DOI:
10.1039/D4RA00583J
(Paper)
RSC Adv., 2024,
14, 11872-11876
Benzoic acid inhibits intrinsic ion migration for efficient and stable perovskite solar cells
Received
23rd January 2024
, Accepted 2nd April 2024
First published on 15th April 2024
Abstract
An efficient perovskite solar cell (PSC) has the following characteristics: (1) large perovskite grain size; (2) small ion migration; (3) low defect density states. Here, benzoic acid was employed as an additive to a perovskite solution to improve the thin film quality. Surprisingly, 1.0%-BA can implement all of these features. Therefore, the power conversion efficiency (PCE) of the champion PSC is 18.05%, which is superior to that of the control device (15.42%). In addition, BA-doped PSC kept 86% of its primary PCE after 30 days (RH: 35%), but the control device only retained 75% under the same conditions. The improvement of its stability is because of the inhibition of the cation migration of perovskite by the addition of BA and the passivation of perovskite defects. The results can acquire a better understanding of the potential applications of small organic molecules in improving the PCE and stability of PSC devices.
1 Introduction
Owing to the low cost of materials, high efficiency and easy preparation processability, perovskite solar cells (PSCs) have attracted considerable attention in the field of photovoltaics.1–3 Nowadays, PSC has realized a power conversion efficiency (PCE) of 26.1%, comparable with those of the market silicon solar cells, which is mainly attributed to the low excitation energy, excellent light absorbency and long carrier lifetime of halide perovskite materials.4–9 Although the PSC has made some progress in recent years, the defects of grain boundary, I vacancy (VI) and Pb vacancy (VPB) in hybrid perovskite films are still striking.1,3,10 The above defects and the grain boundaries can be named as trap states, trapping/recombining photo-generated carriers,11–14 which severely affects the PSC efficiency and device stability.15–18
The grain boundary is an important part of the trap state, a channel for ion migration and the specific location of water-etched perovskite. Therefore, it is very important to prepare high quality perovskite films with a larger grain size and smaller grain boundary to reduce trap state density, inhibit ion migration and reduce external water and oxygen erosion. There are many defects in a perovskite besides grain boundary. These not only cause non-radiative recombination of photocarriers, but also adsorb water and oxygen molecules from the air, thus reducing the efficiency and stability of the devices.
Some efforts have been made to passivate or eliminate these defects.19 At present, the two-step solution method is regarded as an effective method for preparing perovskite films, which has a controllable morphology and better reproducibility than the one-step solution method. In addition, additive engineering has been proved to be another way to solve these defects.20–24 The universally used passivators include inorganic metal salts,25,26 organic small molecules,27 and polymers.28 There are some passivators, including carbonyls and the benzene ring, which are rich in electron functional groups, that can bind to uncoordinated Pb2+ ions/halide anions in the perovskite,20,23 thus effectively reducing deep-level defects. In addition, uncoordinated Pb2+ ions are more sensitive to moisture than to halogen anions.29,30 Therefore, it is urgent to use an effective passivation strategy to eliminate the uncoordinated Pb2+ ions. 3,5-Bis(trifluoromethyl)benzoic acid was used as an additive in (FAPbI3)0.85(MAPbBr3)0.15 to suppress trap-assisted nonradiative recombination.31 Bark et al. employed trimesic acid in a PSC to reduce carrier recombination.32 Mishra et al. used BA post-treatment in CsPbBr3 PNCs to improve the PL and long-term stability.33 However, there are few reports on the improvement of PSC performance by an additive two-step method.
For the above research situation, a benzoic acid (BA) additive was introduced into the perovskite precursor solution to creatively prepare high quality films. BA has many functions: (1) to stabilize the composition of the perovskite and inhibit the internal ion migration; (2) to induce the growth of large perovskite grains, reduce the defects and inhibit the erosion of the perovskite by water and oxygen. Ultimately, the efficiency and stability of the PSCs are greatly enhanced.
2 Experimental section
2.1 Materials and reagents
FTO glass, Ti(iPrO)2(acac)2 and spiro-OMeTAD were ordered from LumTec. Lithium bis-(trifluoromethylsulfonyl)imide (>99.95%), CH3NH3I (MAI), lead iodide, TiCl4, and benzoic acid (BA, 99%, Mw = 122.12 g mol−1) were provided from Aldrich.
2.2 Device fabrication
Distilled water and isopropanol were used to clean the FTO glass, sequentially. The FTO was dried with a N2 stream and treated with UV ozone for 20 minutes. The compact TiO2 was spin-coated at 2000 rpm, 1000 rpm s−1 for 35 s using Ti(iPrO)2(acac)2 (0.3 M) in 2-propanol, which was heated at 125 °C for 10 minutes and 450 °C for 40 minutes. After the substrates cooled down, the TiCl4 solution was used to immerse the substrates at 70 °C for 25 minutes. The mesoporous TiO2 (30 nm particle size) layer was prepared with 5000 rpm for 40 s, then annealed at 125 °C for 10 minutes and 450 °C for 40 minutes.
MAPbI3 was deposited in a glovebox by a two-step deposition. First, PbI2 solution (1 M) was made by dissolving in DMF
:
DMSO (7
:
3 volume ratio). Then, 60 μL of the solution was spun at 5000 rpm for 60 s. Subsequently, the MAI (dissolved in IPA) without or with different amounts (0.5, 1.0, and 1.5%, mass ratio of BA vs. MAI) of BA was spun onto the PbI2 film at 4000 rpm for 30 s and annealed at 120 °C for 45 minutes. Also, 40 μL of a spiro-OMeTAD (60 mM) solution was dropped at 2000 rpm for 35 s on MAPbI3, where 30 mM Li-TFSI and 200 mM 4-tert-butyl-pyridine was added in spiro-OMeTAD. Finally, 100 nm of Au was evaporated to complete the device fabrication.
2.3 Measurements and characterization
The UV-Vis measurements were done using a Cary 60 UV-Vis Spectrophotometer. The XRD patterns were obtained to describe the crystalline structure of the perovskite films by an XRD Rigaku DMAX 2200. FTIR spectra were recorded by a Vertex 7 infrared spectrometric analyzer. The cross-section and top-view images were done by FEI Quanta 600 microscopy. The steady-state PL was determined using an FLS980 spectrometer. The J–V was tested under 100 mW cm−2, AM 1.5 (1 Sun conditions), using a Solar Simulator (ABET 11000). The size of the devices was 0.09 cm2. The EQE was measured under wavelengths from 300 nm to 900 nm.
3 Results and discussion
To study the effect of BA additive on the morphology of the perovskite, SEM was conducted. With the increase of BA content from 0 to 1.0%, the grain size of MAPbI3 becomes larger (Fig. 1a–c). While if the BA content is further increased to above 1.5%, a perovskite film with many pinholes can be observed (Fig. 1d). Moreover, the grain size of the perovskite improved with the increase of BA amount. However, the 1.5%-BA film showed poor quality, which was attributed to the agglomeration of excess BA in the MAPbI3 film. This result indicates that the BA additive could affect the growth manner of the MAPbI3 film. In detail, delayed crystallization is an effective way to control the crystallization process to obtain high quality perovskite films. After adding quantitative BA into MAI, the BA, which has a carboxyl group and a benzene, has a strong interaction with Pb2+ by a chelation effect to form an intermediate phase. The intermediate phase turned into the perovskite phase with the removal of solvents during the heating process and prolonged the crystallization time. As the reaction progresses, the BA could crosslink adjacent perovskite grains, which promoted the inter-connectivity of the film and obtained the large grain size film. However, when an excess amount of BA was added, the excess intermediate phase might in turn hinder the formation of perovskite films, resulting in a poor perovskite film quality with many small particles. The cross-sectional SEM image of a PSC based on a bare perovskite film with an advantageous thickness of 400 nm is shown in Fig. 1e, ensuring adequate light absorption due to a reduced recombination.
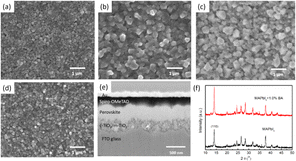 |
| Fig. 1 (a)–(d) Top-view SEM images of MAPbI3, 0.5, 1.0, and 1.5% BA additive films. (e) Cross-sectional SEM image of the MAPbI3 films, (f) XRD patterns of the MAPbI3 films and MAPbI3 with 1.0% BA additive, respectively. | |
The BA additive effect on the perovskite crystallinity was measured using XRD. XRD spectra of the photoactive film without and with 1.0% BA additive are shown in Fig. 1f. The peaks at 2θ of 14.11, 19.91, 23.28, 24.47, 28.15 and 42.86 can be indexed to the (1 1 0), (1 1 2), (2 1 1), (2 0 2), (2 2 0) and (3 1 4) of the perovskite film, respectively. The peaks at 26.45 and 37.69 might belong to the FTO glass. No new peak appears or a clear peak shift after BA passivation, implying BA did not influence the perovskite lattice. The typical diffraction peaks at 14.11° can be indexed to the (1 1 0) plane of BA-perovskite grains which is stronger than that of pristine MAPbI3, indicating that the BA additive can improve the crystallinity of the perovskite. The peak at 12.32° in the MAPbI3 films and MAPbI3 with 1.0% BA additive may be due to the incomplete reaction between MAI and PbI2.
Fig. 2 analyzes the interactions between BA and perovskite using Fourier transform infrared (FTIR) spectra. The C
O vibration band of BA in the photoactive film shifted from 1687 to 1631 cm−1, indicating that the –COOH group in perovskite films formed a chemical bond with Pb ions (Scheme 1).32
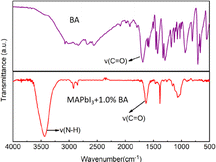 |
| Fig. 2 FTIR spectra of BA and MAPbI3 + 1.0% BA, respectively. | |
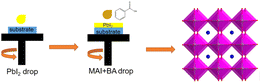 |
| Scheme 1 Diagram of perovskite and BA. | |
The UV-Vis absorption spectra of the MAPbI3 film are presented in Fig. 3A without and with different amounts of BA additive. With the increase of BA amount from 0 to 1.0%, the optical absorption strength of the perovskite films increases gradually, which is due to the increase of crystallinity and perovskite grain size. However, the light absorption of the 1.5% BA-doped perovskite film decreased because of the large amount of small perovskite grains. For Fig. 3B, the intensity of PL increased significantly for the MAPbI3 film with 1.0%-BA in comparison to the bare MAPbI3 film, which may be ascribed to the passivation effect of BA. This could be due to a high-quality crystallinity and fewer defects of the MAPbI3 with BA.
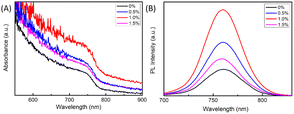 |
| Fig. 3 (A) UV-Vis absorption and (B) steady-PL spectra of PSCs based on Fig. 1(a)–(d) 0, 0.5, 1.0, and 1.5% BA additive films, respectively. | |
To further investigate the effect of BA on the photovoltaic performance of the PSC, J–V curves were tested. The perovskite film containing 1.0% BA additives showed a champion PCE = 18.05%, VOC = 1.09 V, JSC = 23.00 mA cm−2, and FF = 0.72, indicating an optimal BA content of 1.0% (Table 1 and Fig. 4). The improvement in PSC efficiency due to the increased VOC and JSC can be attributed to the improved optical properties and good quality of the photoactive films (Table 1). With the increase of BA content to 1.5%, the PCE decreased to 16.66%, which may be due to the formation of rough perovskite films with multiple pinholes (Fig. 5).
Table 1 The photovoltaic parameters of the champion PSCs based on the J–V curves in Fig. 4
PSC |
JSC (mA cm−2) |
VOC (V) |
FF |
PCE (%) |
a |
21.71 |
1.03 |
0.69 |
15.42 |
b |
22.72 |
1.06 |
0.71 |
17.10 |
c |
23.00 |
1.09 |
0.72 |
18.05 |
d |
22.79 |
1.03 |
0.71 |
16.66 |
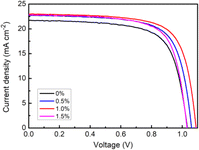 |
| Fig. 4 J–V curves of PSCs based on 0, 0.5, 1.0, and 1.5% BA additive-MAPbI3 films, respectively. | |
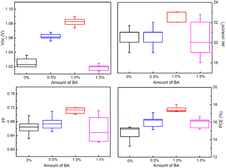 |
| Fig. 5 Statistics for the different parameters of the measured solar cells (20 samples) for 0, 0.5, 1.0, and 1.5% BA additive-MAPbI3 films, respectively. It presents the distribution of device parameters. It can be observed that the Jsc increases obviously for the 1.0%-BA PSC. The enhancement of the Jsc can be attributed to the improved film quality. Moreover, the statistics of the parameters show a narrow distribution for 1.0% BA-MAPbI3, which indicates the great reproducibility of the devices. | |
Fig. 6 shows the EQE spectra of PSCs with BA in contrast to that of the bare cell. The light response from the EQE spectra was significantly increased in the wavelength range of 300–800 nm and the BA-doped PSC was approximately 80%. This could be attributed to the enhanced film quality, as shown in the SEM images. The integrated current densities for the MAPbI3 and MAPbI3 + 1.0% BA devices were calculated to be 19.2 and 22.7 mA cm−2, respectively, matching the Jsc measured from the J–V curve.
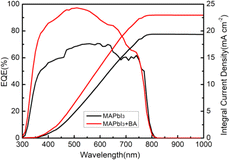 |
| Fig. 6 EQE spectra and integrated current density of MAPbI3 and MAPbI3 + 1.0% BA. | |
Apart from the efficient photovoltaic performance, we also explored the humidity stability. The time evolutions of PCE for PSCs without and with BA are shown in Fig. 7. The unencapsulated devices were stored in ambient air with a RH of 35%. The PSCs with a BA additive maintained over 86% of the original PCE after 30 days, versus pristine PSCs, which only retained 75% under the same conditions. The BA improves the stability of the composition due to the structure of BA, which has a carboxyl group and a benzene ring. The hydrogen bond (hydroxyl group and iodide) suppresses the migration of iodine ions, preventing the perovskite from decomposing. Also, the benzene ring as the hydrophobic alkyl chain could prevent the reaction of the perovskite with water.
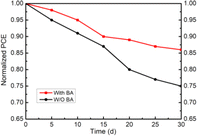 |
| Fig. 7 Normalized PCE values of PSCs versus aging time (room temperature, RH: 35%, time: 30 days). | |
4 Conclusions
To sum up, we introduce a multifunctional BA molecule into an MAPbI3 film to passivate the defect. BA with a –COOH group can bind to uncoordinated Pb2+ and I− ions, reducing the defect density and inhibiting the internal ion migration. The PCE of a BA-doped PSC was 18.05%, which is higher than that of the control device. Moreover, the BA passivated devices showed an improved device stability. After being stored in ambient air for 30 days (temperature: 25 °C and RH: 35%), the unencapsulated devices retained 86% of the initial PCE, versus the PCE of the control device at only about 75%.
Author contributions
All the authors contributed to the study conception and design. Lijun Su: supervision, resources, funding acquisition, project administration, writing review and editing; Xinyu Wu and Xiaoran Chen: methodology, materials preparation, data collection and analysis investigation. All authors commented on previous versions of the manuscript. All authors read and approved the final manuscript.
Conflicts of interest
There are no conflicts to declare.
Acknowledgements
This work was financially supported by the Fund for the Nature Science Foundation of Shanxi Province, China (Grant No. 202203021222414), Shanxi Scholarship Council of China (2022-183), the Higher Education Science and Technology Innovation Program of Shanxi Province, China (Grant No. 2022L584), and the Innovation and Entrepreneurship Program for College Students of Shanxi Province (TYX2023023, TYX2023083).
References
- Y. Wu, H. Zhu, B. B. Yu, S. Akin, Y. Liu, Z. Shen, L. Pan and H. Cai, Chem. Eng. J., 2022, 433, 134613 CrossRef CAS.
- Y. Zhang, L. Xu, Y. Wu, Q. Zhou, Z. Shi, X. Zhuang, B. Liu, B. Dong, X. Bai, W. Xu, D. Zhou and H. Song, Nano Energy, 2021, 90, 106610 CrossRef CAS.
- S. Zhu, J. Wu, W. Sun, W. Pan, F. Cai, J. Liu, L. Chen, X. Chen, C. Wang and X. Wang, ACS Appl. Energy Mater., 2022, 5, 658–666 CrossRef CAS.
- C. Chen, S. Zheng and H. Song, Chem. Soc. Rev., 2021, 50, 7250–7329 RSC.
- National Renewable Energy Laboratory, Best Research-Cell Efficiencies, 2024, http://www.nrel.gov/pv/cell-efficiency.html Search PubMed.
- B. Boro, S. Porwal, D. Kumar, S. Mishra, S. Ghosh, S. Kansal, A. Chandra and T. Singh, Catal. Res., 2022, 2, 1–48 CrossRef.
- M. L. Sun, C. X. Zhao, J. F. Shu and X. Yin, Funct. Nanomater., 2022, 225273 Search PubMed.
- H. Xi, Z. Song, Y. Guo, W. Zhu, L. Ding, W. Zhu, D. Chen and C. Zhang, Polymers, 2022, 14, 2748 CrossRef CAS PubMed.
- R. Kottayi, D. K. Maurya, R. Sittaramane and S. Angaiah, ES Energy Environ., 2022, 18, 1–40 CAS.
- Y. Zhang and N. G. Park, ACS Energy Lett., 2022, 7, 757–765 CrossRef CAS.
- S. Ma, G. Yuan, Y. Zhang, N. Yang, Y. Li and Q. Chen, Energy Environ. Sci., 2022, 15, 13–55 RSC.
- J. E. Kim, S. S. Kim, C. Zuo, M. Gao, D. Vak and D. Y. Kim, Adv. Funct. Mater., 2019, 29, 1809194 CrossRef.
- Y. Zhang, Y. Li, L. Zhang, H. Hu, Z. Tang, B. Xu and N. G. Park, Adv. Energy Mater., 2021, 11, 2102538 CrossRef CAS.
- S. Xiong, Z. Hou, W. Dong, D. Li, J. Yang, R. Bai, Y. Wu, D. Li, H. Wu, Z. Ma, J. Xu, X. Liu and Q. Bao, Adv. Energy Mater., 2021, 11, 2101394 CrossRef CAS.
- D. D. Girolamo, N. Phung, F. U. Kosasih, F. D. Giacomo, F. Matteocci, J. A. Smith, M. A. Flatken, H. Kobler, S. H. T. Cruz, A. Mattoni, L. Cina, B. Rech, A. Latini, G. Divitini, C. Ducati, A. D. Carlo, D. Dini and A. Abate, Adv. Energy Mater., 2020, 10, 2000310 CrossRef.
- L. Duan and A. Uddin, Mater. Chem. Front., 2022, 6, 400–417 RSC.
- R. Xia, X. X. Gao, Y. Zhang, N. Drigo, V. I. E. Queloz, F. F. Tirani, R. Scopelliti, Z. Huang, X. Fang, S. Kinge, Z. Fei, C. Roldan-Carmona, M. K. Nazeeruddin and P. J. Dyson, Adv. Mater., 2020, 32, 2003801 CrossRef CAS PubMed.
- W. Yang, D. Zhong, M. Shi, S. Qu and H. Chen, Science, 2019, 22, 534–543 CAS.
- F. Gao, Y. Zhao, X. Zhang and J. You, Adv. Energy Mater., 2020, 10, 1902650 CrossRef CAS.
- G. Tong, L. K. Ono and Y. Qi, Energy Technol., 2020, 8, 1900961 CrossRef CAS.
- T. Wu, Z. Qin, Y. Wang, Y. Wu, W. Chen, S. Zhang, M. Cai, S. Dai, J. Zhang, J. Liu, Z. Zhou, X. Liu, H. Segawa, H. Tan, Q. Tang, J. Fang, Y. Li, L. Ding, Z. Ning, Y. Qi, Y. Zhang and L. Han, Nano-Micro Lett., 2021, 13, 152–169 CrossRef CAS PubMed.
- Y. Ahmed, B. Khan, M. Bilal Faheem, K. Huang, Y. Gao and J. Yang, J. Energy Chem., 2022, 67, 361–390 CrossRef CAS.
- D. Xin, S. Tie, X. Zheng, J. Zhu and W. H. Zhang, J. Energy Chem., 2020, 46, 173–177 CrossRef.
- S. Yun, S. Ma, H. Kwon, K. Kim, G. Jang, H. Yang and J. Moon, Nano Energy, 2019, 59, 481–491 CrossRef CAS.
- T. Bu, J. Li, F. Zheng, W. Chen, X. Wen, Z. Ku, Y. Peng, J. Zhong, Y.-B. Cheng and F. Huang, Nat. Commun., 2018, 9, 4609 CrossRef PubMed.
- S. Yang, S. Chen, E. Mosconi, Y. Fang, X. Xiao, C. Wang, Y. Zhou, Z. Yu, J. Zhao and Y. Gao, Science, 2019, 365, 473–478 CrossRef CAS PubMed.
- J. Yang, W. Tang, R. Yuan, Y. Chen, J. Wang, Y. Wu, W. J. Yin, N. Yuan, J. Ding and W. H. Zhang, Chem. Sci., 2021, 12, 2050–2059 RSC.
- Q. Wang, Q. Dong, T. Li, A. Gruverman and J. Huang, Adv. Mater., 2016, 28, 6734–6739 CrossRef CAS.
- M. Thambidurai, B. Febriansyah, S. Foo, P. C. Harikesh, K. T. Ming, N. Mathews and C. Dang, J. Power Sources, 2020, 479, 228811 CrossRef CAS.
- H. Si, C. Xu, Y. Ou, G. Zhang, W. Fan, Z. Xiong, A. Kausar, Q. Liao, Z. Zhang, A. Sattar, Z. Kang and Y. Zhang, Nano Energy, 2020, 68, 104320 CrossRef CAS.
- X. D. Ding, H. X. Wang, Y. W. Miao, C. Chen, M. D. Zhai, C. S. Yang, B. Y. Wang, Y. Tian and M. Cheng, ACS Appl. Mater. Interfaces, 2022, 14, 3930–3938 CrossRef CAS.
- H. V. Quy, D. H. Truyen, S. Kim and C. W. Bark, ACS Omega, 2021, 6, 16151–16158 CrossRef CAS.
- V. G. Dutt, S. Akhil, R. Singh, M. Palabathuni and N. Mishra, J. Phys. Chem. C, 2022, 126, 9502–9508 CrossRef CAS.
|
This journal is © The Royal Society of Chemistry 2024 |