites,8 activated carbon,11 and silica12 have been employed for SO2 capture, their adsorption capacity is generally low,13,14 limiting the overall capture efficiency. Metal–organic frameworks (MOFs) have garnered substantial attention for gas adsorption, due to their high porosity, structural diversity, precisely tunable pore dimensions, and highly customizable functionality.9,15,16 However, to date, only a limited number of MOFs have exhibited reversible SO2 adsorption and structural stability upon adsorption–desorption cycles.17–19 This is due to the highly corrosive nature of SO2, which can cause framework degradation in many MOFs.1,20–22 Thus, the ability of a MOF to maintain its structural integrity and retain its adsorption capacity after repeated cycles of SO2 adsorption and desorption is a crucial factor for its practical application in desulfurization processes. Furthermore, highly stable and crystalline MOFs can enable the study of host–guest interactions using in situ X-ray diffraction techniques to understand the underlying mechanism of selective adsorption. To this end, the development of MOFs with reversible SO2 adsorption and excellent structural stability remains a challenging and important area of research.
Over the past few years, Al-MOFs and Zr-MOFs have demonstrated superior efficacy in the adsorption of SO2, underpinned by their high stability.23,24 Their framework robustness arises not only from the strong Al–O and Zr–O bonds but also from the highly connected multinuclear inorganic clusters.25 The ability of these clusters to link with multiple ligands bolsters the stability of the MOFs. Using highly connected organic ligands to synthesize MOFs is an effective strategy for improving framework stability. Thus, the combination of multinuclear inorganic building blocks and multi-topic organic linkers usually leads to MOFs with intriguing connectivity and topology. However, the construction of MOFs from high valence metals (Al3+, Zr4+, Ti4+, etc.) and organic linkers with high connectivity (>6) is particularly challenging due to the inherent synthetic difficulties. For example, only a handful of Zr-MOFs built on hexacarboxylates and octacarboxylates26–30 have been reported, while only a single example of Al-MOF incorporating hexacarboxylates exists.31 Yet, no examples of Al-MOFs made of an octacarboxylate linker have been reported to date.
Here, we report the incorporation of an octacarboxylate, 4′,4′′′,4′′′′′,4′′′′′′′-(ethene-1,1,2,2-tetrayl)tetrakis(([1,1′-biphenyl]-3,5-dicarboxylic acid)) (H8ettbpdc) into a robust aluminum-based MOF, denoted as HIAM-330. It features a 4,8-c scu topology, built on 4-connected Al3O2(COO)4 clusters and 8-connected ettbpdc8− linkers. The structure contains two distinct types of cavities and demonstrates a SO2 adsorption capacity of 12.1 mmol g−1 at 298 K and 1 bar. The adsorption was fully reversible and the adsorption capacity was retained after multiple SO2 adsorption/desorption cycles. HIAM-330 exhibits selective capture of SO2 in the presence of CO2 and N2. Molecular insights into the selective adsorption mechanism were achieved through X-ray diffraction analysis on SO2-loaded HIAM-330, which elucidated the binding domains and host–guest interactions.
Results and discussion
H8ettbpdc was selected as the organic building block for constructing the Al-octacarboxylate MOF due to its well-defined rigidity, planar geometry and facile synthesis. It was synthesized from 1,1,2,2-tetrakis(4-bromophenyl)ethylene via the Suzuki coupling reaction (Fig. S1–S3†). Subsequently, block-shaped crystals of HIAM-330 were solvothermally synthesized at 150 °C by reacting Al(NO3)3·9H2O and H8ettbpdc in DMF/formic acid/acetonitrile mixed solvent (Fig. S5†). Single-crystal X-ray diffraction analysis revealed that it crystallized in the tetragonal crystal system with a space group of I4/mmm (Table S1†). The crystal structure of HIAM-330 is built on a trinuclear Al3O2(COO)4(H2O)6 cluster. In each cluster, the central Al3+ is octahedrally coordinated to four carboxylate oxygen atoms and two bridging oxygen atoms, while the terminal Al3+ is octahedrally connected to two carboxylate oxygen atoms, one bridging oxygen atom, and three water molecules (Fig. 1 and S6†). Thus, the trinuclear Al3 clusters act as 4-connected nodes in the structure of HIAM-330, bridged by ettbpdc8− organic struts. It is noteworthy that the Al3 cluster observed here is distinct from the commonly observed triangular trinuclear Al3 SBU, where a μ3-O connects three Al3+ to form a 6-connected node.25 The octacarboxylate organic linker ettbpdc8− is fully deprotonated and connected to sixteen Al3+ centers from eight Al3 clusters, with all carboxylates coordinated in bidentate mode (Fig. S7†). The overall structure of HIAM-330 features 4,8-c scu topology. It is intriguing that its connectivity is in sharp contrast to that of previously reported scu Zr-MOFs. The latter, as exemplified in PCN-606,32 is constructed on 8-connected Zr6 nodes and 4-connected organic linkers (usually tetracarboxylates, Fig. 1). Nevertheless, in HIAM-330, the Al3 clusters serve as the 4-connected nodes and the organic ligands act as the 8-connected units. This also leads to a different pore structure. The commonly observed scu-type Zr-MOFs such as PCN-606 or Zr-abtc possess one-dimensional rhombic channels, derived from the cubic cages of 4,12-c ftw-type connectivity.33 In contrast, in HIAM-330, there are two distinct types of cages, with large and small cages arrayed in alternating sequences (Fig. 1 and S8†).
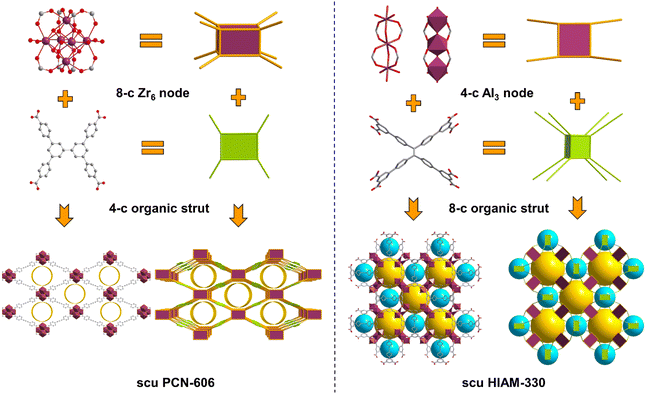 |
| Fig. 1 Building blocks and crystal structure of PCN-660 (left) and HIAM-330 (right). | |
The phase purity of HIAM-330 was verified through powder X-ray diffraction (PXRD) analysis (Fig. 2a). Thermogravimetric analysis (TGA) of as-synthesized HIAM-330 displayed a continuous weight loss, while that of the methanol-exchanged sample showed a plateau from 120–270 °C, indicating the successful exchange of high boiling point solvents by methanol (Fig. S9†). During the subsequent activation of the methanol-exchanged HIAM-330 by heating at 150 °C, its crystal structure was fully retained. Our experimental investigation suggests that HIAM-330 exhibits excellent stability, as evidenced by its fully preserved PXRD patterns after being heated at 150 °C in open air for 1 week, immersed in water at 80 °C for 1 week, or exposed to 90% humidity for 1 week (Fig. 2a). The permanent porosity of HIAM-330 was evaluated by N2 adsorption measurements at 77 K (Fig. 2b). The N2 isotherm displays a typical type I profile, yielding a BET surface area of 1624 m2 g−1 and a pore volume of 0.65 m3 g−1 (Fig. S10†). The pore size distribution curve, determined using the NLDFT model, was centered at approximately 5.5, 8.5, and 13.0 Å (Fig. S11†), which is consistent with the value estimated from Zeo++ software based on the crystal structure (Fig. S12†).
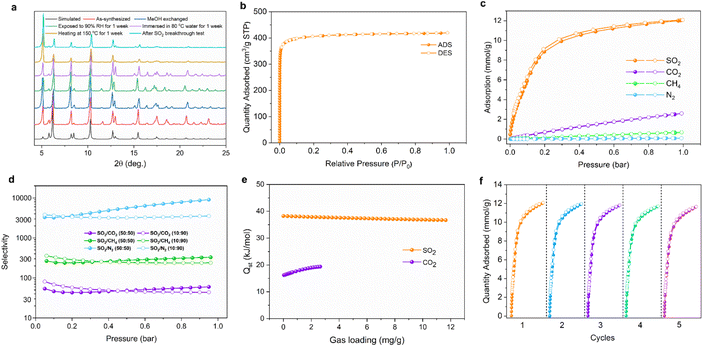 |
| Fig. 2 Gas adsorption on HIAM-330. (a) PXRD patterns of HIAM-330 under various conditions. (b) Adsorption–desorption isotherms of N2 at 77 K by HIAM-330. (c) Adsorption isotherms of SO2, CO2, CH4, and N2 at 298 K. (d) IAST selectivities of SO2/CO2, SO2/CH4, and SO2/N2 at 298 K in HIAM-330. (e) Qst curves for SO2 and CO2 in HIAM-330. (f) Five adsorption–desorption cycles for SO2 in HIAM-330 at 298 K and 1 bar. | |
Single-component adsorption isotherms of SO2 were collected at 298, 283, and 273 K (Fig. S13†). HIAM-330 exhibits fully reversible adsorption for SO2 with a high adsorption uptake of 12.1 mmol g−1 at 298 K and 1 bar. This value is higher than those of most of the MOFs studied for SO2 capture, such as Zr-bptc, UiO-66, UiO-66–NH2, and Zr-DMTDC,24 but is lower than those of the recently reported MFM-190 series showing higher porosity34 (Fig. S14†). Furthermore, the adsorption uptake increased dramatically at relatively low pressure, indicating its ability to capture trace SO2. The calculated isosteric heat of adsorption (Qst) is 38.0 kJ mol−1 at zero coverage. To study the capability of HIAM-330 for selective capture of SO2 from other light gases that often co-exist in the flue-gas stream, its adsorption towards CO2, CH4, and N2 was also evaluated. HIAM-330 adsorbs 2.60 mmol g−1 of CO2, 0.68 mmol g−1 of CH4, and 0.09 mmol g−1 of N2 at 1 bar and 298 K, substantially lower than that of SO2 (Fig. 2c). The Qst of CO2 calculated from adsorption isotherms at 298, 283, and 273 K is 16 kJ mol−1 at zero coverage, indicating its notably weaker adsorption affinity compared to that of SO2 (Fig. 2e). The selectivities of HIAM-330 for SO2/CO2, SO2/N2 and SO2/CH4 were calculated via ideal adsorbed solution theory (IAST) at 298 K (Fig. 2d). Due to the negligible adsorption capacity for nitrogen, the calculated SO2/N2 selectivity values are unusually high (>1000) and are subject to large uncertainties. HIAM-330 also shows high selectivity values of 60 and 330 for equimolar binary mixtures of SO2/CO2 and SO2/CH4, respectively at 298 K and a total pressure of 1 bar. The values remain similar when the concentration of SO2 decreased to 10%, resulting in selectivities of 44 and 239 for SO2/CO2 and SO2/CH4, respectively. These results suggest the preferential adsorption of SO2 over other light gases by HIAM-330.
While many MOF materials exhibit high adsorption capacities for SO2 due to high porosity, only a small number of them show fully reversible adsorption and retain their crystallinity when exposed to highly corrosive SO2. HIAM-330 demonstrates the ability to maintain its structure after undergoing SO2 adsorption–desorption tests (Fig. 2a). In consideration of practical applications, cyclic adsorption–desorption experiments were also conducted. In five consecutive adsorption/desorption cycles, no notable loss of SO2 adsorption capacity (<5%) was observed for HIAM-330 (Fig. 2f), further confirming its high stability to SO2.
To further verify the ability of HIAM-330 for selective capture of trace SO2, dynamic breakthrough experiments were conducted using a fixed bed filled with HIAM-330. At 298 K and 1 bar, a mixture of SO2/N2 (2500 ppm SO2, 75% N2 diluted in He) was passed through the column at a flow rate of 20 mL min−1. The results showed that N2 eluted at the beginning of the process, while SO2 was retained in the column for more than three hours (Fig. 3a). Additionally, the calculated SO2/N2 separation factor was 745. Similarly, another column breakthrough measurement was performed with a mixture of SO2/CO2 (2500 ppm SO2, 15% CO2 diluted in He). CO2 broke through within less than two minutes, while SO2 was retained for 136 minutes (Fig. 3b). The separation factor of SO2/CO2 was calculated to be 65.5. These findings validated the capability of HIAM-330 for selective capture of trace SO2 from other light gases as a durable adsorbent.
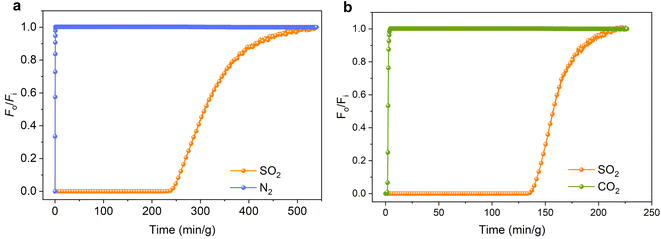 |
| Fig. 3 Separation of SO2, N2 and CO2 by HIAM-330: breakthrough plots for (a) the SO2/N2 mixture (2500 ppm SO2, 75% N2 in He, and total flow rate: 20 mL min−1) and (b) the SO2/CO2 mixture (2500 ppm SO2, 15% CO2 in He, and total flow rate: 20 mL min−1) in HIAM-330 at 298 K. | |
The adsorption domains of SO2 in HIAM-330 were determined by Rietveld refinements of the high-resolution synchrotron PXRD pattern of SO2-loaded HIAM-330 (Fig. S22†). The robust framework of HIAM-330 remained highly crystalline, allowing direct visualization of the adsorbed SO2 molecules in the pores. It was revealed that there were seven SO2 binding sites in the pores, with one in the small tetrahedral cage (Fig. 4a), two in the large octahedral cage (Fig. 4b), and four in the small pocket surrounded by eight trinuclear Al3 clusters (Fig. 4c). The total crystallographic uptake of SO2 was estimated to be 7.35 mmol g−1, corresponding to a crystal structure at a relatively low SO2 pressure of ∼0.14 bar. It is interesting to observe that most of the adsorbed SO2 molecules are located nearby the inorganic nodes rather than aggregating in the two main cages. This indicates that the Al3 clusters are favorable binding sites for SO2 at low pressure. The SO2 molecule at site I was stabilized by the organic linker through hydrogen bonds (OSO⋯H–C = 2.44 Å and O2S⋯H–C = 2.36 Å). At site III, the adsorbed SO2 was immobilized by the phenyl ring (OSO⋯pi = 2.64 Å, electrostatic interactions) and by one hydrogen bond (OSO⋯H–C = 2.16 Å). In the remaining five sites (sites II and IV–VII), the adsorbed SO2 molecules were predominantly interacting with the Al3 cluster (the bridging oxygen or the terminal H2O) through dipole–dipole interactions with the shortest OSO⋯O and O2S⋯O distances of 1.97 and 1.91 Å, respectively. The above results offer molecular insights into the guest–host interaction of SO2 adsorption in HIAM-330 and highlight the important role of the trinuclear Al3 cluster in SO2 capture.
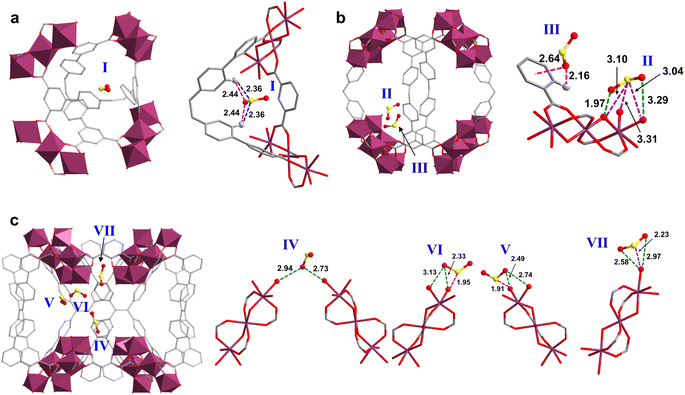 |
| Fig. 4 Adsorption domains of SO2 in HIAM-330 determined by Rietveld refinements of high-resolution in situ synchrotron powder X-ray diffraction. (a) site I, (b) sites II and III, (c) sites IV–VII. | |
Conclusions
The design and synthesis of highly porous and stable MOFs with multi-topic ligands and high valence metals is important for enriching MOF structure diversity, adsorption, and capture of corrosive gases such as SO2. We present the construction of an intriguing 4,8-c scu-type Al-MOF using a rigid octacarboxylate linker. The resulting MOF, HIAM-330, exhibits high porosity, excellent stability, high SO2 uptake (12.08 mmol g−1 at 298 K and 1 bar), and high IAST SO2/CO2 selectivity (60, SO2/CO2 = 50/50). Mixed-gas column breakthrough experiments further confirmed the effective removal of trace SO2 by HIAM-330 in the presence of CO2 and N2. The excellent chemical and thermal stability of HIAM-330 endows it with good recyclability. Further, Rietveld refinement of high-resolution PXRD patterns of SO2-loaded HIAM-330 provides valuable information regarding SO2 adsorption sites and possible guest–host interactions, which is important for understanding the adsorption mechanism. Overall, our findings contribute to rational design strategies for making stable MOFs that hold strong promise for effective capture of target molecules.
Data availability
Data associated to the article are available in the ESI.†
Author contributions
Liang Yu: investigation, methodology, and writing – original draft. Meng He: investigation and methodology. Jinze Yao: investigation and validation. Qibin Xia: conceptualization, supervision, project administration, and writing – review & editing. Sihai Yang: conceptualization, project administration, supervision, and writing – review & editing. Jing Li: conceptualization, supervision, project administration, and writing – review & editing. Hao Wang: conceptualization, supervision, and writing – review & editing.
Conflicts of interest
The authors declare that they have no competing interests.
Acknowledgements
This work was supported financially by the Shenzhen Science and Technology Program (No. KCXFZ20211020163818026) and National Natural Science Foundation of China (22178119). Work in the US was supported by the U.S. Department of Energy, Office of Science, Office of Basic Energy Sciences under Award No. DE-SC0019902.
Notes and references
- J. H. Carter, X. Han, F. Y. Moreau, I. da Silva, A. Nevin, H. G. W. Godfrey, C. C. Tang, S. Yang and M. Schröder, Exceptional Adsorption and Binding of Sulfur Dioxide in a Robust Zirconium-Based Metal-Organic Framework, J. Am. Chem. Soc., 2018, 140, 15564–15567 CrossRef CAS PubMed
.
- M. Savage, Y. Cheng, T. L. Easun, J. E. Eyley, S. P. Argent, M. R. Warren, W. Lewis, C. Murray, C. C. Tang, M. D. Frogley, G. Cinque, J. Sun, S. Rudić, R. T. Murden, M. J. Benham, A. N. Fitch, A. J. Blake, A. J. Ramirez-Cuesta, S. Yang and M. Schröder, Selective Adsorption of Sulfur Dioxide in a Robust Metal-Organic Framework Material, Adv. Mater., 2016, 28, 8705–8711 Search PubMed
.
- M. Kampa and E. Castanas, Human health effects of air pollution, Environ. Pollut., 2008, 151, 362–367 Search PubMed
.
- N. Greenberg, R. Carel and B. A. Portnov, Air Pollution and Respiratory Morbidity in Israel: A Review of Accumulated Empiric Evidence, Isr. Med. Assoc. J., 2015, 17, 445–450 Search PubMed
.
- G. T. Rochelle, Amine scrubbing for CO2 capture, Science, 2009, 325, 1652–1654 CrossRef CAS PubMed
.
- G. Cui, J. Wang and S. Zhang, Active chemisorption sites in functionalized ionic liquids for carbon capture, Chem. Soc. Rev., 2016, 45, 4307–4339 RSC
.
- R. K. Srivastava and W. Jozewicz, Flue gas desulfurization: the state of the art, J. Air Waste Manag. Assoc., 2001, 51, 1676–1688 Search PubMed
.
- I. Matito-Martos, A. Martin-Calvo, J. J. Gutiérrez-Sevillano, M. Haranczyk, M. Doblare, J. B. Parra, C. O. Ania and S. Calero, Zeolite screening for the separation of gas mixtures containing SO2, CO2 and CO, Phys. Chem. Chem. Phys., 2014, 16, 19884–19893 Search PubMed
.
- D. M. D'Alessandro, B. Smit and J. R. Long, Carbon dioxide capture: prospects for new materials, Angew. Chem., Int. Ed., 2010, 49, 6058–6082 Search PubMed
.
- G. L. Smith, J. E. Eyley, X. Han, X. Zhang, J. Li, N. M. Jacques, H. G. W. Godfrey, S. P. Argent, L. J. M. McPherson, S. J. Teat, Y. Cheng, M. D. Frogley, G. Cinque, S. J. Day, C. C. Tang, T. L. Easun, S. Rudić, A. J. Ramirez-Cuesta, S. Yang and M. Schröder, Reversible coordinative binding and separation of sulfur dioxide in a robust metal-organic framework with open copper sites, Nat. Mater., 2019, 18, 1358–1365 CrossRef CAS PubMed
.
- X. Zhou, H. Yi, X. Tang, H. Deng and H. Liu, Thermodynamics for the adsorption of SO2, NO and CO2 from flue gas on activated carbon fiber, Chem. Eng. J., 2012, 200–202, 399–404 CrossRef CAS
.
- Z. Zhang, L. Wu, J. Dong, B.-G. Li and S. Zhu, Preparation and SO2 Sorption/Desorption Behavior of an Ionic Liquid Supported on Porous Silica Particles, Ind. Eng. Chem. Res., 2009, 48, 2142–2148 CrossRef CAS
.
- E. Raymundo-Piñero, D. Cazorla-Amorós, C. Salinas-Martinez de Lecea and A. Linares-Solano, Factors controling the SO2 removal by porous carbons: relevance of the SO2 oxidation step, Carbon, 2000, 38, 335–344 CrossRef
.
- Y. Mathieu, L. Tzanis, M. Soulard, J. Patarin, M. Vierling and M. Molière, Adsorption of SOx by oxide materials: a review, Fuel Process. Technol., 2013, 114, 81–100 CrossRef CAS
.
- X. Lin, N. R. Champness and M. Schröder, Hydrogen, methane and carbon dioxide adsorption in metal-organic framework
materials, Top. Curr. Chem., 2010, 293, 35–76 CrossRef CAS PubMed
.
- Q. Li, W. Zhang, O. S. Miljanić, C. H. Sue, Y. L. Zhao, L. Liu, C. B. Knobler, J. F. Stoddart and O. M. Yaghi, Docking in metal-organic frameworks, Science, 2009, 325, 855–859 CrossRef CAS PubMed
.
- T. G. Glover, G. W. Peterson, B. J. Schindler, D. Britt and O. Yaghi, MOF-74 building unit has a direct impact on toxic gas adsorption, Chem. Eng. Sci., 2011, 66, 163–170 CrossRef
.
- K. Tan, N. Nijem, P. Canepa, Q. Gong, J. Li, T. Thonhauser and Y. J. Chabal, Stability and Hydrolyzation of Metal Organic Frameworks with Paddle-Wheel SBUs upon Hydration, Chem. Mater., 2012, 24, 3153–3167 CrossRef CAS
.
- G. L. Smith, J. E. Eyley, X. Han, X. Zhang, J. Li, N. M. Jacques, H. G. W. Godfrey, S. P. Argent, L. J. M. McPherson, S. J. Teat, Y. Cheng, M. D. Frogley, G. Cinque, S. J. Day, C. C. Tang, T. L. Easun, S. Rudić, A. J. Ramirez-Cuesta, S. Yang and M. Schröder, Reversible coordinative binding and separation of sulfur dioxide in a robust metal–organic framework with open copper sites, Nat. Mater., 2019, 18, 1358–1365 CrossRef CAS PubMed
.
- S. Yang, J. Sun, A. J. Ramirez-Cuesta, S. K. Callear, W. I. David, D. P. Anderson, R. Newby, A. J. Blake, J. E. Parker, C. C. Tang and M. Schröder, Selectivity and direct visualization of carbon dioxide and sulfur dioxide in a decorated porous host, Nat. Chem., 2012, 4, 887–894 CrossRef CAS PubMed
.
- S. M. Cohen, Postsynthetic Methods for the Functionalization of Metal–Organic Frameworks, Chem. Rev., 2012, 112, 970–1000 CrossRef CAS PubMed
.
- T. Islamoglu, S. Goswami, Z. Li, A. J. Howarth, O. K. Farha and J. T. Hupp, Postsynthetic Tuning of Metal–Organic Frameworks for Targeted Applications, Acc. Chem. Res., 2017, 50, 805–813 Search PubMed
.
- L. Li, I. da Silva, D. I. Kolokolov, X. Han, J. Li, G. Smith, Y. Cheng, L. L. Daemen, C. G. Morris, H. G. W. Godfrey, N. M. Jacques, X. Zhang, P. Manuel, M. D. Frogley, C. A. Murray, A. J. Ramirez-Cuesta, G. Cinque, C. C. Tang, A. G. Stepanov, S. Yang and M. Schroder, Post-synthetic modulation of the charge distribution in a metal-organic framework for optimal binding of carbon dioxide and sulfur dioxide, Chem. Sci., 2019, 10, 1472–1482 RSC
.
- J. Li, G. L. Smith, Y. Chen, Y. Ma, M. Kippax-Jones, M. Fan, W. Lu, M. D. Frogley, G. Cinque, S. J. Day, S. P. Thompson, Y. Cheng, L. L. Daemen, A. J. Ramirez-Cuesta, M. Schröder and S. Yang, Structural and Dynamic Analysis of Sulphur Dioxide Adsorption in a Series of Zirconium-Based Metal-Organic Frameworks, Angew. Chem., Int. Ed., 2022, 61, e202207259 CrossRef CAS PubMed
.
- W. Fan, K.-Y. Wang, C. Welton, L. Feng, X. Wang, X. Liu, Y. Li, Z. Kang, H.-C. Zhou, R. Wang and D. Sun, Aluminum metal–organic frameworks: from structures to applications, Coord. Chem. Rev., 2023, 489, 215175 Search PubMed
.
- W. Liu, E. Wu, B. Yu, Z. Liu, K. Wang, D. Qi, B. Li and J. Jiang, Reticular Synthesis of Metal-Organic Frameworks by 8-Connected Quadrangular Prism Ligands for Water Harvesting, Angew. Chem., Int. Ed., 2023, 62, e202305144 CrossRef CAS PubMed
.
- B. Ortín-Rubio, C. Perona-Bermejo, J. A. S. D. Pino, F. J. Carmona, F. Gándara, J. A. R. Navarro, J. Juanhuix, I. Imaz and D. Maspoch, A mesoporous Zr-based metal-organic framework driven by the assembly of an octatopic linker, Chem. Commun., 2023, 59, 7803–7806 RSC
.
- P. T. K. Nguyen, H. T. D. Nguyen, H. N. Nguyen, C. A. Trickett, Q. T. Ton, E. Gutiérrez-Puebla, M. A. Monge, K. E. Cordova and F. Gándara, New Metal-Organic Frameworks for Chemical Fixation of CO(2), ACS Appl. Mater. Interfaces, 2018, 10, 733–744 CrossRef CAS PubMed
.
- Z. Chen, P. Li, X. Wang, K.-i. Otake, X. Zhang, L. Robison, A. Atilgan, T. Islamoglu, M. G. Hall, G. W. Peterson, J. F. Stoddart and O. K. Farha, Ligand-Directed Reticular Synthesis of Catalytically Active Missing Zirconium-Based Metal–Organic Frameworks, J. Am. Chem. Soc., 2019, 141, 12229–12235 CrossRef CAS PubMed
.
- D. Alezi, I. Spanopoulos, C. Tsangarakis, A. Shkurenko, K. Adil, Y. Belmabkhout, M. O. Keeffe, M. Eddaoudi and P. N. Trikalitis, Reticular Chemistry at Its Best: Directed Assembly of Hexagonal Building Units into the Awaited Metal-Organic Framework with the Intricate Polybenzene Topology, pbz-MOF, J. Am. Chem. Soc., 2016, 138, 12767–12770 CrossRef CAS PubMed
.
- Z. Chen, P. Li, R. Anderson, X. Wang, X. Zhang, L. Robison, L. R. Redfern, S. Moribe, T. Islamoglu, D. A. Gómez-Gualdrón, T. Yildirim, J. F. Stoddart and O. K. Farha, Balancing volumetric and gravimetric uptake in highly porous materials for clean energy, Science, 2020, 368, 297–303 CrossRef CAS PubMed
.
- J. Pang, S. Yuan, J. Qin, C. Liu, C. Lollar, M. Wu, D. Yuan, H. C. Zhou and M. Hong, Control the Structure of Zr-Tetracarboxylate Frameworks through Steric Tuning, J. Am. Chem. Soc., 2017, 139, 16939–16945 CrossRef CAS PubMed
.
- H. Wang, X. Dong, J. Lin, S. J. Teat, S. Jensen, J. Cure, E. V. Alexandrov, Q. Xia, K. Tan, Q. Wang, D. H. Olson, D. M. Proserpio, Y. J. Chabal, T. Thonhauser, J. Sun, Y. Han and J. Li, Topologically guided tuning of Zr-MOF pore structures for highly selective separation of C6 alkane isomers, Nat. Commun., 2018, 9, 1745 CrossRef PubMed
.
- W. Li, J. Li, T. D. Duong, S. A. Sapchenko, X. Han, J. D. Humby, G. F. S. Whitehead, I. J. Victórica-Yrezábal, I. da Silva, P. Manuel, M. D. Frogley, G. Cinque, M. Schröder and S. Yang, Adsorption of Sulfur Dioxide in Cu(II)-Carboxylate Framework Materials: The Role of Ligand Functionalization and Open Metal Sites, J. Am. Chem. Soc., 2022, 144, 13196–13204 CrossRef CAS PubMed
.
|
This journal is © The Royal Society of Chemistry 2024 |
Click here to see how this site uses Cookies. View our privacy policy here.