DOI:
10.1039/D4RA01709A
(Paper)
RSC Adv., 2024,
14, 20867-20878
Effect of a mesoporous NiCo2O4 urchin-like structure catalyzed with a surface oxidized LiBH4 system for reversible hydrogen storage applications†
Received
5th March 2024
, Accepted 18th June 2024
First published on
2nd July 2024
Abstract
A mesoporous NiCo2O4 urchin-like structure was synthesized by applying a facile hydrothermal method. Different concentrations of NiCo2O4 urchin-like structures were mixed with a surface oxidized LiBH4 system using a wet-impregnation method, followed by heat treatment. The hydrogen storage capacity of LiBH4 + 25% NiCo2O4, LiBH4 + 50% NiCo2O4 and LiBH4 + 75% NiCo2O4 systems was investigated. Typically, hydrogenated LiBH4 + 25% NiCo2O4, LiBH4 + 50% NiCo2O4 and LiBH4 + 75% NiCo2O4 systems desorbed 2.85 wt%, 3.78 wt% and 3.91 wt% of hydrogen, respectively, at the dehydrogenation temperature ranging from room temperature (RT) to 275 °C. Further, the LiBH4 + 75% NiCo2O4 system exhibited better kinetics than other systems and released ∼5.8 wt% of hydrogen at a isothermal dehydrogenation temperature of 250 °C in 60 minutes. Hydrogen binding energies were calculated as 0.28 eV, 0.27 eV and 0.26 eV for LiBH4 + 25% NiCo2O4, LiBH4 + 50% NiCo2O4 and LiBH4 + 75% NiCo2O4 systems, respectively. Moreover, the calculated activation energies of LiBH4 + 25% NiCo2O4, LiBH4 + 50% NiCo2O4 and LiBH4 + 75% NiCo2O4 systems are 17.99 kJ mol−1, 17.03 kJ mol−1 and 16.92 kJ mol−1, respectively. The calculated BET (Brunauer–Emmett–Teller) surface area of NiCo2O4 and LiBH4 + 75% NiCo2O4 systems is 124.05 and 136.62 m2 g−1, respectively. These results showed that hydrogen sorption and desorption properties are significantly increased by the influence of mesoporous structure, lower binding energy and activation energy of LiBH4 + 75% NiCo2O4 system.
1 Introduction
In the current scenario, over 80% of the global energy supply is obtained from conventional non-renewable fossil fuels, including natural gas, coal and petroleum sources.1,2 The use of fossil fuels releases greenhouse gases and other polluting substances that seriously harm the environment.3 Hydrogen has been recognized as a abundant, clean, economical and efficient energy source for a wide range of applications, including stationary supplies, distribution and various transportable hydrogen-fuelled systems. Hydrogen is an excellent long-term option to overcome the energy-based environmental issues, due to its advantages of high energy density (120 MJ kg−1). During the energy conversion process, it just produces water as an output product.4–7 However, the primary challenge among them is the absence of suitable techniques for storing the hydrogen. Moreover, storing hydrogen using traditional methods, such as compression and liquefaction, requires tremendous pressure and/or very low temperatures, which in turn increases the chances of leakage and safety issues.8,9
Solid-state hydrogen storage is a feasible technique to achieve future hydrogen storage goals considering its safety conditions, compactness and efficiency compared to conventional hydrogen storage systems.10,11 Hydrogen can be bound either physically or chemically with different storage materials, which has a definite advantages in the aspects of safety and efficiency.12,13 In recent decades, LiBH4 has been widely explored14,15 as a reversible hydrogen storage material for on-board energy carrier applications owing to its high volumetric and gravimetric hydrogen densities. Its slow kinetics, higher dehydrogenation temperature and severe reversible conditions (600 °C/35 MPa H2) are major concerns. This behaviour can be associated with its high thermal stability and poor catalytic activity.16 The complex hydrides LiBH4,17–21 NaBH4,22,23 and LiH24 are extremely sensitive to air/moisture in the ambient atmosphere. These materials react instantly with water through exothermic reactions. Therefore, the certain operating conditions such as dry air and inert atmosphere are required to prevent the dehydrogenation of raw materials. Consequently, it is essential to understand how raw materials behave under moisture conditions. To manage the reactivity and unfavourable impacts at each stage of the industrial process, including storage, manufacturing, handling and processing, many possible reaction strategies are suggested for handling LiBH4 under ambient atmospheric conditions according to the report by Goudon et al.17 However, for on-board applications, the reversibility of LiBH4 is still challenging.25,26
Recently, transition metal oxides have been used to improve the reversible hydrogen storage performance of complex hydrides through nanoconfinement and nanocatalytic effects. Some experimental studies involving LiBH4 with transition metal oxides have been reported for hydrogen storage applications.27–29 Zhang and his co-workers30 reported that the re-hydrogenated (at 400 °C under 4.5 MPa) LiBH4/M-Fe2O3/TiF3 system absorbed ∼4.27 wt% of hydrogen within 60 minutes. In a previous study, ∼1.7 wt% hydrogen uptake capacity for LiBH4–2LiNH2-0.05/3Co3O4 systems was found under rehydrogenation conditions of 25–220 °C and 110 bar H2 pressure.31 Additionally, the dehydrogenated LiBH4/SiO2/TiF3 system reabsorbed ∼1.2 wt% of hydrogen at 300 °C within 233 minutes under 4.5 MPa.32 Au et al.33 investigated the reversibility of LiBH4/TiO2 and LiBH4/V2O3 systems at 600 °C under 100 bar H2 pressure with the hydrogen uptake capacities of 7.8 wt% and 7.9 wt%, respectively. Zang and his colleagues34 reported the destabilization of the LiBH4 system by NiCo2O4 nanorods. The mesoporous NiCo2O4 nanorods were mixed with LiBH4 in different mass ratios (2
:
1, 1
:
1, 1
:
2) using the ball milling technique in a controlled atmosphere. As shown in their report, they directly dehydrogenated the raw LiBH4 system using the NiCo2O4 structure and did not hydrogenate and rehydrogenate the LiBH4–NiCo2O4 composites. Additionally, the in situ Co (Ni) and Co–B (Ni–B) are formed as intermediates during dehydrogenation. In this study, we used the surface oxidized LiBH4 system and mixed it with mesoporous NiCo2O4 urchin-like structure in different weight percentages (LiBH4 + 25% NiCo2O4, LiBH4 + 50% NiCo2O4 and LiBH4 + 75% NiCo2O4) by applying a simple wet-impregnation technique under lab atmospheric conditions. Moreover, we studied hydrogenation and dehydrogenation experiments systematically. From the hydrogenation and dehydrogenation results, it was found that no new phases were introduced into the LiBH4/NiCo2O4 systems. Cabo et al. and Zhang et al. found that Ni and Co are good candidates for hydrogen storage due to their variable valence and higher Pauling's electronegativity than Mn, Fe, Ti and other metals. It is reasonable to infer that NiCo2O4, Co3O4 and NiO have a notable influence on improving the hydrogen sorption/desorption properties of the MgH2 and LiBH4 systems. The aforementioned reports mostly present the destabilization effect of the LiBH4 system with sufficient hydrogen release at high temperatures.28,31,35 However, they have not been performed effectively in the reversible hydrogen storage process. Furthermore, nanostructured metal oxides present numerous advantages due to the hierarchical arrangement of pore structures with sizes ranging from nanometres to micrometres and catalytic activity. Moreover, the favourable hydrogen release mechanism depends on the mesoporous materials with large surface areas owing to more active sites to assist the dissociation/recombination of H2 molecules.36,37 Butt et al. investigated the hydrogen storage properties of hierarchical ZnV2O4 (ref. 38) and Zn2V2O7 (ref. 39) nanostructures, which absorbed 1.76 and 1.21 wt% of H2 under 5 MPa at 200 °C, respectively.
Under these scenarios, the present study focuses on investigating the hydrogen sorption/desorption performance of a mesoporous urchin-like NiCo2O4 structure catalyzed using a surface oxidized (air-sensitized) LiBH4 system. From our previously reported study of the hydrogen storage properties of a surface oxidized LiBH4 system catalyzed with NiO nanoflower,19 we adopted nanorods and nanoplates.40 Herein, the NiCo2O4 structure was prepared using the hydrothermal method, and LiBH4/NiCo2O4 systems were prepared using the ultrasonic-assisted wet-impregnation method. From scanning electron microscopy (SEM) and transmission electron microscopy (TEM) assessments, the mesoporous NiCo2O4 structure was found to have an urchin-like formation. According to Kang et al.,41 the surface oxidized LiBH4 could reduce the dehydrogenation and activation energy barrier during hydrogen desorption. In addition, the surface oxidation effects help to reach thermodynamically predicted temperatures for dehydrogenation reactions.40,42,43 In this study, it is observed that mesoporous NiCo2O4 with a surface oxidized LiBH4 system presents an outstanding storage performance. Notably, 5.8 wt% of hydrogen was desorbed using a LiBH4 + 75% NiCo2O4 system in isothermal dehydrogenation at 250 °C for 60 minutes. Moreover, the weak chemical binding nature and activation energy of the LiBH4/NiCo2O4 systems are discussed based on thermogravimetric analysis. Additionally, possible reaction mechanisms are discussed.
2 Experimental section
2.1 Materials
The commercial chemical LiBH4 was purchased from Sigma-Aldrich with ≥95.0% purity. Additional chemicals Ni(NO3)2·6H2O, Co(NO3)2·6H2O, CO(NH2)2, diethyl-ether (DEE) and ethanol were purchased from Siscon Research Laboratory, India, and used without further purification.
2.2 Methods
2.2.1 Synthesis of nickel cobalt oxide.
In a typical hydrothermal process, 1 mol Ni(NO3)2·6H2O and 2 mol Co(NO3)2·6H2O were dissolved in 60 mL distilled water, and 12 mol urea was added into the solution under continuous stirring for 60 minutes. Then, the solution was transferred into a 100 mL Teflon-lined stainless-steel autoclave and heated at 120 °C for 8 hours. After cooling to room temperature, the precipitate was centrifuged and washed several times with water and ethanol. Then, the precipitate was dried at 60 °C for 12 hours. Finally, the NiCo2O4 was obtained after annealing at 400 °C for 3 hours in air. Fig. 1 shows a schematic representation image of the urchin-like NiCo2O4 structure preparation.
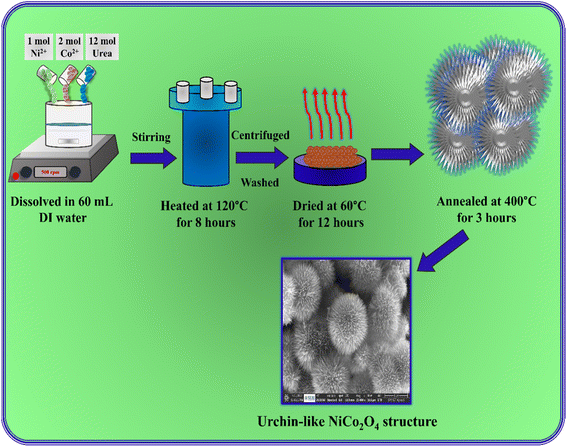 |
| Fig. 1 Schematic representation of the preparation of an urchin-like NiCo2O4 structure. | |
2.2.2 Synthesis of LiBH4/NiCo2O4 systems.
LiBH4/NiCo2O4 systems were prepared using the wet-chemical impregnation method under ambient atmospheric conditions. Surface oxidized LiBH4 + 25% NiCo2O4 was dispersed in 30 mL of diethyl ether (DEE). The mixed solution was sonicated in a bath sonicator for 2 hours. Then, the resultant mixture was dried at 100 °C using a hotplate with vigorous stirring for solvent evaporation. The LiBH4 + 50% NiCo2O4 and LiBH4 + 75% NiCo2O4 were also prepared by applying the aforementioned procedure. Later, LiBH4 + 25% NiCo2O4, LiBH4 + 50% NiCo2O4 and LiBH4 + 75% NiCo2O4 mixtures were annealed at 275 °C for 1 hour under argon atmosphere.
2.3 Hydrogen sorption and desorption experiments
Before hydrogenation, the thermal stability of LiBH4/NiCo2O4 systems was confirmed by applying the TG-STA under an argon atmosphere. Then, 10–20 mg of the sample was placed in the hydrogenation chamber of the custom-built hydrogenation setup. Next, the sample was hydrogenated at 150 °C for 30 minutes under 4, 6 and 10 bar H2 pressure conditions. Immediately, the hydrogenated sample was transferred to the TG-STA for dehydrogenation from RT to 275 °C with a 15 °C min−1 rate under an argon gas flow of 100 mL min−1. Additionally, isothermal dehydrogenation experiments were performed at 250 °C for 1 hour using argon medium with 100 mL min−1 flow for the hydrogenated samples (at 150 °C for 30 minutes under 10 bar H2 pressure).
3 Results and discussion
3.1 Structural and functional group analyses
The structural analysis of NiCo2O4 and LiBH4/NiCo2O4 samples was performed using the powder X-ray diffraction (PXRD) technique. As shown in Fig. 2a, the diffracted peaks of NiCo2O4 indexed at 18.7°, 31.2°, 36.6°, 38.3°, 44.5°, 55.3°, 59.3° and 65.2°, corresponding to the (1 1 1), (2 2 0), (3 1 1), (2 2 2), (4 0 0), (4 2 2), (5 1 1) and (4 4 0) planes, respectively, are matched with standard data of JCPDS card no. #20-0781. The calculated average crystallite size is ∼26 nm. Further, the lattice constant values are found to be a = b = c = 8.11 Å for the NiCo2O4 structure. Fig. 2b–d shows that the XRD spectra of LiBH4 + 25% NiCo2O4, LiBH4 + 50% NiCo2O4 and LiBH4 + 75% NiCo2O4 systems indicate the presence of LiBH4 (JCPDS card no. #27-0287), LiB[OH]4 (JCPDS card no. 01-075-1156) and NiCo2O4 (JCPDS card no. #20-0781) phases. According to the results, it is clear that increasing the concentration of NiCo2O4 in the system produced an increased peak intensity. Additionally, the combined phases of borohydroxide (B[OH]4−) and borohydride (BH4−) were identified in the systems due to the surface oxidation of LiBH4.
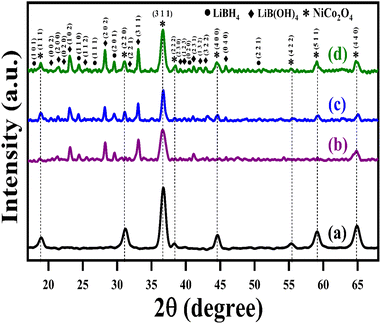 |
| Fig. 2 XRD patterns of (a) NiCo2O4, (b) LiBH4 + 25% NiCo2O4, (c) LiBH4 + 50% NiCo2O4, and (d) LiBH4 + 75% NiCo2O4 systems. | |
Fig. 3(a–c) illustrates the Fourier Transform Infrared Spectroscopy (FTIR) patterns of LiBH4 + 75% NiCo2O4, LiBH4 + 50% NiCo2O4, and LiBH4 + 25% NiCo2O4 systems. The characteristic bands of Ni–O and Co–O are observed at 551 and 645 cm−1,44 respectively, originating from the stretching vibration modes of the spinel NiCo2O4 structure. In addition, the vibration modes, such as O–H stretching (3592–3180 cm−1),19 B–H stretching (2484–2196 cm−1),45 B–O stretching (1559–1375 cm−1),19 B–H bending (1367–1007 cm−1)45 and B–H rocking (867–681 cm−1),19 can be observed in the spectra, confirming the presence of NiCo2O4, LiBH4 and LiB[OH]4. The air exposure of LiBH4 caused the existence of B[OH]4.
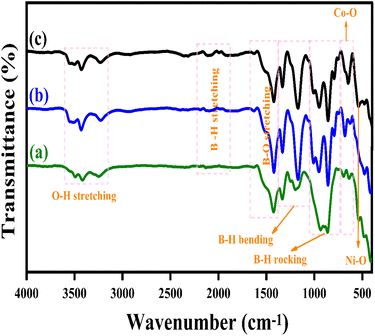 |
| Fig. 3 FTIR patterns of (a) LiBH4 + 75% NiCo2O4, (b) LiBH4 + 50% NiCo2O4,and (c) LiBH4 + 25% NiCo2O4 systems. | |
3.2 Hydrogen desorption analysis
Before hydrogenation, the thermal stability of the systems was investigated using a thermal analyzer. As shown in Fig. 4, negligible 0.05, 0.17, 0.15 and 0.10 wt% of weight losses were observed in the systems, from RT to 275 °C. After confirming the thermal stability, all hydrogen sorption measurements were carried out at 150 °C for 30 minutes under 4 bar H2 pressure. The hydrogenated systems were dehydrogenated using a thermal analyzer from RT to 275 °C under an argon medium at a heating rate of 15 °C min−1. During non-isothermal hydrogen desorption, the hydrogenated NiCo2O4 system released 0.12 wt% of hydrogen, while the LiBH4 + 25% NiCo2O4, LiBH4 + 50% NiCo2O4 and LiBH4 + 75% NiCo2O4 systems gradually released 0.74, 1.15 and 1.71 wt% hydrogen, respectively, from RT to 275 °C in argon atmosphere (Fig. 5a). The hydrogen storage behaviour of the systems was analysed by increasing the hydrogenation conditions of 6 and 10 bar H2 pressures separately under a constant hydrogenation temperature of 150 °C for 30 minutes. As shown in Fig. 5b, the urchin-like NiCo2O4 structure liberated 0.14 and 0.37 wt% hydrogen from their adsorbed surface sites under the hydrogenation conditions of 6 and 10 bar, respectively. Meanwhile, LiBH4 + 25% NiCo2O4, LiBH4 + 50% NiCo2O4 and LiBH4 + 75% NiCo2O4 systems desorbed 1.13, 1.67 and 2.48 wt% of H2, respectively, for 6 bar pressure of hydrogenation. Moreover, under 10 bar hydrogenation condition, LiBH4 + 25% NiCo2O4, LiBH4 + 50% NiCo2O4 and LiBH4 + 75% NiCo2O4 systems released 2.85, 3.78 and 3.91 wt% of H2, respectively, from RT to 275 °C, as shown in Fig. 5c. From the above results, it can be inferred that the increased hydrogen sorption and desorption capacity of LiBH4/NiCo2O4 systems are attributed to the increased hydrogenation pressure.
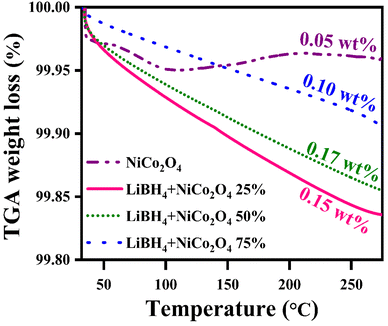 |
| Fig. 4 Thermal stability curves of NiCo2O4, LiBH4 + 25% NiCo2O4, LiBH4 + 50% NiCo2O4 and LiBH4 + 75% NiCo2O4 systems before hydrogenation. | |
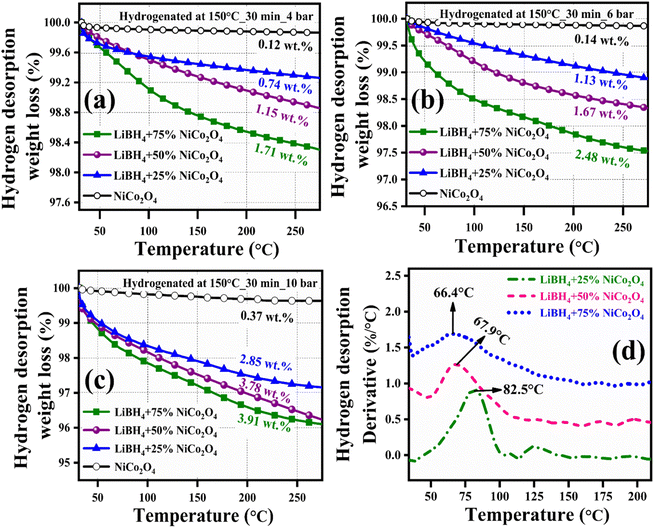 |
| Fig. 5 Non-isothermal hydrogen desorption curves of NiCo2O4, LiBH4 + 25% NiCo2O4, LiBH4 + 50% NiCo2O4 and LiBH4 + 75% NiCo2O4 systems: (a) Hydrogenated at 150 °C for 30 min under 4 bar, (b) hydrogenated at 150 °C for 30 min under 6 bar, and (c) hydrogenated at 150 °C for 30 min under 10 bar; (d) H2 desorption derivative TG curves of hydrogenated (150 °C for 30 min under 10 bar) LiBH4 + 25% NiCo2O4, LiBH4 + 50% NiCo2O4 and LiBH4 + 75% NiCo2O4 systems. | |
To determine the binding and kinetics nature of the hydrogenated (150 °C for 30 minutes under 10 bar) LiBH4/NiCo2O4 systems, the activation and binding energies were calculated using Kissinger's46–48 and van't Hoff47,48 relations by analyzing the hydrogen desorption derivative thermogravimetric (DTG) curves. As shown in Fig. 5d, DTG curves exhibit the hydrogen desorption behaviour of LiBH4 + 25% NiCo2O4, LiBH4 + 50% NiCo2O4 and LiBH4 + 75% NiCo2O4 systems at the H2 desorption peak positions of 82.5, 67.9 and 66.4 °C, respectively. The corresponding activation energy (Ed) and binding energy (Eb) of the systems are, respectively, determined using the following equations:
| 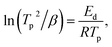 | (1) |
| 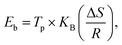 | (2) |
where
Tp is the absolute temperature corresponding to the hydrogen desorption peak position,
β is the heating rate,
Ed is the activation energy,
Eb is the ‘binding energy of 1H
2 molecule,
KB is the Boltzmann's constant, Δ
S is the change in entropy (75.44 J mol
−1 K
−1) and
R is the ‘gas constant’. The calculated activation and binding energies of the systems are presented in
Table 1.
Table 1 Hydrogen desorption characteristics of the systems under non-isothermal H2 desorption conditions
Systems |
Desorption peak temperature |
Desorption capacity up to 275 °C |
Binding energy (Eb) |
Activation energy (Ed) |
LiBH4 + 25% NiCo2O4 |
82.5 °C |
2.85 wt% |
0.28 eV |
17.99 kJ mol−1 |
LiBH4 + 50% NiCo2O4 |
67.9 °C |
3.78 wt% |
0.27 eV |
17.03 kJ mol−1 |
LiBH4 + 75% NiCo2O4 |
66.4 °C |
3.91 wt% |
0.26 eV |
16.92 kJ mol−1 |
For an ideal hydrogen storage material, the binding energy of hydrogen should fall in the range of ∼0.2–0.4 eV.49–52 The recommended binding energy range for physisorption is ∼0.01–0.1 eV and that for chemisorption is ∼2–3 eV. As shown in Table 1, the activation and binding energies of the systems lie in the ranges 16.92–17.99 kJ mol−1 and 0.26–0.28 eV, respectively. As suggested by Silambarasan et al. and Ioannatos et al., this desorption activation energy is close to the adsorption energy in the case of strong physisorption and/or weak chemisorption, which is in the range of 10–40 kJ mol−1.46,47 According to previous studies, it is clear that the H2 is weakly chemisorbed on the LiBH4/NiCo2O4 networks.
Hydrogenation and dehydrogenation cycle studies were investigated for the selected LiBH4 + 75% NiCo2O4 system up to 5 times. Typically, hydrogenation experiments were carried out at 150 °C for 30 minutes under 10 bar pressure conditions for all hydrogenation cycles. Meanwhile, dehydrogenation cycle tests were conducted from RT to 275 °C under an argon atmosphere at a 15 °C min−1 heating rate. As shown in Fig. S1 (in ESI),† it was found to be ±0.25 wt% of variations in the H2 release by the system (3.76 wt%). Additionally, H2 sorption and desorption cycle tests confirm the stability of the systems, and further studies are required to evaluate their long-term performance.
Hydrogen desorption kinetics were studied under isothermal hydrogen desorption conditions at 250 °C for 60 minutes. Initially, the systems were hydrogenated at 150 °C for 30 minutes under 10 bar H2 pressure. As shown in Fig. 6, the LiBH4 + 75% NiCo2O4 system presented a higher desorption kinetics rate, and the capacity is 5.8 wt% in 60 minutes at 250 °C, while the LiBH4 + 25% NiCo2O4 and LiBH4 + 50% NiCo2O4 systems desorbed 3.7 and 4.5 wt% of hydrogen, respectively. Table 2 presents a comparison of the hydrogen desorption capacities of the LiBH4/NiCo2O4 systems in this study with those in other studies.
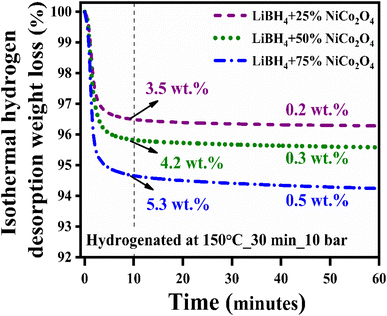 |
| Fig. 6 Isothermal hydrogen desorption curves of LiBH4 + 25% NiCo2O4, LiBH4 + 50% NiCo2O4 and LiBH4 + 75% NiCo2O4 systems. | |
Table 2 Hydrogen storage properties of LiBH4 catalyzed with spinel metal oxide structures
System |
Synthesis method |
Isothermal hydrogen desorption capacity |
Ref. |
Hydrogen desorption condition after hydrogenation.
|
LiBH4 + 2LiNH2 + 0.05 wt% Co3O4 |
Ball milling at the argon-filled glove box |
8.2 wt% (200 °C for 60 min) |
53
|
LiBH4 + NiCo2O4 |
3.8 wt% (250 °C for 50 min) |
34
|
LiBH4 + 9% mol NiFe2O4 |
2.4 wt% (300 °C for 20 min) |
54
|
LiBH4 + 20 wt% M-Fe2O3 + 30 wt% TiF3 |
8.6 wt% (400 °C for 20 min) |
55
|
LiBH4 + 2ZnO/ZnCo2O4 |
Wet chemical impregnation at argon |
3.1 wt% (300 °C for 60 min) |
56
|
LiBH4 + NiMnO3 |
2.8 wt% (300 °C for 60 min) |
57
|
LiBH4 + 75% NiCo2O4 a |
Wet chemical impregnation in the air |
5.8 wt% (250 °C for 60 min) |
This work |
LiBH4 + 50% NiCo2O4 a |
4.5 wt% (250 °C for 60 min) |
LiBH4 + 25% NiCo2O4 a |
3.7 wt% (250 °C for 60 min) |
As shown in Table 2, the other systems also hold significant desorption kinetics through the destabilization effect. However, they did not report the rehydrogenation and then hydrogen desorption. Among other systems, the surface oxidized LiBH4/NiCo2O4 systems exhibit better hydrogen sorption kinetics.
3.3 Morphological and structural composition analyses
SEM and TEM analyses were employed to investigate the morphological and structural nature of the NiCo2O4 and LiBH4 + 75% NiCo2O4 systems. As depicted in Fig. 7a, the SEM micrographs showed the urchin-like structure of NiCo2O4 with an average diameter of ∼4 µm, which consisted of several ultrafine nanoneedles. This nanoneedles-assembled urchin-like structure is attributed to the surfactant used in the reaction, causing more active sites at the nucleation stage.58 The SEM micrographs of the LiBH4 + 75% NiCo2O4 system are shown in Fig. 7b, exhibiting a molten form of LiBH4 with an urchin-like structure. TEM micrographs provide more insight into the morphology and structure of the LiBH4 + 75% NiCo2O4 system, as shown in Fig. 7(c) and (d). The average grain size is calculated as ∼19 nm, as shown in Fig. S2 (in ESI).† The interplanar d-spacing determined from HRTEM images (Fig. 7d) of LiBH4 + 75% NiCo2O4 system was 0.22, 0.27 and 0.47 nm,59 which were assigned to the lattice planes of (1 1 0), (1 1 2) and (1 1 1) for the LiBH4, LiB[OH]4 and NiCo2O4 phases, respectively. Fig. S3 (in ESI)† shows the selected area electron diffraction (SAED) pattern of the LiBH4 + 75% NiCo2O4 system, which showed that the polycrystalline nature and diffracted ring patterns agreed well with the XRD results.
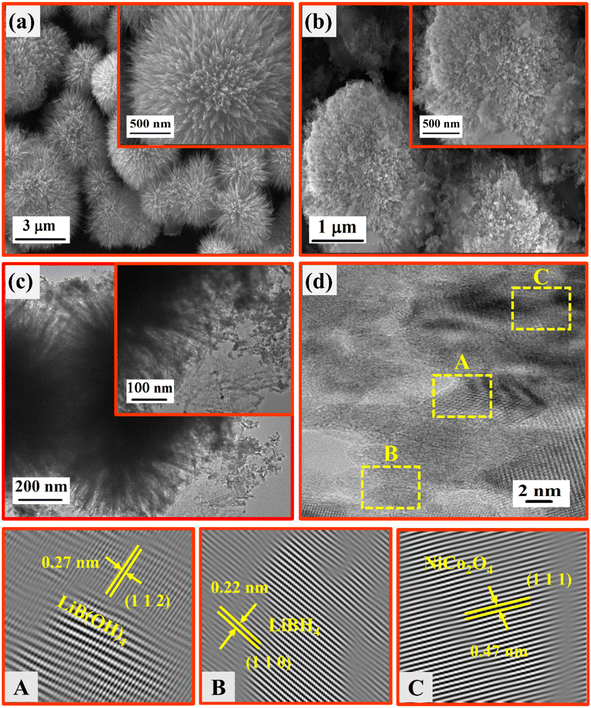 |
| Fig. 7 SEM images of (a) NiCo2O4 and (b) LiBH4 + 75% NiCo2O4; (c) TEM images of LiBH4 + 75% NiCo2O4; (d) HRTEM images of LiBH4 + 75% NiCo2O4. | |
3.4 Surface area analysis
For a better understanding of the H2 storage mechanism, BET analysis was carried out to measure the specific surface area and porous properties of NiCo2O4 and LiBH4 + 75% NiCo2O4 systems. As shown in Fig. 8a, the specific surface areas of NiCo2O4 and LiBH4 + 75% NiCo2O4 systems were determined by N2 adsorption/desorption isotherms. Both systems exhibit BET type IV isotherms. The BET surface areas for the NiCo2O4 and LiBH4 + 75% NiCo2O4 systems were 124.05 and 136.62 m2 g−1, respectively. As can be observed from the Barrett–Joyner–Halenda (BJH) pore size distribution curves, the NiCo2O4 (Fig. 8b) and LiBH4 + 75% NiCo2O4 (Fig. 8c) systems possess average pore volumes of 0.129 and 0.376 cm3 g−1; they exhibit average pore diameters of 4.16 and 11.01 nm, respectively. After the addition of NiCo2O4, the surface area and porous nature of the systems are slightly increased, contributing favourable H2 sorption and desorption to the LiBH4/NiCo2O4 systems.
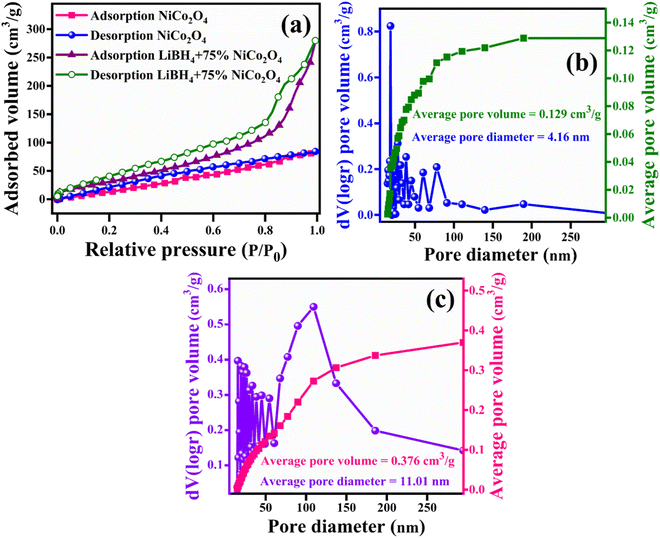 |
| Fig. 8 (a) Nitrogen adsorption/desorption curves of NiCo2O4 and LiBH4 + 75% NiCo2O4; BJH pore size distribution curves of (b) NiCo2O4 and (c) LiBH4 + 75% NiCo2O4. | |
3.5 Elemental and compositional analysis
The binding energies and surface composition of the LiBH4 + 75% NiCo2O4 system were analyzed by applying the X-ray photoelectron spectroscopy (XPS) technique. The survey spectrum and high-resolution spectra of Li 1s, B 1s, O 1s, Ni 2p3 and Co 2p3 are presented in Fig. 9. The survey spectrum illustrates the presence of Li, B, O, Co and Ni elements (Fig. 9a). The binding energy of Li 1s is observed at 55.3 eV (ref. 18 and 60) (Fig. 9b), while B 1s is located at 192.0 eV (ref. 18 and 61) (Fig. 9c). Additionally, the peaks of O 1s are observed at the binding energies of 529.3 and 532.0 eV,59 which correspond to the typical metal–oxygen (M–O) and borohydroxide (B–OH) bonds, respectively (Fig. 9d). As shown in Fig. 9e, the high-resolution Co 2p3 spectrum is distributed into two Co species, that is the fitting peaks at 781.0 and 796.9 eV, which are attributed to the Co2+, and the peaks located at 779.4 and 795.0 eV are assigned to the Co3+ components. Additionally, satellite peaks are observed at 784.9 and 802.8 eV.62 Meanwhile, the Ni 2p3 spectrum is identified with Ni2+ and Ni3+ components having two satellite peaks. The deconvoluted peaks at 854.1 and 872.1 eV correspond to the Ni2+, while the peaks at 856.1 and 874.1 eV are due to the Ni3+. Moreover, the two satellite peaks are observed at binding energies of 861.5 and 879.6 eV (ref. 62) for Ni 2p3, as shown in Fig. 9f.
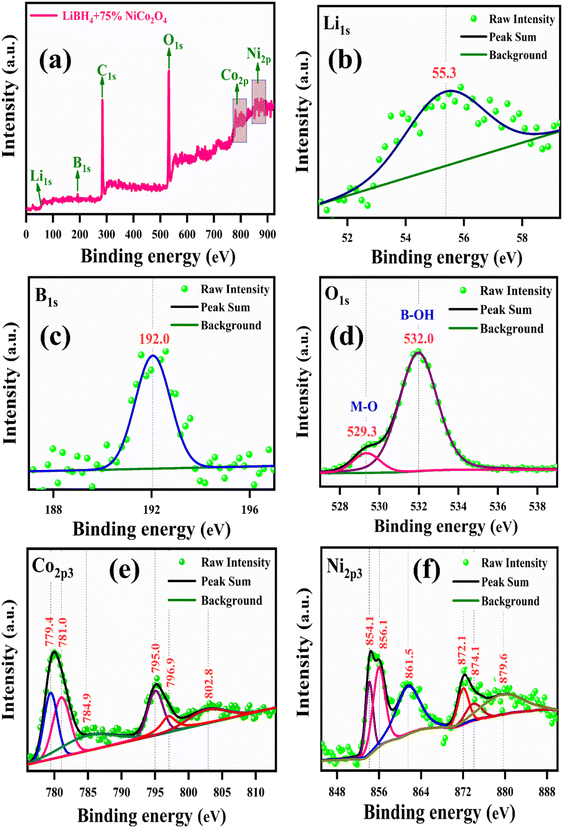 |
| Fig. 9 XPS spectrum of LiBH4 + 75% NiCo2O4: (a) survey spectrum, (b) Li 1s, (c) B 1s, (d) O 1s, (e) Co 2p3, and (f) Ni 2p3. | |
3.6 Structural analysis after hydrogenation, dehydrogenation and rehydrogenation
The microstructural changes in the LiBH4 + 75% NiCo2O4 system were characterized by XRD analysis after hydrogenation and dehydrogenation processes. Fig. 10(a) and (b) show the XRD pattern of the LiBH4 + 75% NiCo2O4 system after hydrogenation and dehydrogenation. Slight changes in peak intensities suggest the lattice rearrangement and defect refinement induced by the presence of hydrogen molecules.57,63 No structural changes were observed in the LiBH4 + 75% NiCo2O4 system after hydrogenation and dehydrogenation.64 The average crystallite size (D), micro-strain (ε) and dislocation density (δ)65–69 are presented in Table 3. From the structural analysis, it can be inferred that the hydrogen charging and discharging conditions did not alter the phase structures of the systems. Further theoretical and experimental investigations on these systems would lead to a better understanding of catalytic and synergistic mechanisms.
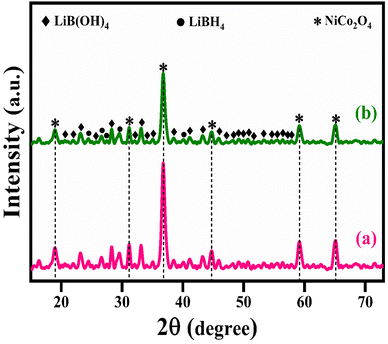 |
| Fig. 10 XRD patterns of LiBH4 + 75% NiCo2O4 (a) after hydrogenation (150 °C for 30 min under 10 bar) and (b) after dehydrogenation at 275 °C. | |
Table 3 Microstructural characteristics of the LiBH4 + 75% NiCo2O4 system
Process |
Average crystallite size (nm) |
Average dislocation density (×10−3 nm−2) |
Average micro strain (×10−3) |
Hydrogenated at 150 °C for 30 minutes under 10 bar |
58 |
2.95 |
5.11 |
Dehydrogenated at 275 °C |
55 |
3.15 |
5.49 |
Fig. 11 illustrates the schematic diagram of hydrogen sorption and desorption mechanisms for LiBH4/NiCo2O4 systems. During hydrogenation, hydrogen molecules diffused through the surface pores, reaching the interfaces of LiBH4/NiCo2O4 systems. It is expected that hydrogen can be absorbed in molecular and dissociated forms. In the following dehydrogenation process, the absorbed H2 molecules/hydrogen ions from the surfaces and interfaces of LiBH4/NiCo2O4 systems were desorbed at a dehydrogenation temperature of 275 °C. This suggests that the increased concentration of NiCo2O4 in the system impacts the active sites of LiBH4/NiCo2O4 systems and causes improved storage capacity.
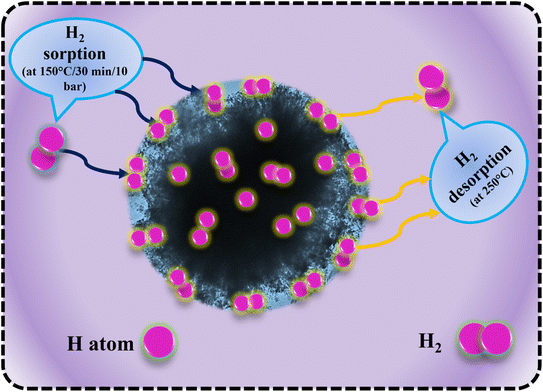 |
| Fig. 11 Hydrogen sorption and desorption mechanisms of LiBH4/NiCo2O4 systems. | |
4 Conclusion
The mesoporous NiCo2O4 was synthesized using the hydrothermal method, and a simple ultrasonication-assisted wet-impregnation method was used for the preparation of LiBH4/NiCo2O4 hydrogen storage systems. The physico-chemical properties were studied using XRD, FTIR, XPS, SEM, TEM, BET and TGA analyses. Furthermore, hydrogenation experiments were performed for the LiBH4 + NiCo2O4 systems at a hydrogenation temperature of 150 °C for 30 min under different pressures. From the non-isothermal dehydrogenation results, LiBH4 + 25% NiCo2O4, LiBH4 + 50% NiCo2O4 and LiBH4 + 75% NiCo2O4 systems exhibited the hydrogen release of 2.85, 3.78 and 3.91 wt%, respectively. Isothermal dehydrogenation of the hydrogenated LiBH4 + 75% NiCo2O4 system at 250 °C released ∼5.8 wt% of hydrogen in 60 minutes. The addition of NiCo2O4 significantly enhanced the dehydrogenation kinetics of the surface oxidized LiBH4 system. Moreover, the estimated hydrogen binding energy of the LiBH4/NiCo2O4 systems lies in the range of 0.26–0.28 eV. Therefore, it can be inferred that the interaction between H2 molecules and LiBH4/NiCo2O4 systems is weak chemisorption or strong physisorption. The remarkable hydrogen storage performance was shown by the LiBH4 + 75% NiCo2O4 system possibly due to the larger surface area, porosity and favourable electronic environment of NiCo2O4. Moreover, these results provide new opportunities to consider LiBH4/NiCo2O4 systems as an economical and prospective material for hydrogen charging/discharging applications.
Data availability
The data supporting this article are included in ESI.†
Author contributions
Ajaijawahar Kaliyaperumal: conceptualization, methodology, data curation, formal analysis, writing – original draft, writing – review & editing. Gokuladeepan Periyasamy: formal analysis, validation, writing – review & editing. Iyakutti Kombiah: formal analysis, validation, investigation, writing – review & editing. Karthigeyan Annamalai: conceptualization, resources, investigation, supervision, writing – original draft, writing – review & editing.
Conflicts of interest
There are no conflicts of interest to declare.
Acknowledgements
The authors acknowledge the SCIF, SRMIST for providing the instrumentation facilities. The authors would like to acknowledge the PNCF and NRC, SRM IST for providing the characterization facilities.
References
- J. Wang and A. Waseem, Geosci. Front., 2024, 15, 101757 CrossRef CAS
.
- X. Liu, S. Bo, P. Lynn, H. Ali, L. Hongyou, Y. Cong and F. Guanyun, Wiley Interdiscip. Rev.: Energy Environ., 2019, 8, e342 CrossRef PubMed
.
-
K. O. D. Yoro and O. Michael, in Advances in Carbon Capture, Elsevier, 2020, pp. 3–28, DOI:10.1016/B978-0-12-819657-1.00001-3
.
- B. C. N. Tashie-Lewis and S. G. Nnabuife, Chem. Eng. J. Adv., 2021, 8, 100172 CrossRef CAS
.
- M. G. Rasul, M. A. Hazrat, M. A. Sattar, M. I. Jahirul and M. J. Shearer, Energy Convers. Manage., 2022, 272, 116326 CrossRef CAS
.
- O. Faye, S. Jerzy and E. Ubong, Int. J. Hydrogen Energy, 2022, 47, 13771–13802 CrossRef CAS
.
- R. Rameshbabu, P. Johnny Koh Siaw, K. Ajaijawahar, V. Victor, J. Sapana, P. Nalandhiran and K. Tiong Sieh, J. Alloys Compd., 2024, 997, 174830 CrossRef CAS
.
- S. R. Bosu, Int. J. Hydrogen Energy, 2024, 52, 352–370 CrossRef CAS
.
- K. Nivedhitha, T. Beena, N. R. Banapurmath, M. A. Umarfarooq, R. Venkatesh, M. E. M. Soudagar and A. Ümit, Int. J. Hydrogen Energy, 2024, 61, 1259–1273 CrossRef CAS
.
- C. Tarhan and Ç. Mehmet Ali, J. Energy Storage, 2021, 40, 102676 CrossRef
.
- H.-J. Lin, L. Hai-Wen, S. Huaiyu, L. Yanshan and A. Kohta, Mater. Today Energy, 2020, 17, 100463 CrossRef
.
- M. S. Salman, L. Qiwen, L. Xiaoxuan, P. Chulaluck, R. Nigel, C. Mehdi, W. Ting, S. Prabal, L. Wei and G. Aiden, Journal of Alloys and Compounds, 2022, 920, 165936 CrossRef CAS
.
- J. Liu, M. Yong, Y. Jinggang, S. Lei, G. Dongliang and X. Peng, Front. Chem., 2022, 982, DOI:10.3389/fchem.2022.945208
.
- X. Zhang, Z. Wenxuan, Z. Lingchao, H. Zhenguo, H. Jianjiang, G. Mingxia, P. Hongge and L. Yongfeng, Chem. Eng. J., 2022, 428, 132566 CrossRef CAS
.
- Y. Huang, Z. Yun, L. Jianding, B. Xiaozhi, G. Junpo, S. Jingjun, G. Yan, Z. Qi, L. Jing and L. Wen, J. Mater. Sci. Technol., 2023, 153, 181–204 CrossRef CAS
.
- C. Li, P. Peng, D. W. Zhou and L. Wan, Int. J. Hydrogen Energy, 2011, 36, 14512–14526 CrossRef CAS
.
- J. Goudon, F. Bernard, J. Renouard and P. Yvart, Int. J. Hydrogen Energy, 2010, 35, 11071–11076 CrossRef CAS
.
- L. Vellingiri, A. Karthigeyan, K. Ramamurthi and K. Iyakutti, RSC Adv., 2019, 9, 31483–31496 RSC
.
- A. Kaliyaperumal, V. Lathapriya, P. Gokuladeepan and A. Karthigeyan, J. Mater. Sci.: Mater. Electron., 2022, 33, 9144–9154 CrossRef CAS
.
- Y. Fan, C. Dandan, Y. Zhenluo, C. Qiang, F. Guangxin, Z. Dan and L. Baozhong, Front. Chem., 2020, 8, 45 CrossRef CAS PubMed
.
- T. C. Lee, S. C. John, W. Lin and D. Eric, ACS Appl. Energy Mater., 2021, 4, 9742–9750 CrossRef CAS
.
- A. M. Beaird, A. D. Thomas and M. A. Matthews, Ind. Eng. Chem. Res., 2010, 49, 9596–9599 CrossRef CAS
.
- M. Murtomaa, E. Laine, J. Salonen and O. Kuusinen, Powder Handling Process., 1999, 11, 87–90 CAS
.
- C. Haertling, R. J. Hanrahan Jr and R. Smith, J. Nucl. Mater., 2006, 349, 195–233 CrossRef CAS
.
- P. Mauron, B. Florian, F. Oliver, R. Arndt, B. Michael, C. N. Zwicky and Z. Andreas, J. Phys. Chem. B, 2008, 112, 906–910 CrossRef CAS PubMed
.
- J. J. Vajo, L. S. Sky and M. Florian, J. Phys. Chem. B, 2005, 109, 3719–3722 CrossRef CAS PubMed
.
- H. Yuan, Z. Xugang, L. Zhinian, Y. Jianhua, G. Xiumei, W. Shumao, L. Xiaopeng and J. Lijun, Int. J. Hydrogen Energy, 2012, 37, 3292–3297 CrossRef CAS
.
- M. Cabo, G. Sebastiano, P. Eva, M. Chiara, G. Alessandro, M. Amedeo, R. Emma, S. Santiago and B. Maria Dolors, Int. J. Hydrogen Energy, 2011, 36, 5400–5410 CrossRef CAS
.
- X. Yu, D. M. Grant and G. S. Walker, J. Phys. Chem. C, 2009, 113, 17945–17949 CrossRef CAS
.
- H. Zhang, C. Zhong, S. Li-Xian, S. Yu-Jia, X. Fen, L. Hui, Z. Jian, H. Zi-Qiang, J. Xia and L. Zhi-Bao, J. Therm. Anal. Calorim., 2013, 112, 1407–1414 CrossRef CAS
.
- Y. Zhang, L. Yongfeng, P. Yuepeng, G. Mingxia and P. Hongge, J. Mater. Chem. A, 2014, 2, 11155–11161 RSC
.
- Y. Zhang, Z. Wan-Sheng, F. Mei-Qiang, L. Shu-Sheng, C. Hai-Liang, Z. Yan-Hua, G. Xiu-Ying and S. Li-Xian, J. Phys. Chem. C, 2008, 112, 4005–4010 CrossRef CAS
.
- M. Au, S. William, J. Arthur and Z. Christine, J. Alloys Compd., 2008, 462, 303–309 CrossRef CAS
.
- L. Zang, Z. Qiuyu, L. Li, H. Yike, C. Xiaoya, J. Lifang, Y. Huatang and W. Yijing, Chem.–Asian J., 2018, 13, 99–105 CrossRef CAS PubMed
.
- Y. Zhang, L. Yongfeng, L. Tao, G. Mingxia and P. Hongge, Int. J. Hydrogen Energy, 2013, 38, 13318–13327 CrossRef CAS
.
- J. Shao, X. Xuezhang, C. Lixin, F. Xiulin, L. Shouquan, G. Hongwei and W. Qidong, J. Mater. Chem. A, 2012, 22, 20764–20772 RSC
.
- G. Xia, Y. H. Guo, Z. Wu and X. B. Yu, J. Alloys Compd., 2009, 479, 545–548 CrossRef CAS
.
- F. K. Butt, T. Muhammad, C. Chuanbao, I. Faryal, R. Ahmed, W. S. Khan, A. Zulfiqar, M. Nasir, M. Tanveer and M. Asif, ACS Appl. Mater. Interfaces, 2014, 6, 13635–13641 CrossRef CAS PubMed
.
- F. K. Butt, C. Chuanbao, I. Faryal, T. Muhammad, H. Rafaqat, R. Ahmed and W. S. Khan, Int. J. Hydrogen Energy, 2015, 40, 9359–9364 CrossRef CAS
.
- A. Kaliyaperumal, P. Gokuladeepan and A. Karthigeyan, Int. J. Hydrogen Energy, 2023, 50, 812–826 CrossRef
.
- J. K. Kang, K. Se Yun, H. Young Soo, R. P. Muller and W. A. Goddard III, Appl. Phys. Lett., 2005, 87, 111904 CrossRef
.
- S. Kato, B. Michael, B. Andreas, V. Zakaznova-Herzog, R. Arndt, O. Shin-ichi and Z. Andreas, Phys. Chem. Chem. Phys., 2010, 12, 10950–10955 RSC
.
- J. Carrillo-Bucio, T.-G. Juan, L. Rogelio and K. Suárez-Alcántara, Inorganics, 2017, 5, 82 CrossRef
.
- V. Venkatachalam, A. A., A. Alghamdi and R. Jayavel, Ionics, 2017, 23, 977–984 CrossRef CAS
.
- T. Wang and K. F. Aguey‐Zinsou, Energy Technol., 2019, 7, 1801159 CrossRef
.
- G. E. Ioannatos and E. V. Xenophon, Int. J. Hydrogen Energy, 2010, 35, 622–628 CrossRef CAS
.
- D. Silambarasan, V. J. Surya, V. Vasu and K. Iyakutti, Int. J. Hydrogen Energy, 2013, 38, 4011–4016 CrossRef CAS
.
- L. Vellingiri, A. Karthigeyan, K. Ramamurthi and K. Iyakutti, Int. J. Hydrogen Energy, 2018, 43, 10396–10409 CrossRef CAS
.
- E. Klontzas, T. Emmanuel and G. E. Froudakis, J. Phys. Chem. Lett., 2011, 2, 1824–1830 CrossRef CAS
.
- R. C. Lochan and H.-G. Martin, Phys. Chem. Chem. Phys., 2006, 8, 1357–1370 RSC
.
- R. Rameshbabu, K. Siaw Paw, K. Ajaijawahar, J. Sapana, A. John, Y. Chong Tak, T. Sieh Kiong and Y. Talal, Int. J. Hydrogen Energy, 2024, 61, 743–753 CrossRef CAS
.
- G. Periyasamy, K. Ajaijawahar, R. Rameshbabu and A. Karthigeyan, J. Mater. Sci.: Mater. Electron., 2024, 35, 1–11 CrossRef
.
- Y. Zhang, L. Yongfeng, P. Yuepeng, G. Mingxia and P. Hongge, J. Mater. Chem. A, 2014, 2, 11155–11161 RSC
.
- J. Zhang, P. Li, Q. Wan, F. Zhai, A. A. Volinsky and X. Qu, RSC Adv., 2015, 5, 81212–81219 RSC
.
- H. Zhang, Z. Cao, L.-X. Sun, Y.-J. Sun, F. Xu, H. Liu, J. Zhang, Z.-Q. Huang, X. Jiang and Z.-B. Li, J. Therm. Anal. Calorim., 2013, 112, 1407–1414 CrossRef CAS
.
- X. Xu, Z. Lei, Z. Yaran, Z. Yan, W. Yijing and J. Lifang, J. Power Sources, 2017, 359, 134–141 CrossRef CAS
.
- X. Xu, Z. Lei, Z. Yaran, L. Yongchang, W. Yijing and J. Lifang, Int. J. Hydrogen Energy, 2017, 42, 25824–25830 CrossRef CAS
.
- J. Wang, Z. Yangyang, Y. Junhua, W. Hongmei, H. Jinghua, M. Juyi, Z. Shuoqing and H. Shahid, RSC Adv., 2016, 6, 70077–70084 RSC
.
- Y. Zhang, Z. Yihe, Z. Deyang and S. Li, Dalton Trans., 2017, 46, 9457–9465 RSC
.
- M. Shek, J. Hrbek, T. K. Sham and G.-Q. Xu, Surf. Sci., 1990, 234, 324–334 CrossRef CAS
.
- X. Lü, W. Jianjun, L. Tianquan, W. Dongyun, H. Fuqiang, X. Xiaoming and J. Mianheng, J. Mater. Chem., 2011, 21, 10685–10689 RSC
.
- J. Zhang, M. Qianwen, D. Yaping, G. Kai, Y. Xinxin and Z. Jingtai, ACS Appl. Mater. Interfaces, 2017, 9, 29771–29781 CrossRef CAS PubMed
.
- K. Xian, N. Bo, L. Zigen, G. Mingxia, L. Zhenglong, S. Congxiao, L. Yongfeng, G. Zhengxiao and P. Hongge, Chem. Eng. J., 2021, 407, 127156 CrossRef CAS
.
- J. Shao, X. Xuezhang, F. Xiulin, Z. Liuting, L. Shouquan, G. Hongwei, W. Qidong and C. Lixin, J. Phys. Chem. C, 2014, 118, 11252–11260 CrossRef CAS
.
- S. Mustapha, M. M. Ndamitso, A. S. Abdulkareem, J. O. Tijani, D. T. Shuaib, A. K. Mohammed and A. Sumaila, Adv. Nat. Sci.: Nanosci. Nanotechnol., 2019, 10, 045013 Search PubMed
.
- K. Maniammal, G. Madhu and V. Biju, Phys. E, 2017, 85, 214–222 CrossRef CAS
.
- P. Bindu and T. Sabu, J. Theor. Appl. Phys., 2014, 8, 123–134 CrossRef
.
- S. K. Sen, B. Utpal Chandra, M. S. Manir, M. Pritish, D. Supria, P. Mollika, M. A. M. Chowdhury and M. A. Hakim, Adv. Nat. Sci.: Nanosci. Nanotechnol., 2020, 11, 025004 CAS
.
- E. Karvannan, V. V., T. S. Nivin, J. Archana, M. Navaneethan and A. Karthigeyan, Mater. Chem. Phys., 2024, 312, 128506 CrossRef CAS
.
|
This journal is © The Royal Society of Chemistry 2024 |