DOI:
10.1039/D4RA03131H
(Paper)
RSC Adv., 2024,
14, 20105-20112
Fabrication of thermo-responsive multicore microcapsules using a facile extrusion process†
Received
27th April 2024
, Accepted 16th June 2024
First published on 24th June 2024
Abstract
A process employing extrusion was used to produce multicore microcapsules composed of multiple beads. The inner beads were made from κ-carrageenan (κ-c), a thermo-responsive linear sulphated polymer whose gelling temperature ranges at 40–60 °C, depending on the concentration of κ-c polymer and the amount of potassium chloride used for gelation. The resulting beads were then enveloped by chitosan through gelation with sodium triphosphate. The pesticide ammonium glufosinate was encapsulated in the κ-c/chitosan multicore microcapsules for demonstration of controlled release of the encapsulant. It was found that in response to an external stimulus, such as elevated temperature or solar simulation, the microcapsules exhibit the gradual release of encapsulated pesticide molecules from multicore microcapsules, compared with beads only. This process of making multicore microcapsules can be extended to other polymer pairs based on applications. This work is relevant to agriculture, where the controlled-release of the pesticides or fertilizers could be triggered by the sun and/or temperature changes, thus extending the residual period of the chemicals as well as decreasing the extent of pollution by leaching of abundant chemicals.
1. Introduction
Polysaccharide-based microparticles have been extensively studied as carriers for hydrophobic therapeutic agents.1,2 The latter are usually incorporated into microparticles through encapsulation – a technique in which one material or a mixture of materials is entrapped within another substance and thus the encapsulated materials could be protected from external environments. Depending on the form, the encapsulated substance is referred to as the core, fill, internal or payload phase and the substance that encapsulates is referred to as the coating membrane, shell, capsule, carrier material, external phase or matrix.3 Encapsulation is commercially employed in agriculture, food, pharma, textile and biotechnology industries to create high-value end products.4–8 The materials used for the membrane and the encapsulation technique determine the effectiveness of the operation and significantly influence outputs such as encapsulation efficiency, particle size distribution, system feeding rate, and system rheology.9 For instance, there is a growing demand for crop production, which has been fueled by the rising human population, changing consumption habits, climate change and decrease in arable land. It has been projected that these factors will necessitate a doubling of agricultural outputs by 2050.10 So far, this increase in food production has been partly addressed through improved crop germplasm and the usage of agrochemicals such as fungicides, pesticides and fertilizers. However, this has led to an unsustainable surge in agrochemicals that does not equate to a continuous improvement of crop yields, and has a negative impact on human health and the environment.11 Encapsulation of agricultural chemicals could help in finding a balance between crop losses and the environmental impacts of chemical applications.
The common encapsulation methods are spray drying and extrusion, due to their scalability and application in industries. Spray-drying is a flexible, continuous, scalable and economically viable operation12,13 and thus is widely used to transform a liquid into a dried particulate.2 It entails the heating and atomization of a polymer solution into small droplets, which are quickly dried to form solid particles with the average diameter to be <40 μm. On the other hand, extrusion is a process in which a hydrocolloid is passed through a nozzle-like opening to generate droplets that can give rise to a bead or capsule.14 A bead is formed when the shell-forming polymer solution is mixed with the core-forming solution or the encapsulant.15 There is no specific phase or delimitation since the encapsulant permeates the whole droplet, which in turn can be hardened in a gelling bath to form (or produce) a three-dimensional grid via cross-linking of the polymer. Extrusion can also be used to produce capsules, where the encapsulant is completely entrapped by a shell.5 Capsules can also be fabricated by coextrusion, a simultaneous extrusion process of two immiscible liquids through a concentric nozzle.16–18 The hardening process is similar to forming beads by regular extrusion with the underlying principle that if a material can form a bead with a certain material, it can likely be used as a shell for capsules. To facilitate the formation of capsules with clear separation between cores and shells, the components can be made from non-miscible materials, giving rise to capsules with a liquid oil-based core and an aqueous polymer gel as a shell.
Sodium alginate and chitosan have been exploited as the most promising materials to form microcapsules using various methods such as spray-drying, extrusion, and chemical modification.19 Alginate is an anionic natural polysaccharide containing β-(1,4)-D-mannuronic acid and α-(1,4)-L-guluronic acid monomers (Fig. 1).20 It is biocompatible, environmentally benign, biodegradable and economically favorable with unique physical and chemical properties, enabling its application in various interdisciplinary areas.21,22 In addition, alginate is a negatively charged polyelectrolyte and therefore, possess significant benefits for encapsulation and targeted delivery of therapeutics.21,22 Chitosan is a positively charged biodegradable polysaccharide, obtained by the deacetylation of chitin (Fig. 1).23 The latter is commonly found in the exoskeletons of insects and crustaceans and can be sustainably sourced from seafood waste. Chitosan possesses antibacterial activities (due to the presence of pendant amine groups) and has been used to prepare hydrogels, films, fibres and sponges targeted towards biomedical applications.24,25 Microencapsulation is far from limited to alginate and chitosan since a plethora of materials such as kappa-carrageenan (κ-c), pectin, starch and whey are compatible with this process.26,27 κ-c is a linear sulphated polysaccharides extracted from seaweed (Fig. 1). It exists as a random coil in aqueous solution at elevated temperatures; however, it turns to a gel through gel–sol transition when being cooled to its gelation temperature (Tg), attaining thermoreversibility.28 In addition, when exposed to potassium ions, κ-c polymer chains adopt a double helix configuration, leading to gelation.29 κ-c hydrogels are known to exhibit syneresis, a process to spontaneously release fluid under certain gel composition conditions without the application of external forces, resulting in the expulsion of a liquid. This property has attracted much attention and interest in the usage of κ-c in the drug delivery systems.28,30
 |
| Fig. 1 Chemical structures of alginate, chitosan and κ-carrageenan polymers. | |
Single core microcapsules have been synthesized with UV-cured tripropylene glycol diacrylate (TPGDA) shells using microfluidic double emulsion technology.31 Liu and coworkers developed monodisperse core–shell chitosan microcapsules, which maintained its spherical shape and structural integrity at neutral pH but underwent decomposition at acidic pH to release the encapsulated hydrophobic drugs.32 Alginate microparticles and microcapsules were generated by chemical gelation using a robust hybrid glass-polydimethylsiloxane (PDMS) microfluidic device in presence of an electric field.33 Hia and coworkers fabricated alginate microcapsules containing epoxy resin using an electrospraying method to produce a capsule-based self-healing composite system.34 They were able to demonstrate multiple healing events up to 3 cycles due to their structure with healing efficiency as high as 86% under an in situ healing test. Similarly, Barbaz-Isfahani and coworkers recently reported the formation of a sodium alginate single multicore microcapsule containing encapsulated epoxy and mercaptan hardener using a two-step electrospraying technique for as self-healing applications.35
Herein, we developed a multicore microcapsule system consisting of thermo-responsive κ-c polymer beads entrapped in larger chitosan shells. This was achieved by first extruding κ-c beads, after which the hardened beads were mixed in a chitosan solution and extruded in a gelling bath of sodium triphosphate. The morphology of the resulting microcapsules was visualized using optical and electron microscopies. The thermo-responsiveness of the system was demonstrated by entrapping primary-colored dyes in the inner κ-c matrix, which upon heating or solar simulation allowed the co-mixing of the dyes to produce a secondary color while still being entrapped within the outer polymer membrane. This type of system can be relevant to agricultural applications, where the controlled release of chemicals could be triggered by sun and/or temperature change, and extend the residual period as well as decrease the extent of pollution by leaching. The pesticide ammonium glufosinate was encapsulated in the κ-c and chitosan multicore microcapsules and it was found that there was a controlled release of the pesticide from the multicore microcapsules compared to the beads only. This process of making multicore microcapsules can be extended to other polymer pairs based on applications.
2. Materials and methods
2.1. Materials
Low molecular weight (LMW) chitosan, sodium alginate, glacial acetic acid, κ-carrageenan (κ-c), Tween 80 (polysorbate 80 or polyoxyethylene sorbitan monooleate), sodium triphosphate pentabasic, potassium chloride, calcium chloride and ammonium glufosinate were purchased from Sigma Aldrich. Food grade food coloring was procured from a grocery store.
2.2. General procedure to fabricate κ-c polymer beads
A 1% (w/v) solution of κ-c polymer was prepared in deionized water and used to produce beads via hot prilling (see ESI†). Using Buchi 390 encapsulator (for schematic of instrument, see Fig. S1 in ESI†), κ-c solution was extruded using a 200 μm diameter nozzle at a temperature of 60 °C into a bath containing solution of 2% KCl and 1% Tween 80 at ambient temperature. At high temperature, it is possible to keep the polymer solution in the liquid form and facilitate pumping, preventing gelling and clogging the system. The air pressure was maintained between 800 and 900 mbar and the frequency was set at 2000 Hz. An electric potential between the hardening bath and the nozzle was set at 2500 V. The beads were left to harden in the bath for 30 min under mild stirring. The beads were then collected by mild vacuum filtration to ensure that the beads do not collapse.
2.3. General procedure to fabricate calcium-alginate beads
A 1% (w/v) sodium alginate was prepared and used for the bead formation using a Buchi 390 encapsulator using a single nozzle procedure (see ESI†). The sodium alginate solution was extruded at ambient temperature using an 80 μm diameter nozzle, at a rate of 1 mL min−1 into a 0.1 M solution of CaCl2 under mild stirring. An electric potential between the hardening bath and the nozzle was set at 1000 V with the frequency set at 2000 Hz. The beads were left to harden for 30 min. The beads were then collected by mild vacuum filtration to ensure that the beads do not collapse.
2.4. General procedure for automated production of multicore capsules
For an automated process, the multicore capsules were produced using a Buchi 390 encapsulator. A suspension of κ-c beads in chitosan solution was prepared by mixing 5 g of κ-c beads and 5 g of 2% chitosan in 1% acetic acid. The suspension was agitated as necessary using a vortex until beads were evenly distributed. They were then transferred to a pressure bottle which was attached to the air output and bead producing unit of the encapsulator for extrusion using a 1000 μm diameter nozzle. The multicore beads were formed in a mildly stirred gelling bath of 1% sodium triphosphate solution. The beads were then collected by mild vacuum filtration to ensure that the beads do not collapse.
2.5. Optical microscopy
An aliquot of the beads was first transferred to a microscope glass slide, using a spatula or a wide mouth pipette. The amount of liquid on the slide was adjusted before covering the sample with a cover slip. The slide was placed under a Keyence computer with an xyz optical microscope in the upright position. A magnification between 123× and 456× was used. The average diameter of the microcapsules was calculated using Image J software.
2.6. Solar simulation
To predict whether irradiation with light could trigger a similar effect as a temperature increase, irradiation experiments on the samples were conducted using artificial sunlight generated from a SolSim Solar Simulating Photoreactor (Luzchem Research, Inc., Ottawa, Canada). Luzchem's simulator is designed to yield the internationally accepted standard for sunlight (ASTM Air Mass 1.5 Global Tilt Standard). The total irradiance of the solar simulator output spectrum calibrated to the best approximation of AM1.5 standard in the 280–800 nm region was 590
000 mW m−2. Experiments were conducted at 25 °C on capsules that were placed in a Petri dish and irradiated for 1 h.
2.7. Measurement of color coordinates
The color of the samples was measured using a NixPro Color Sensor. The latter has a light-emitting diode (LED) light source located within the concave base of the sensor and was be controlled wirelessly by a phone. The sensor scan resulted in various color system codes and RGB coordinates were measured. The desired sample consisting of beads or capsules were laid tightly in a single layer on a white background surface and the RGB coordinates were measured by placing the Nix Pro Color Sensor on the sample to prevent any outside light from entering the scan area.
2.8. Encapsulation and release of ammonium glufosinate
Ammonium glufosinate was first encapsulated in κ-c by mixing ammonium glufosinate (21.8 mg) in 1% (w/v) κ-c solution in deionized water (80 mL). The resulting solution was extruded using a Buchi 390 encapsulator equipped with a 200 μm diameter nozzle at a temperature of 60 °C into a bath containing solution of 2% KCl and 1% Tween 80 at ambient temperature. The air pressure was maintained between 800 and 900 mbar and the frequency was set at 2000 Hz. An electric potential between the hardening bath and the nozzle was set at 2500 V. The fabricated beads were kept to be hardened in the bath for 30 min under mild stirring, and then collected by mild vacuum filtration to ensure that the beads did not collapse. An aliquot of the κ-c beads (10 g) containing ammonium glufosinate was mixed with a solution of 1% chitosan in 1% acetic acid (10 mL). The suspension was agitated as necessary in a vortex until beads were evenly distributed. They were then transferred to a pressure bottle which was attached to the air output and bead producing unit of the encapsulator for extrusion. The multicore beads were formed in a mildly stirred gelling bath of 1% sodium triphosphate solution. The beads were then collected by mild vacuum filtration to ensure that the beads do not collapse.
For the release experiments, 5 g of the single beads containing ammonium glufosinate or multicore capsules containing ammonium glufosinate were placed in dialysis tubing (MWCO 3500 Da) and dialyzed against deionized water (1 L). Aliquots (1 mL) of the resulting water containing leached ammonium glufosinate were collected over time and used for HPLC analysis. LS: single κ-c beads in a water bath at ambient temperature (22 °C); HS: single κ-c beads in a water bath, heated for 5 min at 40 °C and then allowed to cool to ambient temperature (22 °C); LE: multicore capsules of inner κ-c beads in chitosan in a water bath at ambient temperature (22 °C); HE: multicore capsules of inner κ-c beads in chitosan, heated for 5 min at 40 °C and then allowed to cool to ambient temperature (22 °C).
2.9. HPLC
HPLC was carried out using a system consisting of a Waters HPLC system equipped with a model 2996 photodiode array detector, a model 600 pump, a model 717 plus injector, and a temperature control module. 2-Amino-4-[hydroxy(methylphosphonoyl)]butanoic acid (commonly called ammonium glufosinate) was analyzed on a polar C18 column (150 × 4.6 mm, 3 μm) (Luna®, Omega) at 40 °C. An injection volume of 100 μL was used. The mobile phase (prepared a mixed buffer of 8 g L−1 KH2PO4 solution, pH adjusted at 3.0 with phosphoric acid, and acetonitrile in the proportion 90
:
10 v/v) was run isocratically at 0.5 mL min−1. The detection was made at 195 nm. Stock solutions of ammonium glufosinate as well as standards and samples were in water. Standard solutions of the ammonium glufosinate at concentrations of 1000 mg L−1, 500 mg L−1 and 100 mg L−1 were prepared and used for HPLC. The calibration range was from 100 to 1000 mg L−1. The retention time of glufosinate under these conditions was of 4.2 min.
3. Results and discussion
3.1 Preparation of multicore microcapsules
Multicore microcapsules can be formed using a variety of polymer combinations. Sodium alginate and chitosan were first used to demonstrate the formation of multicore microcapsules and the former was used to produce beads that were hardened in calcium chloride solution. Sodium alginate forms a relatively robust gel in presence of calcium ions and does not need a surfactant like Tween 80 to prevent beads from undesired agglomeration. It can form homogenous, circular shaped beads and its incorporation into a multicore capsule is seamless and easily observable. Gelation and cross-linking were achieved by the exchange of sodium ions with calcium ions. The polymer network is made of carboxylate groups physically crosslinked with calcium ions, which makes it resilient to external factors such as a higher temperature. A chelating agent like sodium citrate or an excess of sodium ions can be added to break the network and favor the dissolution of the gel. The resulting beads were then mixed in a chitosan polymer solution and extruded into a sodium triphosphate bath, producing the multicore microcapsules (Fig. 2). The positively charged chitosan is able to cross-link with the non-toxic polyanion sodium triphosphate via electrostatic interactions to form a network, leading to gelation. While sodium triphosphate solidifies a chitosan solution, just like sodium citrate, it also liquefies calcium alginate.36 As such, this two-step double encapsulation process resulted in liquid pouches from the liquefied beads, surrounded by a solidified matrix. The cores and matrix were made from oppositely charged polymers, where the cores remain liquid-like coacervates. This technique could be useful to obtain a robust capsule with a water-based, liquid center, capable of housing and multiplying bacteria.
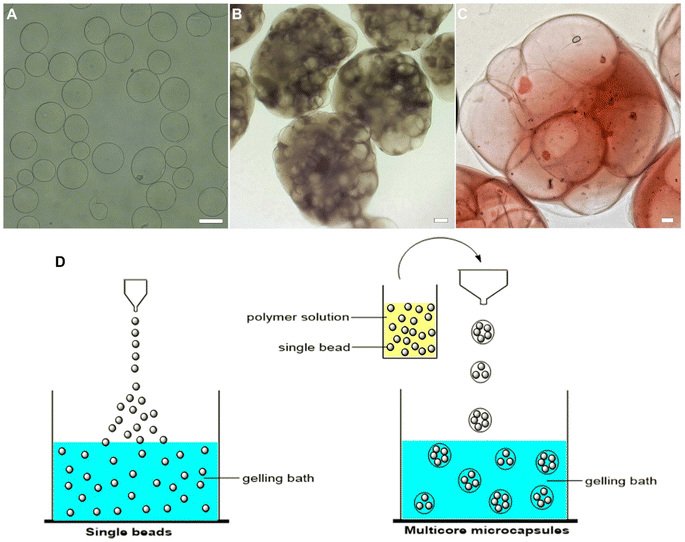 |
| Fig. 2 Optical microscopy images of single beads and multicore beads obtained by extrusion: (A) calcium alginate beads; (B) multicore capsules containing beads of calcium alginate beads in a chitosan outer envelope; (C) multicore capsules containing beads of calcium alginate beads, stained with a red dye prior to encapsulation in a chitosan outer envelope; and (D) schematic showing the encapsulation process to produce single beads and multicore microcapsules. Scale bar = 100 μm. | |
Next, thermo-responsive multicore microcapsules were designed using the thermo-responsive κ-c polymer for the inner beads. κ-c is a linear sulphated thermo-reversible polysaccharide, extracted from seaweed. At elevated temperature, the polymer has a random coil conformation in aqueous solution and forms a gel when the solution is cooled to the gelation temperature.28,29 It has been shown that the peak temperature of gel–sol transition of 1 wt% κ-c hydrogels dispersed in double distilled water is 45 °C. During the formation of the inner κ-c beads, the polymer solution was heated to 60 °C to ensure a smooth extrusion process into a potassium chloride bath. Unlike sodium alginate, κ-c did not seem to form particularly rigid gels at the concentrations of potassium ions used and Tween 80 was required to prevent agglomeration. The κ-c beads were slit across and as expected, at room temperature, the beads had a solid core, while heating the beads at higher temperatures between 50–60 °C, a liquid oozed out due to a phase change.
Scanning electron microscopy (SEM) was used to image the morphology of the beads and multicore capsules formed (Fig. 3). The beads were either spin-dried or vacuum dried prior to imaging. For the κ-c beads, while spin-drying only shrunk the beads (A and B), vacuum drying led to a flattening of the beads (C and D). For the multicore capsules, drying led to a collapse of the structure, exposing the porous nature due to the presence of several beads within the outer envelope (E and F).
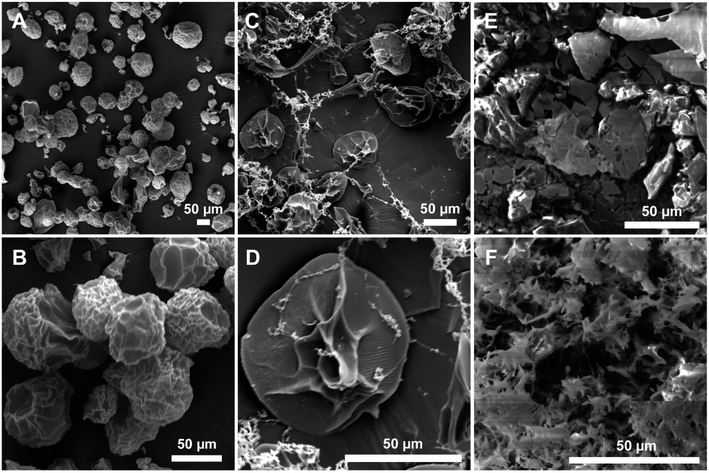 |
| Fig. 3 SEM images of: (A and B) κ-c beads after spin-drying; (C and D) κ-c beads after vacuum drying; and (E and F) multicore capsules of κ-c beads in chitosan after vacuum drying. | |
3.2. Encapsulation and controlled release
The multicore microcapsules contain beads that do not appear to be interconnected. They can potentially be used to encapsulate two or more ingredients in various chambers. The use of a stimuli-responsive material for the inner beads can allow these ingredients to be mixed or reacted when exposed to a stimulus, prior to release. The combination of primary colors was used to demonstrate this process (Fig. 4). Food grade red and blue dyes were first mixed with κ-c solution and extruded into potassium chloride bath to produce red and blue κ-c beads (see Fig. S2 in ESI†). Red and blue are two primary colors, which produces a purple secondary color when mixed. The beads were made from a thermally reversible polymer exhibiting upper critical solution temperature (UCST) that liquefied and diffused its contents at higher temperature.37 At this point, these structures break down and the color from the gel can diffuse, which gives an irreversible product with a mix of both colors. The red and blue κ-c beads were then mixed with chitosan polymer solution and extruded into a sodium triphosphate bath to yield multicore microcapsules containing both red and blue κ-c beads. Each multicore microcapsule enclosed several red and blue κ-c beads within the chitosan envelope. The thermal responsiveness of the multicore capsules was determined by heating them at 50 °C and it was found that while the microcapsules were intact, the inner beads had ruptured, leading to a mixing of the primary colors to produce a secondary purple color (Fig. 4A and B). A solar light exposure of the multicolored capsules in a solar simulator at room temperature resulted in a similar color change. This result shows that this technology can be applied where exposure to sunlight can be used to trigger a reaction or mixing of active ingredients prior to the release of newly formed chemical or formulation into a desired environment (Fig. 4C and D). The color coordinates were recorded using Nixpro color sensor.
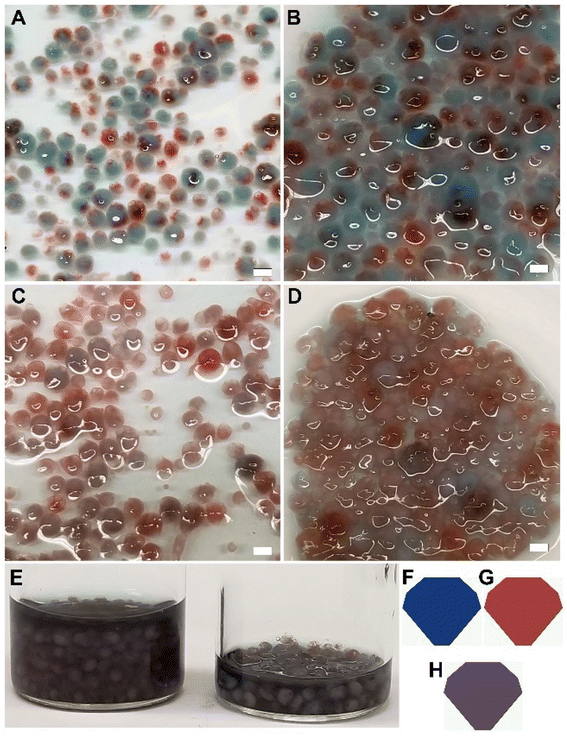 |
| Fig. 4 Multicore capsules containing red and blue κ-c beads (A) before and (C) after heating; (B) before and (D) after solar simulation for 1 h (E) visual comparison after heating (left) and after solar simulation (right). (F, G and H) Are the color patches for the red κ-c beads before heating, blue κ-c beads before heating, and the multicore containing red and blue κ-c beads after heating, respectively. The average RGB coordinates were obtained using a NixPro color sensor and are as follows: R = 21, G = 55, B = 125 (F, blue); R = 162, G = 61, B = 59 (G, red); and R = 90, G = 74, B = 89 (H, purple). Scale bar = 1000 μm. | |
The encapsulation using the multicore capsules system can help address environmental impact of agricultural chemicals. A low residual half-life of a pesticide often necessitates multiple applications to combat the pests at various stages of their life cycles. As such, there is a need to design sustainable solutions such as a delivery approach to provide crops with optimal nutrients and protection against harmful pests and pathogens, along with improving the crop yields. Wijerathna and coworkers tested the efficacy of insecticidal seed treatment using thiamethoxam and foliar insecticide using lambda-cyhalothrin for pea leaf weevil control using a multi-year field plot study.38 Pea leaf weevil is an invasive pest in North America for field pea and faba bean, resulting in yield losses and nitrogen content decrease. These legumes are the preferred hosts of reproductively active weevils, with adults feeding on the plant foliage and larvae feeding on Rhizobium leguminosarum bacteria associated with host plant root nodules along with root nodule tissue. Thiamethoxam has a half-life of 4 days in tomato fruits and a half-life of 9 days in soil under tomato crop, with the total residues reaching below detectable level at 20 days in soil.39 On the other hand, lambda-cyhalothrin showed a half-life of 3.12 days, with the total residues reaching below detectable level at 14 days in soil.40 Wijerathna and coworkers found that thiamethoxam protected faba bean yield by reducing adult and larval damage, while both lambda-cyhalothrin and a nitrogen amendment were ineffective in protecting yield. They attributed the longer residual life of the thiamethoxam as the reason behind the efficiency against the complex life cycle of pea leaf weevil.38 We believe that our multicore microcapsule system can be used to increase the residual life of pesticides in the soil and as such they were used to demonstrate a controlled release of pesticides. Ammonium glufosinate is a broad-spectrum herbicide that is widely used throughout of the world.41,42 Along with its efficacy in controlling important weeds, it has also shown to be effective against certain plant diseases and pests.43 To test the multicore capsules for their controlled release, ammonium glufosinate was encapsulated in thermo-responsive κ-c and further enveloped in chitosan. Its efficacy was compared to single κ-c beads containing ammonium glufosinate by allowing the single (LS) and multicore (LE) materials to leach the herbicide in a water bath at ambient temperature, as well as an initial heating at 40 °C for 5 min, followed by leaching at ambient temperature (HS and HE, respectively). The HPLC data obtained from analysis of water containing the leached herbicide showed a controlled release of the ammonium glufosinate from the multicore microcapsules (LE and HE) compared to single beads (LS and HS) only. While heating the single beads (HS compared to LS), a significant increase in the release of the pesticide was observed, which was triggered by the thermo-responsive nature of the κ-c material, the impact on the multicore microcapsules was minimal. The pesticide was able to find its way out by leaching through the gel surface. The delayed release profile for the multicore capsules is due to the presence of two gel layers, which slows down the process. It is also possible to see how the thermo-responsiveness of the inner material can influence the release profile. Such a system can ensure the release of pesticides like ammonium glufosinate into a plant environment, without over-polluting the environment (Fig. 5).
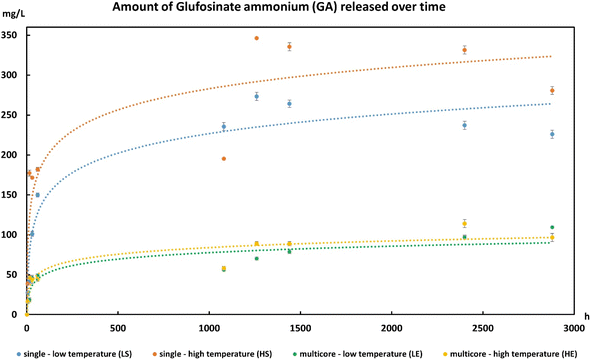 |
| Fig. 5 HPLC data obtained from analysis of water containing the leached herbicide ammonium glufosinate over time from single beads and multicore microcapsules (LE and HE) compared to single beads (LS and HS) only. LS corresponds to single κ-c beads containing ammonium glufosinate that were allowed to leach at ambient temperature; HS corresponds to single κ-c beads containing ammonium glufosinate that were allowed to leach at ambient temperature after an initial heating at 40 °C for 5 min; LE is for multicore microcapsules with inner κ-c beads containing ammonium glufosinate and an outer chitosan envelope that was allowed to leach at ambient temperature; and HE is for multicore microcapsules with inner κ-c beads containing ammonium glufosinate and an outer chitosan envelope that was allowed to leach at ambient temperature after an initial heating at 40 °C for 5 min. | |
4. Conclusion
A novel double encapsulation technique was showcased with multicore capsules, where production parameters can be customized based on the applications. An example of thermally dependent diffusion was shown using the thermal proprieties of κ-c beads as the cores surrounded by chitosan, as a proof of concept. The functioning of these multicore capsules was demonstrated using primary colors, which when exposed to stimuli (heat and solar simulation) resulted into a secondary color. We showed that encapsulation of the commonly used herbicide ammonium glufosinate in the multicore capsules displayed a controlled release of the herbicide and the release of the herbicide from the multicore capsules were lower than the chemical encapsulated in the single bead. We anticipate that this work can be relevant to agriculture, where the controlled-release of the pesticides or fertilizers could be triggered by the sun and/or temperature changes, thus extending the residual period of the chemicals to boost crop productivity as well as minimizing the extent of pollution by leaching of abundant chemicals.
Conflicts of interest
The authors declare no competing financial interest.
Acknowledgements
The authors would like to thank the National Research Council (NRC) New Beginnings Initiative Ideation fund for the financial support.
References
- M. Matsusaki and M. Akashi, Expert Opin. Drug Delivery, 2009, 6, 1207–1217 CrossRef CAS PubMed.
- Z. Ahmad and G. K. Khuller, Expert Opin. Drug Delivery, 2008, 5, 1323–1334 CrossRef CAS PubMed.
- C. Wandrey, A. Bartkowiak, S. E. Harding, Encapsulation Technologies for Active Food Ingredients and Food Processing, in Materials for Encapsulation, ed. N. Zuidam, V. Nedovic, Springer, New York, NY, 2010 Search PubMed.
- B. F. Gibbs, S. Kermasha, I. Alli and C. N. Mulligan, Int. J. Food Sci. Nutr., 1999, 50, 213–224 CrossRef CAS PubMed.
- S. Koudelka and J. Turanek, J. Controlled Release, 2012, 163, 322–334 CrossRef CAS PubMed.
- M. Nuruzzaman, M. M. Rahman, Y. Liu and R. Naidu, J. Agric. Food Chem., 2016, 64, 1447–1483 CrossRef CAS PubMed.
- N. Sozer and J. L. Kokini, Trends Biotechnol., 2009, 27, 82–89 CrossRef CAS PubMed.
- K. A. Whitehead, R. Langer and D. G. Anderson, Nat. Rev. Drug Discovery, 2009, 8, 129–138 CrossRef CAS PubMed.
- P. J. Chawda, J. Shi, S. Xue and S. Young Quek, Food Qual. Saf., 2017, 1, 302–309 CrossRef CAS.
- D. K. Ray, N. D. Mueller, P. C. West and J. A. Foley, PLoS One, 2013, 8, e66428 CrossRef CAS PubMed.
- C. Zhao, B. Liu, S. Piao, X. Wang, D. B. Lobell, Y. Huang, M. Huang, Y. Yao, S. Bassu, P. Ciais, J. L. Durand, J. Elliott, F. Ewert, I. A. Janssens, T. Li, E. Lin, Q. Liu, P. Martre, C. Muller, S. Peng, J. Penuelas, A. C. Ruane, D. Wallach, T. Wang, D. Wu, Z. Liu, Y. Zhu, Z. Zhu and S. Asseng, Proc. Natl. Acad. Sci. U. S. A., 2017, 114, 9326–9331 CrossRef CAS PubMed.
- M. Geranpour, E. Assadpour and S. M. Jafari, Trends Food Sci. Technol., 2020, 102, 71–90 CrossRef CAS.
- S. Ray, U. Raychaudhuri and R. Chakraborty, Food Biosci., 2016, 13, 76–83 CrossRef CAS.
- M. Whelehan and I. W. Marison, J. Microencapsulation, 2011, 28, 669–688 CrossRef CAS PubMed.
- T. Heidebach, P. Forst and U. Kulozik, Crit. Rev. Food Sci. Nutr., 2012, 52, 291–311 CrossRef CAS PubMed.
- M. Tabib, C. Ginies, N. Rakotomanomana and A. Remmal, Int. J. Food Sci., 2022, 2022, 7847276 Search PubMed.
- Z. Fang and B. Bhandari, Trends Food Sci. Technol., 2010, 21, 510–523 CrossRef CAS.
- F. Weinbreck, M. Minor and C. G. de Kruif, J. Microencapsulation, 2004, 21, 667–679 CrossRef CAS PubMed.
- H. J. Kim, H. C. Lee, J. S. Oh, B. A. Shin, C. S. Oh, R. D. Park, K. S. Yang and C. S. Cho, J. Biomater. Sci., Polym. Ed., 1999, 10, 543–556 CrossRef CAS PubMed.
- P. C.-L. Hui, W.-Y. Wang, C.-W. Kan, F. S.-F. Ng, C.-E. Zhou, E. Wat, V. X. Zhang, C.-L. Chan, C. B.-S. Lau and P.-C. Leung, Colloids Surf., A, 2013, 434, 95–101 CrossRef CAS.
- M. Z. I. Mollah, H. M. Zahid, Z. Mahal, M. R. I. Faruque and M. U. Khandaker, Front. Mol. Biosci., 2021, 8, 719972 CrossRef CAS PubMed.
- K. Y. Lee and D. J. Mooney, Prog. Polym. Sci., 2012, 37, 106–126 CrossRef CAS PubMed.
- M. Rinaudo, Prog. Polym. Sci., 2006, 31, 603–632 CrossRef CAS.
- D. Raafat and H. G. Sahl, Microb. Biotechnol., 2009, 2, 186–201 CrossRef CAS PubMed.
- E. T. Baran, J. F. Mano and R. L. Reis, J. Mater. Sci.: Mater. Med., 2004, 15, 759–765 CrossRef CAS PubMed.
- V. Nedovic, A. Kalusevic, V. Manojlovic, S. Levic and B. Bugarski, Procedia Food Sci., 2011, 1, 1806–1815 CrossRef CAS.
- O. P. Bamidele and M. N. Emmambux, Crit. Rev. Food Sci. Nutr., 2021, 61, 3100–3118 CrossRef PubMed.
- K. Ako, Carbohydr. Polym., 2015, 115, 408–414 CrossRef CAS PubMed.
- R. Willaert and G. Baron, Rev. Chem. Eng., 1996, 12, 1–205 CAS.
- G. W. Scherer, J. Non-Cryst. Solids, 1989, 108, 18–27 CrossRef CAS.
- Y. Hennequin, N. Pannacci, C. P. de Torres, G. Tetradis-Meris, S. Chapuliot, E. Bouchaud and P. Tabeling, Langmuir, 2009, 25, 7857–7861 CrossRef CAS PubMed.
- L. Liu, J.-P. Yang, X.-J. Ju, R. Xie, Y.-M. Liu, W. Wang, J.-J. Zhang, C. H. Niu and L.-Y. Chu, Soft Matter, 2011, 7, 4821–4827 RSC.
- M. D. Eqbal and V. Gundabala, J. Micromech. Microeng., 2020, 30, 045002 CrossRef CAS.
- I. L. Hia, P. Pasbakhsh, E. S. Chan and S. P. Chai, Sci. Rep., 2016, 6, 34674 CrossRef PubMed.
- R. Barbaz-Isfahani, S. Saber-Samandari and M. Salehi, Int. J. Biol. Macromol., 2022, 200, 532–542 CrossRef CAS PubMed.
- M. Chanratana, G. H. Han, M. Melvin Joe, A. Roy Choudhury, S. Sundaram, M. A. Halim and T. Sa, Arch. Agron. Soil Sci., 2018, 64, 1489–1502 CrossRef CAS.
- C. Rochas, M. Rinaudo and S. Landry, Carbohydr. Polym., 1990, 12, 255–266 CrossRef CAS.
- A. Wijerathna, M. Evenden, P. Reid, B. Tidemann and H. Cárcamo, J. Econ. Entomol., 2021, 114, 1597–1606 CrossRef CAS PubMed.
- R. Karmakar and G. Kulshrestha, Pest Manage. Sci., 2009, 65, 931–937 CrossRef CAS PubMed.
- F. Malhat, N. M. Loutfy and M. T. Ahmed, Int. J. Food Contam., 2016, 3, 8 CrossRef.
- G. Hoerlein, Rev. Environ. Contam. Toxicol., 1994, 138, 73–145 CAS.
- A. G. Calas, O. Richard, S. Meme, J. C. Beloeil, B. T. Doan, T. Gefflaut, W. Meme, W. E. Crusio, J. Pichon and C. Montecot, Neurotoxicology, 2008, 29, 740–747 CrossRef CAS PubMed.
- S. O. Duke, Z. Pan, A. G. Chittiboyina, D. R. Swale and T. C. Sparks, Pestic. Biochem. Physiol., 2023, 191, 105340 CrossRef CAS PubMed.
|
This journal is © The Royal Society of Chemistry 2024 |
Click here to see how this site uses Cookies. View our privacy policy here.