DOI:
10.1039/D4PM00176A
(Review Article)
RSC Pharm., 2024,
1, 928-948
Advances in liposomal nanotechnology: from concept to clinics
Received
10th June 2024
, Accepted 15th October 2024
First published on 28th October 2024
Abstract
Liposomes, spherical phospholipid vesicles with a unique morphology mimicking that of body cells, have emerged as versatile nanoparticles for drug delivery. Their biocompatibility, low cytotoxicity, targeted delivery, and hydrophobic and hydrophilic characteristics make them stand out over traditional drug delivery systems. Liposomes can be tailored in size, composition, lamellarity, and surface charge, offering a unique level of customization for various applications. Extensive research in liposome technology has led to the development of a wide range of liposomal formulations with enhanced functionalities, such as PEGylated liposomes, ligand-targeted liposomes, and stimuli-responsive liposomes. Beyond their crucial role in cancer treatment, liposomes play a significant role in influenza, COVID-19, cancer, and hepatitis A vaccines. They are also utilized in pain management, fungal treatment, brain targeting, and topical and ocular drug delivery. This review offers insight into the types of liposomes, their composition, preparation methods, characterization methods, and clinical applications. Additionally, it discusses challenges and highlights potential future directions in liposome-based drug delivery.
1. Introduction
Drug Delivery Systems (DDSs) have improved the pharmacological attributes of free drugs by sequestering them in a nano-vehicle and delivering them to the required site. They also minimize the cytotoxicity of drugs and improve their biodistribution.1–3 Several types of nanocarriers ranging in size between 10 to 500 nm are used in DDSs for several purposes,4 including nanocrystals, hydrogels, dendrimers, nanotubes, micelles, solid lipid nanoparticles, ceramic nanoparticles, nanosuspensions, nanowires, and liposomes.5 Liposomes are a safe and practical choice for encapsulating various drugs with their biocompatibility, low toxicity, biodegradability, and hydrophobic and hydrophilic characteristics.6 Furthermore, the toxicity of liposomes has been found to be lower than other nanoparticles.7 They were first discovered in the 1960s by Alec Bangham while researching the nature of cell membranes and the effect of phospholipids on blood clotting. While conducting his experiments, he accidentally observed that liposomes formed spontaneously when phospholipids were dispersed in water.8,9 Currently, liposomes are being heavily researched to treat various diseases, including fungal and viral infections and cancer, besides being used in vaccinations.1 This review describes liposome types, their composition, preparation methods, characterization methods, and clinical applications and highlights the challenges and future prospects.
2. Types of liposomes
2.1. Liposomes’ formulation
Liposomes have a spherical shape and range in diameter between 50–500 nm. Their amphiphilic properties allow them to assemble into bilayer membranes in an aqueous environment, creating a closed bilayer structure of polar hydrophilic heads and non-polar hydrophobic tails, as shown in Fig. 1.6 The hydrophilic heads contain the phosphate group facing the inner and outer aqueous medium. In contrast, hydrophobic tails are chains of fatty acids centered within the bilayer and face each other to create a water-free zone.7 This formulation allows the liposomes to accommodate hydrophobic drugs in their lipid bilayer and hydrophilic drugs in their core.6,7
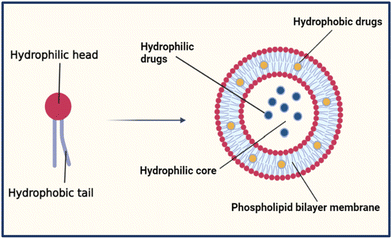 |
| Fig. 1 Schematic representation of the liposome structure and encapsulation of drug molecules. | |
The biocompatibility of liposomes relies on the fact that they have a similar structure and composition to the cell membrane in the human body. They are made of natural phospholipids extracted from animal tissues like egg yolk and bovine brain or vegetable oils like soybean and cotton seed. Liposomes could also be made up of semi-synthetic or synthetic phospholipids. The two main natural phospholipids used in the synthesis of liposomes are glycerophospholipids and sphingomyelin. The head group (denoted as X) in the glycerophospholipids can be varied to form different types of phospholipids like phosphatidylethanolamines (PE), phosphatidylcholines (PC), phosphatidylglycerols (PG), phosphatidylserines (PS), phosphatidic acid (PA), cardiolipin (CL), and phosphatidylinositol (PI) with a backbone of glycerol as shown in Table 1. Moreover, sphingomyelins have a sphingosine backbone, as shown in Table 1.8 Additionally, cholesterol is incorporated into liposomes to enhance their in vivo stability and permeability. It also reduces the membrane fluidity and limits their interactions with plasma proteins, minimizing the encapsulated drug's loss.6,9 Furthermore, polyethylene glycol (PEG), a hydrophilic polymer, is beneficial for altering the liposome's biodistribution profile and pharmacokinetics.10
Table 1 The two main natural phospholipids used in liposome synthesis and common head groups found with glycerophospholipids. Retrieved and edited from ref. 11–13
Basic glycerophospholipid |
|
Head-group (x) substituent
|
Phospholipid name
|
–H |
Phosphatidic acid (PA) |
|
Phosphatidylethanolamines (PE) |
|
Phosphatidylcholines (PC) |
|
Phosphatidylserines (PS) |
|
Phosphatidylglycerols (PG) |
|
Phosphatidylinositol (PI) |
|
Cardiolipin (CL) |
Sphingomyelin
|
|
2.2. Size and lamellarity
Based on the size and number of the bilayers, liposomes are classified into unilamellar, multilamellar (MLV >500 nm), and multivesicular vesicles (MVV >1000 nm), as shown in Fig. 2. Unilamellar vesicles can be further divided into small unilamellar vesicles (SUVs, 25–50 nm), large unilamellar vesicles (LUVs, 100 nm–1 mm), and giant unilamellar vesicles (GUVs, 1–200 mm).14,15 Generally, liposomes must be prepared with a specific size to allow their absorption into body cells. MVVs and MLVs are commonly used for long-term drug delivery effects. However, SUVs have steady drug encapsulation, release kinetics, and prolonged circulation time in the body, making them beneficial for chemotherapy and vaccines.16 Dynamic light scattering (DLS) and Nanoparticle tracking analysis (NTA) are the most used techniques to measure liposome size.17
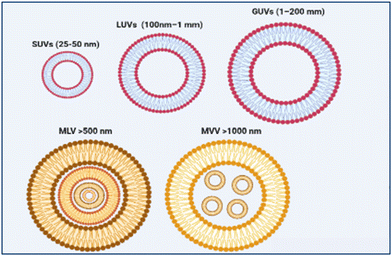 |
| Fig. 2 Liposomes classes based on size and lamellarity. | |
Based on their application and composition, liposomes can be classified into six primary types: conventional, stealth, charged, bubble, actively targeted, and stimuli-responsive liposomes.
2.2.1. Conventional liposomes.
Conventional liposomes are mostly made up of phospholipids, natural or synthetic, with or without cholesterol,18 and have limited stability, making them easily absorbed and removed by the reticuloendothelial system (RES) in the body.19 They have a very short blood circulation time as they can be taken up by the mononuclear phagocytic cells shortly after being administered intravenously.20 The mononuclear phagocytic system prevents conventional liposomes from accumulating at the target region, causing a rapid accumulation in the spleen and liver.21 For this reason, sterically stabilized liposomes (stealth liposomes) were researched and proved to have a prolonged circulation time and increased drug effectiveness compared to conventional liposomes.22
2.2.2. Stealth liposomes.
Stealth liposomes were introduced in the 1970s as second-generation liposomes. They are surface-modified with hydrophilic polymers like PEG, polysaccharides, or glycoproteins to achieve enhanced pharmacokinetics and better accumulation at the targeted site. This is done by coating the liposome's surface with a steric barrier,23 minimizing opsonization in which liposomes are marked for elimination by the mononuclear phagocytic system.23,24 Additionally, they have enhanced biological stability and reduced leakage of the encapsulated drug.23 Stealth liposomes showed efficiency in both chemotherapy and gene therapy.25
2.2.3. Charged liposomes.
N-[1(2,3-Dioleoyloxy) propyl]-N,N,N-trimethyl-ammonium chloride (DOTMA) is a cationic lipid used to synthesize cationic liposomes, while oleic acid is used for anionic liposomes. Generally, charged liposomes demonstrate enhanced stability during storage because charged particles repel each other, minimizing aggregation.1 In chemotherapy, cationic liposomes tend to accumulate in newly formed tumor vessels, enhancing their antitumor effect. Jung and colleagues developed PEG-complexed cationic liposomes (PCL) loaded with doxorubicin and compared them to Doxil®, the first liposomal drug formulation approved in 1995. In vitro cellular uptake results, evaluated by fluorescence microscopy and flow cytometry, showed higher PCL cellular uptake and cytotoxicity against B16F10 cells (murine melanoma cell line from mice) than Doxil®. Moreover, in vivo anticancer activity of the same PCL against the human SKOV-3 ovarian adenocarcinoma xenograft model was comparable to Doxil®; however, PCL showed a slightly reduced plasma level of doxorubicin compared to Doxil® in a pharmacokinetic study in rats.26 Furthermore, Chi and colleagues developed redox-responsive liposomes functionalized with hyaluronic acid (HA). These liposomes were loaded with doxorubicin and coated with HA, which is negatively charged and serves as a targeting ligand for the CD44 receptor (hyaluronan receptor). The innovative aspect of these liposomes is their ability to destabilize under reducing conditions or low pH environments. This feature significantly enhanced cytotoxic effects in treating osteosarcoma in vitro with the MG63 cell line and in a murine xenograft model.27 Nevertheless, increasing the cationic lipids in the liposome formulation suppresses the Enhanced Permeability and Retention (EPR) effect, the mechanism by which liposomes accumulate in tumor tissues more than in healthy tissues due to the leaky nature of tumor blood vessels. Hence, it causes aggregation by the electrostatic interactions of liposomes with anionic compounds present in the blood. Cationic liposomes have also been effective in gene therapy as they successfully encapsulate nucleic acid by exploiting the electrostatic interactions between their positive charge and the negatively charged nucleic acids.28 Moreover, anionic liposomes are used in transdermal drug delivery. Studies showed that anionic liposomes diffuse through the stratum corneum in the skin faster than the cationic liposomes, contributing to enhanced drug penetration through the skin.29 However, anionic liposomes have a faster clearance rate and are less stable in the bloodstream than cationic liposomes.1
2.2.4. Bubble liposomes.
Bubble liposomes are submicron-sized microbubbles that constitute a coat and a gas-filled core, such as air, nitrogen, or oxygen. The coating could be galactose, lipids, proteins, surfactant, silver, or biopolymer; however, lipids are the most common shell material for bubble liposomes. They are intrinsically echogenic and can be utilized as carriers that can deliver and discharge their contents at the targeted site when exposed to ultrasound through the cavitation mechanism. Ultrasound waves introduce oscillations in bubble liposomes, causing a disruption in their membrane and leading to drug release at the targeted site.30,31 Moreover, microjets are formed in their surrounding fluid upon applying ultrasound to bubble liposomes, creating pores in the membrane of targeted cells, a mechanism called sonoporation. These transient pores allow the liposome's contained materials, like siRNA, plasmid DNA, proteins, or drugs, to enter the cell. This makes bubble liposomes beneficial in the fields of DDSs and gene delivery. Moreover, the combination of bubble liposomes and ultrasound can also be used to deliver antigens in dendritic cell-based cancer immunotherapy, hence preventing cancer from metastasizing.32 Additionally, bubble liposomes containing nitric oxide (NO) and oxygen are novel techniques in intravenous therapy. NO bubble liposomes are used as vasodilators and have many other applications. Encapsulating NO in bubble liposomes prevents them from interacting with hemoglobin, enhancing the effectiveness and stability of NO administration. Furthermore, oxygen bubble liposomes have proven effective at supplying high oxygen concentrations to the lungs. Hence, they can be used to overcome hypoxic conditions in tumors, which contribute to higher resistance to chemotherapy in some tumors.33 These developments eliminate the drawbacks of conventional techniques and can potentially improve treatment results.1,30
2.3. Actively targeted liposomes
Actively targeted liposomes are considered third-generation liposomes, primarily used in chemotherapy. Generally, liposomes concentrate at the tumor site through the EPR effect, in which tumors possess a leaky vasculature and enhanced permeability to maximize the uptake of oxygen and nutrients and proliferate. Additionally, tumors have slow venous return and poor lymphatic drainage, making liposomes accumulate even more.34 In other words, liposomes can passively target tumors by the EPR effect. However, the drawback of passive targeting is that it does not discriminate between cancerous and healthy cells.1 Therefore, actively targeted liposomes were developed to target cancerous cells through the receptor–ligand mechanism. This mechanism is achieved by conjugating certain types of ligands, called recognition moieties, to the surface of liposomes. These ligands are specific to the angiogenic endothelial cells or the tumor cells and bind to the receptors they overexpress, enhancing the tumor-targeting.35 Recognition moieties can be antibodies, nucleic acid, carbohydrates, vitamins, or simple peptides.1 The difference between active and passive targeting mechanisms is illustrated in Fig. 3 below. Furthermore, Maruyama et al. developed PEGylated immunoliposomes with attached antibodies specifically for lung endothelial cells in 1999. Their results showed that these liposomes had high targetability and effectively avoided the RES.36 Furthermore, Moghimipour et al. synthesized folate-conjugated liposomes loaded with 5-fluorouracil (5FU) in another study. In vitro studies on colon carcinoma (CT26) cells proved that these liposomes have higher cellular uptake and tumor inhibition compared to the free drug. Moreover, Zhang et al. synthesized integrin β3 specific ligand (B3int)-modified liposomes loaded with DOX. Prostate cancer, including PC-3 and DU-145 cell lines, were used to perform in vitro cellular uptake studies. The results showed that the prepared liposomes had higher uptake in PC-3 cells with a three-fold increase in cellular DOX compared to DU-145 cells.37
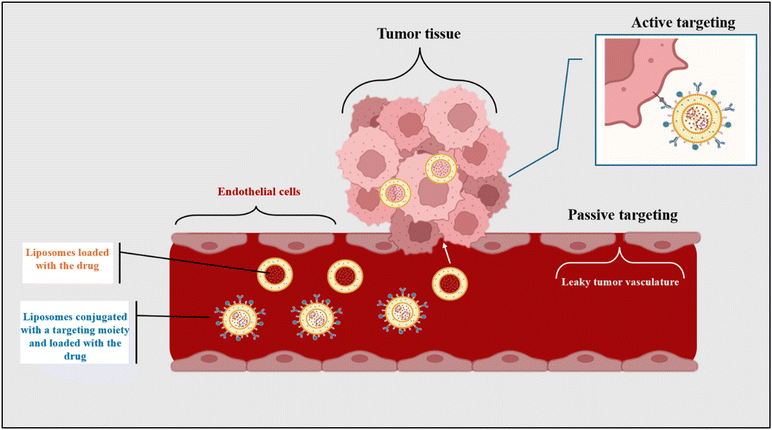 |
| Fig. 3 Schematic representation of the passive and active targeting mechanisms. | |
2.4. Stimuli-responsive liposomes
Stimuli-responsive liposomes release their content at the targeted site through exogenous (external) or endogenous (internal) stimuli. The internal stimuli could be pH change, unique enzymatic activity, or redox reactions. In contrast, the external stimuli could be light, heat, ultrasound, or magnetic fields, as shown in Fig. 4.38Table 2 lists the most common types of stimuli-responsive liposomes and their working principle.
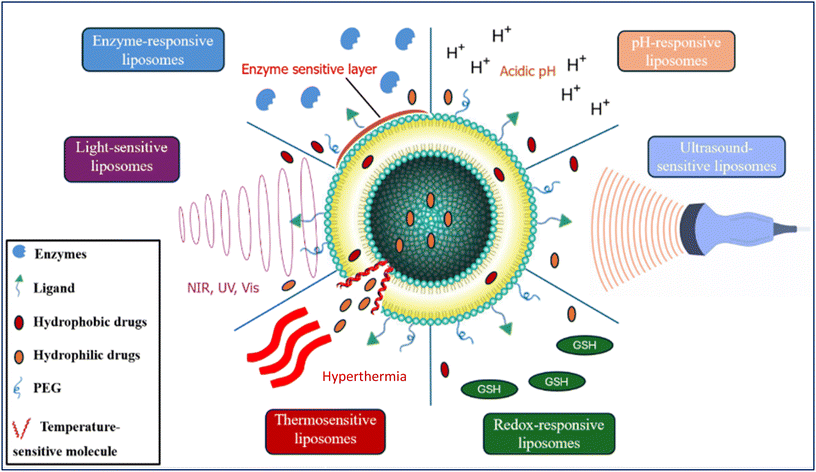 |
| Fig. 4 Different types of stimuli-responsive liposomes. | |
Table 2 The most common types of stimuli-responsive liposomes and their working principle
Liposome type and trigger |
Principle |
Ref. |
pH-Responsive liposomes
|
These liposomes are stable in neutral pH but become unstable in acidic environments, and they are commonly found in some pathological sites in the body. The pH change triggers the liposome's functional groups to be protonated, causing liposome instability and drug release |
38
|
-pH change |
|
|
Redox-responsive liposomes
|
Pathogenic sites in the body have higher amounts of glutathione (GSH), which acts as a reducing agent and donates a hydrogen atom to the liposomes. These liposomes have disulfide bonds in their structure, and upon receiving a hydrogen atom, these bonds are reduced, causing their cleavage and triggering drug release at the targeted site |
39 and 40 |
-Electron transfer reaction |
|
|
Enzyme-responsive liposomes
|
These liposomes utilize the fact that some enzymes are overexpressed in diseased cells. Generally, they rely on the cleavage of amides or esters by proteases or esterases to alter the liposome's lipid bilayer and release the drug at the targeted site |
41
|
-Elevated enzyme expression |
|
|
Light-sensitive liposomes
|
During synthesis, photoreactive groups are added to the phospholipid bilayer. These liposomes become unstable after applying a light source and release their content at the targeted site |
42 and 43 |
-Ultraviolet (UV), near-infrared (NIR), and visible (Vis) light |
|
|
Thermosensitive liposomes
|
When these liposomes are exposed to mild hyperthermia (HT), their lipid bilayer transitions from a gel to a liquid-crystalline state. This transition raises the bilayer permeability, facilitating drug release. The lipid bilayer composition determines the required temperature and can be tailored to achieve HT, typically between 40 and 43 °C. Moreover, magnetic field-sensitive liposomes are considered a type of thermosensitive liposomes. A paramagnetic agent like iron oxide is incorporated in these liposomes, and an AMF is used to generate magnetic hyperthermia that leads to drug release at the targeted site |
44–46
|
-Mild hyperthermia by: water baths, electromagnetic applicators, ultrasound, magnetic resonance-guided focused ultrasound, radiofrequency, laser fibers, microwave, and alternating current magnetic field (AMF) |
|
|
Ultrasound-sensitive liposomes
|
Drug release is achieved by applying high-intensity focused ultrasound to liposomes, causing drug release through several phenomena: |
47
|
-High-intensity focused ultrasound |
1. Cavitation: Gas bubbles are formed and collapse suddenly near the liposomes, disrupting them and releasing their content |
|
2. Acoustic streaming: The radiation forces caused by the US waves displace the liposomes and destabilize them, causing drug release |
|
3. Microbubble nucleation: This may also occur due to cavitation. It involves the growth of microbubbles inside the liposomes, causing drug release when US is applied |
|
4. Hyperthermia: The US energy is dissociated to thermal energy, causing localized heat that leads to drug release |
|
2.4.1. pH-Responsive liposomes.
Vila-Caballer and colleagues developed liposomes with the pH-responsive polymer stearoyl–PEG–polySDM. These liposomes provide a vehicle for delivering bovine serum albumin (BSA) to the bladder epithelium. The study results showed a significant increase in BSA uptake by the MB49 cells (mouse bladder carcinoma cells) at an acidic pH (6.5) compared to a neutral pH (7.4). Moreover, at pH 6.5 and 7.4, control liposomes failed to deliver BSA to the bladder epithelium. In contrast, the pH-responsive liposomes successfully delivered BSA to MB49 cells at pH 6.5, suggesting potential for targeted bladder cancer therapy.37,48
2.4.2. Redox-responsive liposomes.
Wang et al. synthesized redox-responsive liposomes based on a disulfide-derivative paclitaxel-ss lysophosphatidylcholine prodrug (PTX-ss-PC). The in vitro cytotoxicity of the liposomes conducted on lung and breast cancer cells revealed that these PTX-ss-PC liposomes are promising in inhibiting tumor growth mediated by the presence of GSH.37,49
2.4.3. Enzyme-responsive liposomes.
Pourhassan et al. synthesized secretory phospholipase A2 (sPLA2) sensitive liposomes loaded with oxaliplatin (L-OHP). Nude mice bearing sPLA2-deficient FaDu squamous carcinoma and sPLA2-expressing Colo205 colorectal adenocarcinoma were used to evaluate these liposomes’ in vivo therapeutic efficiency. Their study showed that using sPLA2-sensitive liposomes did not enhance drug efficiency compared to low- or non-sensitive liposomes, and the mice did not show more prolonged survival. In contrast, these sPLA2-responsive liposomes accumulated in the liver and posed a risk of systemic toxicity and liver failure due to their high sensitivity and repeated dosage to reach an effective therapeutic window. Moreover, reducing their toxicity comes at the expense of lowering their sensitivity, which caused them to lack an actively sPLA2-controlled released mechanism, thus behaving similar to conventional pegylated liposomes.50
2.4.4. Light-sensitive liposomes.
Chen et al. synthesized a NIR-responsive bubble-generating thermosensitive liposome (BTSL) system loaded with Cypate, a photothermal agent, DOX, and NH4HCO3. The in vitro release study demonstrated that after NIR irradiation, there was an increase in DOX released from BTSL compared to (NH4)2SO4 liposomes at 42 °C. Moreover, the in vivo study results showed that the system highly increased the accumulation of DOX in tumors, inhibiting its growth and reducing the DOX side effects.51
2.4.5. Thermosensitive liposomes.
Derieppe et al. monitored the penetration of doxorubicin released from thermosensitive liposomes in the subcutaneous rat R1 rhabdomyosarcoma xenograft. A water bath with a temperature of 43 °C and fibered confocal fluorescence microscopy (FCFM) were used for the setup. The tumor-bearing leg was immersed in the water bath, and the liposomes were injected intravenously. The results from the FCFM showed an increase in the fluorescence signal in the tumor cells, indicating an increase in doxorubicin concentration at the targeted site.52 Furthermore, Lu et al. developed magnetic and thermally responsive thermosensitive magnetic liposomes (TML) loaded with citric acid-coated magnetic Fe3O4 and Camptosar (CPT-11). The liposomes were surface-conjugated with Cetuximab (CET) to target epidermal growth factor receptors on human primary glioblastoma (U87) cells. The in vitro results revealed a rise in the TML–CPT-11–CET solution temperature after applying AMF and enhanced drug release, contributing to the enhanced cytotoxicity of CPT-11 against the cancer cells. Moreover, the in vivo therapeutic efficacy was confirmed from PET/MRI studies in mice orthotopic xenograft brain tumor models.53
2.4.6. Ultrasound-sensitive liposomes.
Deng et al. synthesized iRGD-conjugated low temperature-sensitive liposomes loaded with DOX (iRGD–LTSL–DOX) to explore their effect with high-intensity focused ultrasound (HIFU) in targeting mammary carcinoma cells (4T1), human breast adenocarcinoma (MCF-7), and human umbilical vein endothelial (HUVEC) cells. The in vivo results showed that DOX was intravascularly released from the liposomes and penetrated the tumor upon applying HIFU.37,54
3. Liposome preparation methods
3.1. Organic solvents methods
Several methods are used to synthesize liposomes using organic solvents like chloroform, ether, acetone, ethanol, and methanol. These include reverse-phase evaporation, thin-film hydration, ethanol injection, detergent removal methods, and microfluidics methods, as listed in Table 3.
Table 3 Organic-solvent preparation methods of liposomes
Method |
Preparation technique |
Advantages |
Disadvantages |
Ref. |
Reverse-phase evaporation |
Lipids and amphiphilic molecules are dissolved in an organic solvent and mixed with an aqueous buffer containing the water-soluble drug to be encapsulated. During the sonication of the mixture, inverted micelles are formed. A rotary evaporator then evaporates the organic solvent under low pressure, causing micelles to become viscous. Some collapse at a critical point, creating a bilayer around the remaining micelles and forming liposomes. The product is a mixture of MLVs and LUVs, and extrusion using an extruder with a polycarbonate membrane can then be used to reduce the size of the liposomes |
-Easy to prepare |
-Difficult to remove the organic solvent due to its contact with the encapsulated material |
1, 14 and 54–56 |
|
-Used widely |
-A possibility of denaturation of peptides due to sonication conditions |
|
|
-Large molecule encapsulation like nucleic acids and proteins |
|
|
Thin-film hydration (Bangham method) |
This is the most common method for preparing liposomes. Lipids and amphiphilic molecules are dissolved in an organic solvent and placed in a round-bottom flask. A rotary evaporator then evaporates the organic solvent under vacuum, forming a thin lipid film on the wall of the flask. The hydration of the thin film then takes place by adding an aqueous solution that may contain the drug needed to be encapsulated, followed by sonication using a sonication bath. This leads to MLVs formation, which can be extruded to form unilamellar liposomes of a specific diameter. Fig. 5 illustrates the steps of preparing liposomes using the thin-film hydration method |
-Easy to prepare |
-Heterogeneous MLVs are formed |
1, 5, 14, 15, 54 and 57 |
|
-Used widely |
-Water soluble drugs have inferior encapsulation efficiency |
|
|
|
-Poor repeatability and inappropriate for mass production |
|
|
|
-Time-consuming |
|
Solvent injection (ethanol/ether) |
This method may be done using several organic solvents. The phospholipids and the drug can be dissolved in ethanol and injected into a water phase with a high stirring speed, as shown in Fig. 6. The organic solvent is then removed by filtration or a rotary evaporator to form liposomes. Another organic solvent, diethyl ether, has a low boiling point and can dissolve lipids and other amphiphilic molecules. The solution is then injected into an aqueous solution with a higher boiling point than the ether, allowing it to evaporate and form liposomes, mostly LUVs |
-Suitable for the preparation of large liposomal formulation volumes |
-High polydispersity index (PDI) |
1, 14, 15, 54 and 58 |
|
|
-MLVs are present in most cases |
|
|
|
-Low encapsulation efficiency |
|
|
|
-Low stability due to the high temperatures and use of organic solvents |
|
Detergent removal method |
Detergents with high critical micelles concentration (CMC) and lipids are dissolved in an organic solvent. After solvent evaporation under low pressure, a thin film lipid layer is created in the round bottom flask, which is then hydrated using an aqueous solution. The detergent is finally removed by dialysis, dilution, size-extrusion chromatography, or adsorption onto hydrophobic beads |
-LUVs with high capacity and uniform size are formed |
-Hydrophilic drugs detached from liposomes during detergent removal |
1, 15, 54 and 58 |
|
-Easy to prepare |
-Low liposome concentration in the final solution |
|
Microfluidic methods |
This versatile method allows the manipulation of microfluidics in microchannels, usually 5–500 μm cross-sectional dimension. Lipids are dissolved in an organic solvent and then injected into microchannels in the opposite direction to the aqueous solution inside the microchannels. Continuous mixing of the organic solvent and the aqueous solution forms liposomes. A popular method is microfluidic hydrodynamic focusing (MHF), in which lipids are dissolved in alcohol in a central channel connected to two vertical side channels containing an aqueous solution. When the alcohol gets mixed with and diluted by the aqueous solution, the lipids self-assemble, forming liposomes |
-Proper liposome polydispersity, morphology, average size, and lamellarity |
-High production cost of the micro-circuits |
1, 14, 15, 59 and 60 |
|
|
-Low concentration of liposomes in the final solution (MHF) |
|
3.2. Organic solvent-free methods
Several methods are used to synthesize liposomes without organic solvents. These include heating, dehydration–rehydration, pH jumping, and supercritical fluidic method, as listed in Table 4.
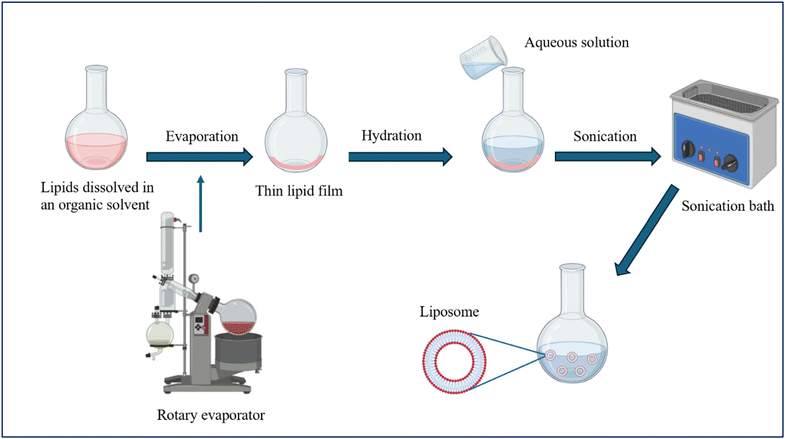 |
| Fig. 5 Thin-film hydration method of liposomes preparation. | |
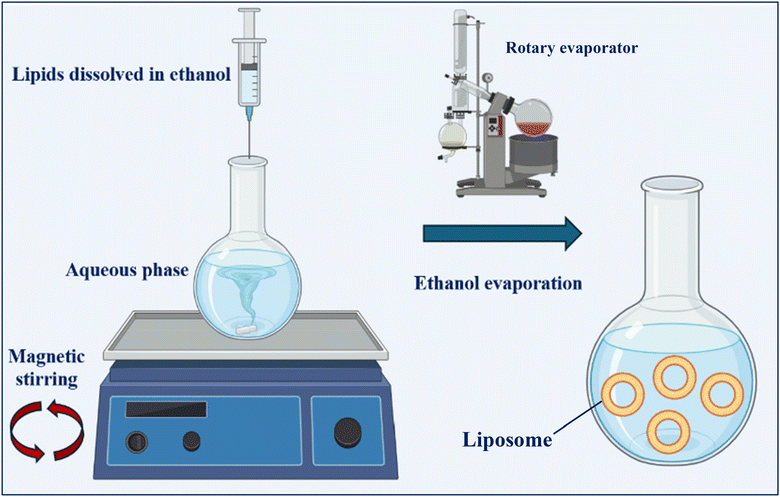 |
| Fig. 6 Solvent injection method of liposomes preparation using ethanol. | |
Table 4 Organic-solvent free preparation methods of liposomes
Method |
Preparation technique |
Advantages |
Disadvantages |
Ref. |
Heating method |
Phospholipids are directly hydrated with an aqueous solution at 60 °C or 120 °C and continuously stirred for an hour |
-Fast and easy |
-High temperatures are required |
1, 15, 61 and 62 |
Hydration agents like propylene glycerol or glycerin are added as stabilizers and isotonising agents that prevent the liposomes from coagulating |
-No toxic solvents used; no need to remove the solvent from the final solution |
-Drug and phospholipid degradation |
|
Dehydration-rehydration method |
Lipids and other amphiphilic molecules are directly dispersed in an aqueous solution that may contain the drug to be encapsulated. Water is then evaporated (dehydration step) under nitrogen in a rotating flask, forming a multilamellar film that sandwiches the drug molecules. Water is then added (rehydration step), forming LUVs |
-Easy to prepare |
-Synthesized liposomes have high PDIs (heterogenous) |
1, 54 and 63 |
|
-No toxic solvents; no need to remove the solvent from the final solution |
|
|
|
-Suitable for large-scale production |
|
|
pH jumping method |
Phospholipids are dispersed in an aqueous solution containing phosphatidylcholine and phosphatidic acid, then subjected to a 3.5-fold increase in pH for a short time (less than 2 min), forming SUVs. Increasing the phosphatidic acid to phosphatidylcholine ratio yields a higher percentage of SUVs versus LUVs |
-Easy and quick |
-Need for precise pH levels |
1, 54, 59, 64 and 65 |
|
-Suitable for large-scale production |
-Potential damage or denaturation of sensitive molecules |
|
|
-Highly stable SUVs |
|
|
Supercritical fluidic method |
A supercritical fluid like scCO2 dissolves the lipids. It is non-condensable, non-toxic, and non-inflammable and has a low critical temperature and pressure. A liquid pump continuously feeds the aqueous phase into a cell containing the supercritical lipid solution, enabling the phase transition of the dissolved phospholipids. After all CO2 has been removed, liposomes form due to the sudden drop in pressure during depressurization |
-High encapsulation efficiency |
-High cost despite scCO2 having low cost |
1, 15, 66 and 67 |
|
-Suitable for low-solubility drugs |
-Low yield |
|
|
-Controlled liposome size |
|
|
3.3. Post-preparation handling methods
The following techniques are used after liposome synthesis to improve the formation of uniform and unilamellar vesicles, enhance lipid packing, or increase the shelf-life of liposomes.
3.3.1. Freeze-drying (lyophilization).
This method prolongs the shelf-life of prepared liposomes by preserving them in a dry and stable state. It prevents the liposomes from physical degradation during storage and prevents the hydrolysis of phospholipids.68 Several cryoprotectants, including trehalose, lactose, and glucose, can be mixed with the prepared liposomes and then frozen at −30 °C. The mixture is then freeze-dried under a vacuum at −10 °C for 24 hours, resulting in fluffy solid particles that can be rehydrated with phosphate-buffered saline at room temperature upon use.1,69
3.3.2. Freeze–thaw cycles.
This technique can be used after any preparation method to enhance lamellarity and drug encapsulation efficiency. The process starts with freezing the liposomes in liquid nitrogen (−196 °C) and then leaving it to melt at room temperature. Vesicles fuse and form LUVs when the solution thaws, and the process can be repeated up to 10 times to get the required results. Moreover, pores are formed in the lipid bilayer, enhancing the permeability and allowing enhanced drug encapsulation when the drug is added.55,70
3.4. Drug loading
There are two techniques of drug loading inside the liposomes, including passive and active loading techniques. The drug is encapsulated during the liposome synthesis process in the passive loading, while it is loaded after liposome formation in the active loading technique. Hydrophobic drugs are encapsulated in the lipid bilayer, and the hydrophilic drugs are encapsulated in the aqueous core in passive loading. The main drawback of this method is low encapsulation efficiency; hence, free drugs must be removed. Several marketed liposomes, including AmBisome®, Expel®, DepoCyte®, and DepoDur®, are prepared through the passive loading technique.71 Moreover, the active loading technique, also called remote loading, transforms the drug from being membrane-permeable to impermeable to trap it inside the liposomes.55 Loading the drug remotely can be done using several techniques, such as citric gradient, ammonium sulfate gradient, and pH gradient.5 All of these techniques follow the same principle. Upon the diffusion of free drugs inside the liposomes, they are modified to entrap them inside the liposomes due to the inhibition of membrane permeation.72 Several FDA-approved liposomes, including Doxil®,5,72,73 DuanoXome©, and Myocet™,5,72 are synthesized using the active loading technique.55
4. Characterization methods of liposomes
Several methods have been used to evaluate the efficacy of liposome formulation by characterizing different physiochemical parameters. These include size and size distribution, shape and morphology, encapsulation efficiency, lamellarity, surface properties, release profile, and phase behavior. Table 5 summarizes the most common characterization techniques.
Table 5 Characterization methods used for the evaluation of different liposome parameters
Studied attribute |
Characterization technique |
Ref. |
Size and size distribution (polydispersity index)
|
-Dynamic Light Scattering (DLS): A diluted sample of liposomes is used to measure the diameter and the polydispersity index. The principle is based on the change in the light intensity scattered from the liposome vesicles upon applying a light source like a laser beam |
74–76
|
Measuring the mean hydrodynamic radius (Rh) and the broadness of the vesicles’ size distribution |
-Transmission Electron Microscopy (TEM): A highly accelerated electron beam (100–1000 keV) is passed through the liposomes to analyze their size. Several types of TEMs exist, including cry-TEM (visualizes the liposomes in their native state without staining) and freeze-fracture TEM (visualizes the internal and external structures of the liposomes) |
|
-Scanning Electron Microscopy (SEM): A high-energy focused beam of electrons (20 keV) analyzes the size of liposomes. |
|
Shape and morphology
|
TEM, SEM, and Atomic Force Microscopy (AFM) evaluate the shape and morphology of liposomes |
77 and 78 |
-AFM: A scanning probe microscope technique used to take high-resolution 3D images of liposomes. Due to the sample features, a sharp-tip cantilever moves up and down on a sample (liposomes). An optical sensor then senses the interaction and surface forces between the tip and the sample surface. It draws a detailed image showing the liposomes’ surface modifications and detects ligands |
|
Encapsulation Efficiency (EE)
|
The process involves preparing the liposomes and then separating the encapsulated drug from the free, unencapsulated drug. This separation is achieved through centrifugation, ultrafiltration, or dialysis. After separation, the amount of drug within the liposomes is measured using analytical methods such as spectrophotometry or chromatography. The encapsulation efficiency is then expressed as a percentage, calculated by dividing the amount of drug encapsulated by the total drug amount and multiplying by 100 |
15 and 79 |
EE is the ratio of the drug successfully encapsulated within the liposome vesicles compared to the total amount of drug initially used |
|
|
Release profile
|
The process starts with preparing the drug-loaded liposomes in a buffer solution. The liposomes are then subjected to a triggering mechanism. Samples are taken from the solution, and the amount of drug released is measured using UV-vis spectrophotometry, High-performance liquid chromatography (HPLC), or Liquid chromatography-mass spectrometry (LC-MS). The data collected are plotted as a cumulative percentage of drug released versus time, providing a detailed profile of the release kinetics and mechanism. This profile is crucial for predicting the behavior of liposomal formulations in biological systems and optimizing their therapeutic efficacy |
77 and 80 |
Release profile is the amount of drug released from liposomes |
|
|
Surface charge (zeta potential)
|
Liposome surface charge can be measured through DLS and Electrophoretic mobility to evaluate their stability |
1 and 74 |
Measures the electrical charge on the surface of liposomes, which significantly influences their colloidal stability |
-Electrophoretic mobility: An electric field is applied across the sample, and the particles’ velocity is measured as they migrate to the electrode of opposite charge. The velocity is proportional to the magnitude of the zeta potential |
|
Phase transition temperature behavior
|
-X-ray Diffraction (XRD): A liposome sample is placed in a glass capillary and exposed to X-rays that interact with the electrons within the liposomes. The X-rays get diffracted after striking the electrons, resulting in a diffraction pattern that changes with the gel-to-liquid phase transition or vice versa |
80–83
|
Measure the changes in the lipid bilayer properties as a response to temperature change |
-Differential Scanning Calorimetry (DSC): A liposome sample is subjected to an increase in temperature, and as heat is absorbed, the liposomes shift from gel to liquid phase. This process occurs at a melting temperature (Tm) that varies depending on the lipid composition of the vesicles. The liposomes could also be subjected to a decrease in temperature, where the heat is released, and they shift from liquid to gel phase |
|
Lamellarity
|
TEM, SEM, XRD, and Phosphorous-31 Nuclear Magnetic Resonance (31P-NMR) |
1, 82 and 83 |
Number of lipid bilayers |
|
|
5. Clinical uses of liposomes
5.1. Cancer treatment
Cancer is the second leading cause of death globally after heart disease, causing a significant impact on public health.6 According to an estimate by the American Cancer Society and based on incidence data collected by central cancer registries and mortality data collected by the National Center for Health Statistics, 2
001
140 new cancer cases, and 611
720 cancer deaths are projected to occur in the United States in 2024.84 Healthy body cells tend to divide rapidly and uncontrollably, causing tissue failure and many complications in cancer patients. Moreover, genetic disorders, smoking, and environmental carcinogens may cause cancer.84,85 There are many treatment options for cancer; however, the choice depends on factors including the patient's age, comorbidity, and cancer stage. These options include radiation therapy, chemotherapy, hormonal therapy, and immunotherapy. A combination of the mentioned therapies is used in most advanced cancer stages. However, chemotherapy remains one of the most common treatment options as it uses drugs to destroy cancer cells and prevent them from dividing. Despite that, chemotherapy has many toxic side effects, including hair loss, vomiting, fever, anxiety, fatigue, nausea, and many other severe side effects. Furthermore, a common challenge in chemotherapy is the resistance of cancer cells to anticancer drugs. Cancer cells can evade the effect of cancer drugs through several mechanisms, including cell survival by activating specific survival pathways, epigenetic alterations, and multi-drug resistance by pumping the chemotherapy drugs out of the cancerous cells, causing a reduction in their efficiency.6,86,87 Non-specified targeting that causes damage to healthy organs and cells is also a common problem associated with chemotherapy.
For these reasons, there is a pressing demand to address the mentioned drawbacks and find treatment approaches that target cancerous cells with minimal harm to the surrounding healthy cells.14 Nanocarriers, especially liposomes as they are FDA approved, are being investigated heavily in chemotherapy, as they can sequester anticancer drugs and release them at the targeted cancer cells, therefore minimizing the cytotoxicity of healthy cells.5 In addition to providing passive targeting to tumor tissues via the EPR effect, they can be utilized for active targeting by binding with specific site ligands, as described earlier. They also improve the pharmacokinetic effects of the encapsulated drug by reducing the chance of being eliminated and increasing the circulation time.88 Doxil®, also called Caelyx®, was the first liposomal drug formulation that was released in 1995 for the treatment of ovarian cancer, HIV-related Kaposi's sarcoma, and myeloid melanoma.89 Several other products were later approved, like DaunoXome® in 1996 for Kaposi's sarcoma, Myocet® in 2000 for breast cancer, Mepact® in 2009 for osteosarcoma, Marqibo® in 2012 for leukemia,90 Lipo-Dox® in 2013 for ovarian cancer,91 Onivyde® in 2015 for pancreatic adenocarcinoma,90 and Vyxeos® in 2017 for acute myeloid leukemia.92 Furthermore, many liposomal formulations are currently being tested in clinical trials. For example, Lyso-thermosensitive liposomal DOX (LTLD, ThermoDox®), a phase II clinical trial drug for nephroblastoma, liver tumors, and rhabdomyosarcoma, is being studied for its ability to release medication from liposomes using Magnetic Resonance-Guided High-Intensity Focused Ultrasound (ClinicalTrials.gov-study: NCT04791228
93). Moreover, MBP-426, now in phase II clinical trial for treating second-line gastric, gastro-esophageal, or esophageal adenocarcinoma, uses oxaliplatin-loaded, transferrin-conjugated N-glutaryl phosphatidylethanolamine-liposomes combined with leucovorin and 5-FU (ClinicalTrials.gov-study: NCT00964080
94).
5.2. Fungal treatment
When the immune function in the body is suppressed, the body becomes prone to fungal infection. Amphotericin B is the most used lipophilic antibiotic for treating systemic fungal infections.95 However, it has several undesirable side effects, including nephrotoxicity, vomiting, headache, nausea, fever, anemia, and many other severe conditions. For this reason, the encapsulation of this drug in liposomes reduces its toxicity through two main mechanisms: modulating the drug transfer rate from the liposomes to the healthy cells and lowering the drug clearance rate from the bloodstream.96 Liposomes have a high affinity for ergosterol, a sterol found in the fungi cell membrane, causing them to accumulate at the fungi site rather than in healthy cells.95 AmBisome®, a liposomal formulation of amphotericin B, was approved in 1997 to treat systemic fungal infection.89 It can bind to fungal cell walls and release amphotericin B, which is transferred through the cell wall and attaches to ergosterol in the fungal membrane. Moreover, AmBisome® is used to treat extracellular (aspergillosis and candidiasis) and intracellular (histoplasmosis and leishmaniasis) systemic fungal infections.97 In a recent phase III clinical trial, AmBisome® is being compared to olorofim (non-liposomal anti-fungal drug) to treat invasive fungal disease caused by Aspergillus species (ClinicalTrials.gov-study: NCT05101187
98). The FDA also approved Abelcet® in 1995 for treating invasive fungal infections and Amphotec® in 1996 for treating visceral leishmaniasis.56,95,99
5.3. Liposomes in pain management
Multivesicular liposomes comprise many water-filled polyhedral sections divided by bilayer lipid septa. This structure allows them to encapsulate drugs with increased effectiveness. DepoDur® is a liposomal formulation containing morphine and made up using multivesicular liposome technology referred to as DepoFoam®. It was approved by the FDA in 2004 for a single epidural morphine injection in postoperative pain management.100 Moreover, Exparel®, FDA-approved in 2011, is also made up using the DepoFoam® technology to release bupivacaine for postsurgical analgesia. A single-dose injection of liposomal bupivacaine is used at the surgical site to provide up to 72 hours of analgesia for pain relief.101 Moreover, a clinical study, currently in phase IV, evaluates the efficiency of Exparel® compared to standard 25% bupivacaine in decreasing pain medication consumption (ClinicalTrials.gov-study: NCT02499159
102).
5.4. Liposomes in vaccination
5.4.1. Cancer vaccine.
Cancer vaccines aim to boost the body's immune response against cancerous cells. Antigen-presenting cells (APCs) are immune cells that play a crucial role in initiating the body's immune response, including dendritic cells, macrophages, and B-cells. APCs capture tumor antigens and present them to T-cells, which are effector cells that can recognize and kill cancerous cells. However, the specific activation of APCs through oncological vaccines, also known as therapeutic cancer vaccines, poses a technical obstacle.103 Liposomes are being heavily researched as antigen-carrying vehicles for APCs. They can encapsulate tumor-specific antigens in their core or carry them on their surface and then deliver them to the cytoplasm of APCs, thereby initiating an immune response against tumor cells.104 PDS0101 is a cancer vaccine currently in phase I/II trial to study its effect in shrinking tumors in patients with human papillomavirus-associated oropharynx cancer metastasized to lymph nodes. It comprises specific peptides that help the body build an immune response against cancerous cells (ClinicalTrials.gov-study: NCT05232851
105). Another recent study in phase IIA explores the effect of the PDS0101 vaccine, a liposomal vaccine using Cisplatin drug, combined with chemoradiation therapy in treating patients with stage IB3-IVA cervical cancer. The Vaccine contains two active components: one intended to boost the immune response against human papillomavirus viral protein, and the other is a group of peptides taken from the human papillomavirus (ClinicalTrials.gov-study: NCT04580771
106).
5.4.2. Hepatitis A vaccine.
Hepatitis A is characterized by liver inflammation caused by the hepatitis A virus (HAV). It spreads through contaminated food and water containing the feces of an infected person, and it is associated with poor hygiene and sanitation. There are several hepatitis A vaccines available worldwide, providing protection from HAV.107 Virosomes are a type of liposome that act as vaccine carriers, encapsulating specific antigens and presenting them to the immune cells. After treatment with a detergent, they are prepared by removing the protein shell that envelops the viral genetic material from an enveloped virus. The phospholipid bilayer membrane of liposomes is then loaded with the viral membrane protein to facilitate their fusion with immune cells.95 Epaxal® is the first hepatitis A vaccine utilizing virosome technology developed by Crucell Berna Biotech and released in Europe in 1993. HAV was deactivated with formaldehyde and attached to the virosome surface to facilitate the delivery of HAV antigen to immunocompetent cells. The clinical trials on adults showed that more than 95% of the people had anti-HIV titers for up to 20 years after a second booster vaccine.9,108
5.4.3. Influenza vaccine.
The WHO recommends influenza vaccination for acute respiratory infections resulting from the influenza virus. Influenza evolves constantly, and the body's immunity wanes over time; annul vaccinations are recommended to boost the body's immunity against influenza.109 Inflexal® V is based on virosome technology, and it was developed by Crucell Berna Biotech and approved in 1997.9 It differs from the traditional influenza vaccine, which usually contains an attenuated form of the same virus. Inflexal® V contains haemagglutinin (HA), a surface glycoprotein derived from influenza A and B virus strains and incorporated into the virosomes synthesized from synthetic and natural phospholipids.9,110 HA in the influenza virus causes it to attach to respiratory cells, allowing it to replicate in the host. For this reason, it can be encapsulated in the bilayer membrane of the liposomes to guide the virosomes to the APCs, causing the fusion of virosomes with their membrane and initiating an immune response.111 The virosomes used are unilamellar carriers with a size of 150 nm mean diameter, and they are characterized by their low immunogenicity due to their minimal viral protein content. However, despite this low immunogenicity, studies showed that Inflexal® V can initiate a better immune response than traditional vaccines. Furthermore, cephalin is a phospholipid that is incorporated in the liposome bilayer membrane of Inflexal® V. It acts as an adjuvant to boost the immune response as it can independently stimulate B-cells regardless of T-cells and can bind hepatitis A antigen.9,110,111
5.4.4. COVID-19 vaccine.
Coronavirus disease 19 (COVID-19) is caused by severe acute respiratory syndrome coronavirus 2 (SARS-CoV-2). It first appeared in December 2019, causing a global pandemic and massive crisis that affected the economy and global health. Coronavirus is mainly made up of four types of proteins: nucleocapsid (N), membrane (M), envelope (E), and spike (S) protein. The four proteins work together to activate the virus. However, the spike protein is responsible for the attachment and fusion of the host cell receptors and the virus. Two liposome-based vaccines, Pfizer/BioNTech and Moderna, were developed and approved in 2021 for SARS-CoV-2.95 These vaccines work by delivering mRNA entrapped into liposomes to the cytoplasm of the host cells; mRNA is translated into spike protein and acts as an antigen to develop an immune response to SARS-CoV-2.112 The liposomes remain stable in circulating blood and are then taken by the phagocytic cells through endocytosis. Once they enter the cytosol, their membrane destabilizes, releasing mRNA. The mRNA is then translated into spike proteins and expressed on the surface of the infected cells, triggering an immune response and releasing antibodies.112,113 Based on clinical data on Pfizer/BioNTech, with the product name Comirnaty®, the Vaccine offered robust protection from coronavirus with a two-dose regimen offering 95% efficacy for individuals aged 16 years old and above (ClinicalTrials.gov-study: NCT 04368728
114). Moreover, the Moderna vaccine illustrated 94.1% efficacy in preventing COVID-19 and garnered emergency use authorization from the FDA and other global agencies (ClinicalTrials.gov-study: NCT04470427
115).95
5.5. Liposomes in other drug delivery applications
Brain drug delivery is restricted by the presence of the blood–brain barrier (BBB). The BBB comprises endothelial cells joined by tight junctions, forming a selective protective barrier that prevents harmful substances from entering the brain. However, the BBB also prevents many drugs and chemotherapeutic agents from entering the brain. Therefore, finding optimal approaches to cross the BBB and effectively deliver drugs with minimal risk to patients is crucial. Several types of nanoparticles, including micelles, gold nanoparticles, solid–lipid nanoparticles, and liposomes, offer prominent strategies for delivering drugs to the brain, solving the permeation problem associated with traditional drug delivery techniques. Among all, liposomes show great potential as their components are non-immunogenic, biologically inert, and biodegradable, with low inherent toxicity. Many liposomal formulations made it to the market, including Doxil® (Caelyx®) and Myocet® for glioblastoma multiforme, and Abelcet® and AmBisome® for Cryptococcal meningitis, while many others are still under clinical trials.116,117
Apart from the diverse intravenous applications mentioned above, liposomes have become increasingly crucial for delivering drugs transdermally. Using liposomes for such applications offers controlled and targeted delivery of dermatologic compounds like corticosteroids, antibiotics, and retinoic acid to the skin layers. The presence of phospholipids in their lipid layer provides targeted delivery to the stratum corneum and epidermis while reducing systemic absorption. Several studies have evaluated the effect of skin disease-related drugs encapsulated in liposomes and delivered through the topical route.118 Methotrexate (MTX), an immunosuppressant used to treat psoriasis, is known to cause adverse effects, such as mucosal ulceration and cirrhosis, when delivered orally or parenterally over a long term. Topical delivery of MTX showed limited skin penetration and ineffective treatment for patients with psoriasis. Ali et al. developed MTX-loaded liposomes for treating localized psoriasis and compared them to plain MTX gel. In a double-blind study on albino mice, the gels were applied daily, followed by irradiation with a 650 nm diode laser at 80 joules. Results showed 3–4 times higher skin permeation than conventional MTX cream, providing an effective and safer topical treatment for psoriasis and avoiding the adverse effects of systemic MTX treatment.119 Furthermore, several recent studies evaluated the effects of liposomal amphotericin B, AmBisome®, and showed promising results in the topical treatment of cutaneous leishmaniasis.120
Another interesting and promising application of liposomes is in the ocular drug delivery field. Among their many benefits in this field, liposomes can enhance drug levels in different ocular tissues by extending drug persistence on the eye's surface, improving corneal permeation, and managing drug release. Also, they do not pose any toxicity or irritation to the eyes. Dry eye, caused by inferior tear quality and quantity, is a chronic condition affecting many people. Several studies have been conducted to create artificial tears for treating dry eye disease using liposomes. Ectoin® Eye Spray – Colloidal was compared to liposomal eye spray Tears Again® in treating mild–moderate dry eye disease patients in a recently completed study. The study aimed to evaluate the efficiency and tolerability of both formulations, and the results have not yet been published (ClinicalTrials.gov-study: NCT03519815
121). In another phase II clinical trial, the safety and efficacy of liposomal latanoprost in treating glaucoma, an eye condition in which the optic nerve becomes damaged and contributes to ocular hypertension, was assessed, and the study results are yet to be published (ClinicalTrials.gov-study: NCT01987323
122).123
Table 6 illustrates the clinical trials made in different liposomes applications. Additionally, Table 7 illustrates some of the recent patents of liposomes in drug delivery.
Table 6 Clinical trials in different liposomes applications
Study number |
Title |
Phase |
Ref. |
NCT04791228 |
Thermodox and MR-HIFU for treatment of relapsed solid tumors |
Phase II |
93
|
NCT00964080 |
Study of MBP-426 in patients with second line gastric, gastroesophageal, or esophageal adenocarcinoma |
Phase II |
94
|
NCT05101187 |
Olorofim Aspergillus infection study |
Phase III |
98
|
NCT02499159 |
Pain management in response to Exparel vs. standard Bupivicaine |
Phase IV |
102
|
NCT05232851 |
A vaccine (PDS0101) alone or in combination with Pembrolizumab for the treatment of locally advanced Human Papillomavirus-associated Oropharynx cancer |
Phase I/II |
105
|
NCT04580771 |
A vaccine (PDS0101) and chemoradiation for the treatment of stage IB3-IVA cervical cancer, the IMMUNOCERV trial |
Phase IIA |
106
|
NCT 04368728 |
Study to describe the safety, tolerability, immunogenicity, and efficacy of RNA vaccine candidates against COVID-19 in healthy individuals |
Phase 3 |
114
|
NCT04470427 |
A study to evaluate efficacy, safety, and immunogenicity of mRNA-1273 vaccine in adults aged 18 years and older to prevent COVID-19 |
Phase 3 |
115
|
NCT03519815 |
Clinical study to evaluate the efficacy of Ectoin® containing eye spray for treatment of dry eye disease |
Not applicable |
121
|
NCT01987323 |
Safety and efficacy of liposomal Latanoprost in ocular hypertension |
Phase II |
122
|
Table 7 Some patents in liposomal drug delivery
Patent number |
Year |
Inventor |
Assignee |
Brief description |
Ref. |
11890352 |
2024 |
Kimberly A. Kelly, Siva Sai Krishna Dasa |
University of Virginia Patent Foundation |
Plectin-targeted liposomes/PARP inhibitor in the treatment of cancer |
124
|
US20190110989A1 |
2020 |
Ghaleb Husseini, Mohammad Al-Sayah, Amal Elsadig |
American University of Sharjah |
Ultrasound triggered drug release from immunoliposomes for breast cancer treatment |
125
|
US10213385B2 |
2019 |
Jun Yang, Stephen H. Wu, Cliff J. Herman |
Mallinckrodt LLC |
Administration of a therapeutic liposome with an active agent, followed by an attacking liposome that induces the agent's release from the therapeutic liposome |
126
|
US-9895313-B2 |
2016 |
Zhu De Min, Chen Guoqiang |
Cureport Inc |
A pharmaceutical composition containing two liposome types for delivering docetaxel and doxorubicin to treat lung, colon, breast, and liver cancers |
127
|
US20060002994A1 |
2006 |
James Thomas, Hung-Yin Lin, Natalia Rapoport |
University of Utah Research Foundation |
Liposomes modified with a surface-active dopant to enhance permeability when exposed to ultrasound |
128
|
6. Challenges and future prospective
Liposomes are increasingly being researched and used as drug carriers in drug delivery systems. Despite many benefits, such as reduced toxicity, extended circulation time, and improved pharmacokinetic properties of the encapsulated drug, liposomes face some pitfalls and challenges that hinder their clinical translation from bench to bedside. While the phospholipid bilayer of liposomes has the advantage of being similar to cells, it may sometimes undergo oxidation reactions or hydrolysis, two leading causes of chemical instability. Hydrolysis happens due to the cleavage of ester bonds that hold the lipid constituents together.129 Upon hydrolysis, the free fatty acids lower the pH in the surrounding medium and produce harmful substances to the human body. Antioxidants like vitamins C and E and flavonoids can be incorporated in liposome formulations to prevent oxidation. Cholesterol can be added to slow down the hydrolysis process, and the freeze-drying method can be used to prepare and preserve liposomes. Another factor influencing chemical stability is the zeta potential; synthesizing liposomes within the stable range by properly selecting lipids and optimizing the attachment of moieties to the surface of the liposomes reduces the possibility of aggregation.14
Moreover, the physical stability of liposomes is another critical limitation affecting their clinical translation. Physical stability highly depends on the liposome's lipid composition, size distribution, and storage conditions. Any defects in the liposome's structure lead to the leakage of the encapsulated drug and liposomes aggregation during storage. Therefore, using saturated phospholipids like hydrogenated phosphatidylcholine instead of unsaturated phospholipids enhances the physical stability of the lipid membrane. Additionally, using the freeze-drying method to preserve the liposomes could guarantee better and long-term physical stability.108
Despite the many benefits of PEGylation, especially in overcoming the immune response against liposomes, many studies discuss its serious challenges. In fact, PEGylated liposomes face difficulties in effectively interacting with target cells because of the steric hindrance caused by the PEG chain. Therefore, endocytosis, which is the primary pathway for the uptake of liposomes by cells, could be hindered. Moreover, when administering the PEGylated liposomes in multiple doses, antibodies are produced due to a phenomenon known as accelerated blood clearance (ABC), which affects the circulation time of liposomes in the body.129,130 Furthermore, researchers adopted several strategies to evade this phenomenon. For example, Saadati et al. examined how changing the dose interval of PEGylated nanoparticles would affect their pharmacokinetics. They found that increasing the dosing interval from 7 to 28 days reduced the ABC phenomenon.132 Furthermore, Ishida et al. examined the effect of the density of PEG chains. They found that very high or very low density of PEG on the liposome's surface could cause an immune response towards the liposomes.133 Additionally, reducing the length of PEG chains could also help in evading the ABC phenomenon.131
Furthermore, human cancers’ heterogeneous extracellular matrix (ECM) increases the interstitial fluid pressure, preventing liposome accumulation and extravasation, particularly in the tumor center. Additionally, the ECM density affects the efficiency of the EPR effect in tumor tissues. For example, pancreatic adenocarcinomas have a dense ECM, hindering the penetration of liposomes in tumor tissue.134 Nevertheless, enhancing the EPR effect can be achieved by increasing the vascular leakiness through hyperthermia, sonoporation, and radiotherapy or by tumor-specific vasodilation.131
Moreover, to overcome multi-drug resistance and achieve synergistic efficacy, co-delivery strategies in which multiple drugs are encapsulated simultaneously in the liposomes can be utilized.135
Nevertheless, animal models are crucial for assessing the efficiency of liposomes as anti-tumor drugs; however, their results do not always align with the clinical trials. Mouse solid tumor models usually overestimate the therapeutic effectiveness of ligand-targeted liposomes due to the exaggeration in the EPR effect due to the rapid tumor growth and the leaky blood vessels, especially in subcutaneous tumor models. Since the tumor cell's inoculation site influences the degree of the EPR effect, using orthotopic tumor models could be a promising method of assessing the effectiveness of the liposomal formulation. Additionally, intrarectal injection of cell lines showed more accuracy in evaluating colorectal cancer than other models. Mouse models also struggle to assess liposomal formulation's efficiency in metastatic and advanced cancer cases. For this reason, incorporating metastatic models, selecting proper animal models, and utilizing multiple models in preclinical studies might aid researchers in achieving more reliable results.131
Lastly, the existing liposome manufacturing methods pose a significant challenge in scaling up production due to their complexity and inefficiency. The most commonly used method, the ethanol injection method followed by extrusion, involves multiple steps requiring energy, time, and expertise, making large-scale production challenging. However, microfluidics and nanoprecipitation provide promising solutions by potentially eliminating the need for solvent removal and extrusion, streamlining the process, and enhancing the efficiency of liposome production on a commercial scale.136
Future research must also focus on integrating liposomes with personalized medicine to tailor drug delivery systems according to patient's disease conditions and genomic information.135
7. Conclusions
Liposomes, among other nanoparticles, represent an up-and-coming technology for revolutionizing drug delivery systems due to their biocompatibility, versatility, morphological similarity to the body cells, and increased pharmacokinetics and stability of the encapsulated drug. Since their first entry into the market in 1995, they have been continuously researched for various applications, including cancer treatment, vaccines, and pain management. Several liposomal formulations and drug-release triggering mechanisms have been developed since then, including thermosensitive, pH-sensitive, enzyme-sensitive, ultrasound-sensitive, light-sensitive, and redox-sensitive liposomes. This reflects the intensive and dedicated research to advance liposome technology. Despite the successful translation of many liposomes into clinical use, several limitations impede many others in different clinical phases from progressing into clinical use. Significant aspects like their chemical and physical stability, drug leakage, encapsulation efficiency, large-scale production methods, and model evaluation must be considered in future research to unlock the full potential of liposomes in drug delivery systems.
Author contributions
Reem M. Senjab wrote the review. Nour AlSawaftah, Waad H. Abuwatfa, and Ghaleb A. Husseini reviewed and approved the final version.
Data availability
There is no new data generated in this review. This is a review article with no new experiments.
Conflicts of interest
There are no conflicts to declare.
Acknowledgements
This research study was funded by the Dana Gas Endowed Chair for Chemical Engineering, the American University of Sharjah Faculty Research Grants (FRG20-L-E48, FRG22-C-E08), Sheikh Hamdan Award for Medical Sciences (MRG/18/2020), and Friends of Cancer Patients (FoCP).
References
- H. Nsairat, D. Khater, U. Sayed, F. Odeh, A. Al Bawab and W. Alshaer, Liposomes: structure, composition, types, and clinical applications, Heliyon, 2022, 8(5), e09394 CrossRef CAS PubMed.
- T. M. Allen and P. R. Cullis, Drug Delivery Systems: Entering the Mainstream, Science, 2004, 303(5665), 1818–1822 CrossRef CAS PubMed . Available from: https://www-science-org.aus.idm.oclc.org/doi/10.1126/science.1095833 [cited 2024 Feb 10].
- D. Khater, H. Nsairat, F. Odeh, M. Saleh, A. Jaber and W. Alshaer,
et al., Design, preparation, and characterization of effective dermal and transdermal lipid nanoparticles: A review, Cosmetics, 2021, 8(2), 39 CrossRef CAS.
- F. U. Din, W. Aman, I. Ullah, O. S. Qureshi, O. Mustapha and S. Shafique,
et al., Effective use of nanocarriers as drug delivery systems for the treatment of selected tumors, Int. J. Nanomed., 2017, 12, 7291 CrossRef PubMed . Available from: https://pmc/articles/PMC5634382/ [cited 2024 Mar 5].
- M. A. Elkhodiry, C. C. Momah, S. R. Suwaidi, D. Gadalla, A. M. Martins and R. F. Vitor,
et al., Synergistic Nanomedicine: Passive, Active, and Ultrasound-Triggered Drug Delivery in Cancer Treatment, J. Nanosci. Nanotechnol., 2016, 16(1), 1–18 CrossRef CAS PubMed.
- M. D. Fulton and W. Najahi-Missaoui, Liposomes in Cancer Therapy: How Did We Start and Where Are We Now, Int. J. Mol. Sci., 1960, 2023, 6615 Search PubMed.
-
C. I. Nkanga, A. M. Bapolisi, N. I. Okafor, R. W. M. Krause, C. I. Nkanga and A. M. Bapolisi, et al., General Perception of Liposomes: Formation, Manufacturing and Applications, in Liposomes - Advances and Perspectives, 2019; Available from: https://www.intechopen.com/chapters/66369 [cited 2024 Feb 17] Search PubMed.
- J. Li, X. Wang, T. Zhang, C. Wang, Z. Huang and X. Luo,
et al., A review on phospholipids and their main applications in drug delivery systems, Asian J. Pharm. Sci., 2015, 10(2), 81–98 CrossRef.
- U. Bulbake, S. Doppalapudi, N. Kommineni and W. Khan, Liposomal Formulations in Clinical Use: An Updated Review, Pharmaceutics, 2017, 9(2), 12 CrossRef PubMed . Available from: https://www.mdpi.com/1999-4923/9/2/12/htm [cited 2024 Mar 5].
- R. Mare, D. Paolino, C. Celia, R. Molinaro, M. Fresta and D. Cosco, Post-insertion parameters of PEG-derivatives in phosphocholine-liposomes, Int. J. Pharm., 2018, 552(1–2), 414–421 CrossRef CAS PubMed.
- MEMBRANE LIPIDS I and II: – ppt download. Available from: https://slideplayer.com/slide/3377402/ [cited 2024 Feb 17].
- General structure of phospholipids and common head groups. PLs contain… | Download Scientific Diagram. Available from: https://www.researchgate.net/figure/General-structure-of-phospholipids-and-common-head-groups-PLs-contain-two-fatty-acids_fig1_261605192 [cited 2024 Feb 17].
- Sphingomyelin (SM) – Lipoid. Available from: https://lipoid.com/en/products/natural-phospholipids/sphingomyelin/ [cited 2024 Feb 17].
- S. Wang, Y. Chen, J. Guo and Q. Huang, Liposomes for Tumor Targeted Therapy: A Review, Int. J. Mol. Sci., 2023, 24(3), 2643 CrossRef CAS PubMed.
- G. Liu, S. Hou, P. Tong and J. Li, Liposomes: Preparation, Characteristics, and Application Strategies in Analytical Chemistry, Crit. Rev. Anal. Chem., 2022, 52(2), 392–412 CrossRef CAS PubMed . Available from: https://www-tandfonline-com.aus.idm.oclc.org/doi/abs/10.1080/10408347.2020.1805293 [cited 2024 Feb 10].
- G. Zhang and J. Sun, Lipid in chips: A brief review of liposomes formation by microfluidics, Int. J. Nanomed., 2021, 16, 7391–7416 CrossRef CAS PubMed . Available from: https://www.tandfonline.com/action/journalInformation?journalCode=dijn20 [cited 2024 Feb 10].
- V. Filipe, A. Hawe and W. Jiskoot, Critical evaluation of nanoparticle tracking analysis (NTA) by NanoSight for the measurement of nanoparticles and protein aggregates, Pharm. Res., 2010, 27(5), 796–810 CrossRef CAS PubMed . Available from: https://link-springer-com.aus.idm.oclc.org/article/10.1007/s11095-010-0073-2 [cited 2024 Feb 10].
- X. Wang, Y. Song, Y. Su, Q. Tian, B. Li and J. Quan,
et al., Are PEGylated liposomes better than conventional liposomes? A special case for vincristine, Drug Delivery, 2016, 23(4), 1092–1100 CrossRef CAS PubMed . Available from: https://pubmed.ncbi.nlm.nih.gov/26024386/ [cited 2024 Feb 11].
- J. X. Zhang, K. Wang, Z. F. Mao, X. Fan, D. L. Jiang and M. Chen,
et al., Application of liposomes in drug development–focus on gastroenterological targets, Int. J. Nanomed., 2013, 8, 1325–1334 CrossRef PubMed . Available from: https://pubmed.ncbi.nlm.nih.gov/23630417/ [cited 2024 Feb 11].
- B. Čeh, M. Winterhalter, P. M. Frederik, J. J. Vallner and D. D. Lasic, Stealth® liposomes: from theory to product, Adv. Drug Delivery Rev., 1997, 24(2–3), 165–177 Search PubMed.
- J. Senior and G. Gregoriadis, Stability of small unilamellar liposomes in serum and clearance from the circulation: The effect of the phospholipid and cholesterol components, Life Sci., 1982, 30(24), 2123–2136 CrossRef CAS PubMed.
- Therapy of human ovarian carcinoma xenografts using doxorubicin encapsulated in sterically stabilized liposomes – PubMed. Available from: https://pubmed.ncbi.nlm.nih.gov/8252484/ [cited 2024 Feb 12].
- C. Rangger, A. Helbok, E. von Guggenberg, J. Sosabowski, T. Radolf and R. Prassl,
et al., Influence of PEGylation and RGD loading on the targeting properties of radiolabeled liposomal nanoparticles, Int. J. Nanomed., 2012, 7, 5889–5900 CrossRef CAS PubMed . Available from: https://www.tandfonline.com/action/journalInformation?journalCode=dijn20 [cited 2024 Feb 12].
- B. Čeh, M. Winterhalter, P. M. Frederik, J. J. Vallner and D. D. Lasic, Stealth® liposomes: from theory to product, Adv. Drug Delivery Rev., 1997, 24(2–3), 165–177 Search PubMed.
- K. He and M. Tang, Safety of novel liposomal drugs for cancer treatment: Advances and prospects, Chem.-Biol. Interact., 2018, 295, 13–19 CrossRef CAS PubMed.
- S. H. Jung, S. H. Jung, H. Seong, S. H. Cho, K. S. Jeong and B. C. Shin, Polyethylene glycol-complexed cationic liposome for enhanced cellular uptake and anticancer activity, Int. J. Pharm., 2009, 382(1–2), 254–261 CrossRef CAS PubMed.
- Y. Chi, X. Yin, K. Sun, S. Feng, J. Liu and D. Chen,
et al., Redox-sensitive and hyaluronic acid functionalized liposomes for cytoplasmic drug delivery to osteosarcoma in animal models, J. Controlled Release, 2017, 261, 113–125 CrossRef CAS PubMed.
- W. Zhao, S. Zhuang and X. R. Qi, Comparative study of the in vitro and in vivo characteristics of cationic and neutral liposomes, Int. J. Nanomed., 2011, 3087 CAS . Available from: https://www.tandfonline.com/action/journalInformation?journalCode=dijn20 [cited 2024 Feb 12].
- T. Ogiso, T. Yamaguchi, M. Iwaki, T. Tanino and Y. Miyake, Effect of Positively and Negatively Charged Liposomes on Skin Permeation of Drugs, J. Drug Targeting, 2001, 9(1), 49–59 CrossRef CAS PubMed . Available from: https://www.tandfonline.com/doi/abs/10.3109/10611860108995632 [cited 2024 Feb 12].
- C. Sun, Bubble Liposome: A Modern Theranostic Approach of New Drug Delivery, World J. Pharm. Pharm. Sci., 2017, 1290–1314 Search PubMed.
- K. Sakaguchi, N. Kudo, K. Yamamoto, R. Suzuki and K. Maruyama, Characterization of bubble liposomes by of ultrasound attenuation effects of shell materials, Proc. - IEEE Ultrason. Symp., 2008, 1675–1678 CAS.
- Debasis Bagchi by. M. Bagchi, H. Moriyama, F. Shahidi, K. Maruyama and R. Suzuki,
et al., Ultrasound-Mediated Delivery Systems: Using Nano/Microbubbles or Bubble Liposomes. Bio-Nanotechnology: A Revolution in Food, Biomed. Health Sci., 2013, 227–245 Search PubMed . Available from: https://onlinelibrary.wiley.com/doi/full/10.1002/9781118451915.ch12 [cited 2024 Feb 14].
- M. S. Khan, J. Hwang, K. Lee, Y. Choi, Y. Seo and H. Jeon,
et al., Anti-Tumor Drug-Loaded Oxygen Nanobubbles for the Degradation of HIF-1α and the Upregulation of Reactive Oxygen Species in Tumor Cells, Cancers, 2019, 11(10), 1464 CrossRef CAS PubMed . Available from: https://pmc/articles/PMC6826834/ [cited 2024 May 1].
-
C. Andreou, Y. Gregoriou, A. Ali and S. Pal, In vivo imaging with SERS nanoprobes, in SERS for Point-of-care and Clinical Applications, 2022, pp. 199–235 Search PubMed.
- T. O. B. Olusanya, R. R. H. Ahmad, D. M. Ibegbu, J. R. Smith and A. A. Elkordy, Liposomal Drug Delivery Systems and Anticancer Drugs, Molecules, 2018, 23(4), 907 CrossRef PubMed . Available from: https://www.mdpi.com/1420-3049/23/4/907/htm [cited 2024 Feb 14].
- K. Maruyama, O. Ishida, T. Takizawa and K. Moribe, Possibility of active targeting to tumor tissues with liposomes, Adv. Drug Delivery Rev., 1999, 40(1–2), 89–102 CrossRef CAS PubMed.
- N. Alsawaftah, W. G. Pitt and G. A. Husseini, Dual-Targeting and Stimuli-Triggered Liposomal Drug Delivery in Cancer Treatment, ACS Pharmacol. Transl. Sci., 2021, 4(3), 1028–1049 CrossRef CAS PubMed . Available from: https://pubs.acs.org/doi/abs/10.1021/acsptsci.1c00066 [cited 2024 May 2].
- F. Movahedi, R. G. Hu, D. L. Becker and C. Xu, Stimuli-responsive liposomes for the delivery of nucleic acid therapeutics, Nanomedicine, 2015, 11(6), 1575–1584 CrossRef CAS PubMed.
- Y. Lee and D. H. Thompson, Stimuli-Responsive Liposomes for Drug Delivery, Wiley Interdiscip. Rev.: Nanomed. Nanobiotechnol., 2017, 9(5), 10.1002/wnan.1450, DOI:10.1002/wnan.1450 . Available from: https://pmc/articles/PMC5557698/ [cited 2024 Feb 17].
- H. F. Abed, W. H. Abuwatfa and G. A. Husseini, Redox-Responsive Drug Delivery Systems: A Chemical Perspective, Nanomaterials, 2022, 12(18), 3183 CrossRef CAS PubMed . Available from: https://www.mdpi.com/2079-4991/12/18/3183/htm [cited 2024 Mar 31].
- E. Fleige, M. A. Quadir and R. Haag, Stimuli-responsive polymeric nanocarriers for the controlled transport of active compounds: Concepts and applications, Adv. Drug Delivery Rev., 2012, 64(9), 866–884 CrossRef CAS PubMed.
- S. Gai, G. Yang, P. Yang, F. He, J. Lin and D. Jin,
et al., Recent advances in functional nanomaterials for light–triggered cancer therapy, Nano Today, 2018, 19, 146–187 CrossRef CAS.
- A. Yavlovich, B. Smith, K. Gupta, R. Blumenthal and A. Puri, Light-sensitive lipid-based nanoparticles for drug delivery: design principles and future considerations for biological applications, Mol. Membr. Biol., 2010, 27(7), 364–381 CrossRef CAS PubMed . Available from: https://www.tandfonline.com/doi/abs/10.3109/09687688.2010.507788 [cited 2024 Feb 18].
- M. B. C. de Matos, N. Beztsinna, C. Heyder, M. H. A. M. Fens, E. Mastrobattista and R. M. Schiffelers,
et al., Thermosensitive liposomes for triggered release of cytotoxic proteins, Eur. J. Pharm. Biopharm., 2018, 132, 211–221 CrossRef CAS PubMed.
- T. Ta and T. M. Porter, Thermosensitive liposomes for localized delivery and triggered release of chemotherapy, J. Controlled Release, 2013, 169(1–2), 112–125 CrossRef CAS PubMed.
- L. Tavano and R. Muzzalupo, Multi-functional vesicles for cancer therapy: The ultimate magic bullet, Colloids Surf., B, 2016, 147, 161–171 CrossRef CAS PubMed.
- S. R. Sirsi and M. A. Borden, State-of-the-art materials for ultrasound-triggered drug delivery, Adv. Drug Delivery Rev., 2014, 72, 3–14 CrossRef CAS PubMed.
- M. Vila-Caballer, G. Codolo, F. Munari, A. Malfanti, M. Fassan and M. Rugge,
et al., A pH-sensitive stearoyl-PEG-poly(methacryloyl sulfadimethoxine)-decorated liposome system for protein delivery: An application for bladder cancer treatment, J. Controlled Release, 2016, 238, 31–42 CrossRef CAS PubMed.
- Z. Wang, L. Ling, Y. Du, C. Yao and X. Li, Reduction responsive liposomes
based on paclitaxel-ss-lysophospholipid with high drug loading for intracellular delivery, Int. J. Pharm., 2019, 564, 244–255 CrossRef CAS PubMed . Available from: https://pubmed.ncbi.nlm.nih.gov/31022499/ [cited 2024 May 2].
- H. Pourhassan, G. Clergeaud, A. E. Hansen, R. G. Østrem, F. P. Fliedner and F. Melander,
et al., Revisiting the use of sPLA2-sensitive liposomes in cancer therapy, J. Controlled Release, 2017, 261, 163–173 CrossRef CAS PubMed.
- M. M. Chen, Y. Y. Liu, G. H. Su, F. F. Song, Y. Liu and Q. Q. Zhang, NIR responsive liposomal system for rapid release of drugs in cancer therapy, Int. J. Nanomed., 2017, 12, 4225–4239 CrossRef CAS PubMed . Available from: https://www.dovepress.com/nir-responsive-liposomal-system-for-rapid-release-of-drugs-in-cancer-t-peer-reviewed-fulltext-article-IJN [cited 2024 May 3].
- M. Derieppe, J. M. Escoffre, B. Denis De Senneville, Q. Van Houtum, A. B. Van Rijbroek and K. Van Der Wurff-Jacobs,
et al., Assessment of Intratumoral Doxorubicin Penetration after Mild Hyperthermia-Mediated Release from Thermosensitive Liposomes, Contrast Media Mol. Imaging, 2019, 2019, 2645928 Search PubMed.
- Y. J. Lu, E. Y. Chuang, Y. H. Cheng, T. S. Anilkumar, H. A. Chen and J. P. Chen, Thermosensitive magnetic liposomes for alternating magnetic field-inducible drug delivery in dual targeted brain tumor chemotherapy, Chem. Eng. J., 2019, 373, 720–733 CrossRef CAS.
- Z. Deng, Y. Xiao, M. Pan, F. Li, W. Duan and L. Meng,
et al., Hyperthermia-triggered drug delivery from iRGD-modified temperature-sensitive liposomes enhances the anti-tumor efficacy using high intensity focused ultrasound, J. Controlled Release, 2016, 243, 333–341 CrossRef CAS PubMed . Available from: https://pubmed.ncbi.nlm.nih.gov/27984104/ [cited 2024 May 3].
- D. E. Large, R. G. Abdelmessih, E. A. Fink and D. T. Auguste, Liposome composition in drug delivery design, synthesis, characterization, and clinical application, Adv. Drug Delivery Rev., 2021, 176, 113851 CrossRef CAS PubMed.
- (PDF) Stealth liposomes: a review. Available from: https://www.researchgate.net/publication/266225913_Stealth_liposomes_a_review [cited 2024 Mar 19].
-
N. Q. Shi and X. R. Qi, Preparation of Drug Liposomes by Reverse-Phase Evaporation 3, 2011, DOI:10.1007/978-3-662-49320-5_3 [cited 2024 Mar 19].
- S. Vemuri and C. T. Rhodes, Preparation and characterization of liposomes as therapeutic delivery systems: a review, Pharm. Acta Helv., 1995, 70(2), 95–111 CrossRef CAS PubMed.
- A. Akbarzadeh, R. Rezaei-Sadabady, S. Davaran, S. W. Joo, N. Zarghami and Y. Hanifehpour,
et al., Liposome: Classification, preparation, and applications, Nanoscale Res. Lett., 2013, 8(1), 1–9 CrossRef PubMed . Available from: https://link.springer.com/article/10.1186/1556-276X-8-102 [cited 2024 Feb 10].
- H. Nsairat, W. Alshaer, F. Odeh, E. Esawi, D. Khater and A. Al Bawab,
et al., Recent advances in using liposomes for delivery of nucleic acid-based therapeutics, OpenNano, 2023, 11, 100132 CrossRef CAS.
- X. Wang, J. Liu, P. Wang, A. deMello, L. Feng and X. Zhu,
et al., Synthesis of Biomaterials Utilizing Microfluidic Technology, Genes, 2018, 9(6), 283 CrossRef PubMed . Available from: https://www.mdpi.com/2073-4425/9/6/283/htm [cited 2024 Mar 20].
- V. V. S. N. L. Andra, S. V. N. Pammi, L. V. K. P. Bhatraju and L. K. Ruddaraju, A Comprehensive Review on Novel Liposomal Methodologies, Commercial Formulations, Clinical Trials and Patents, Bionanoscience, 2022, 12(1), 274–291 CrossRef PubMed . Available from: https://link.springer.com/article/10.1007/s12668-022-00941-x [cited 2024 Mar 20].
- Liposomes: an overview of manufacturing techniques – PubMed. Available from: https://pubmed.ncbi.nlm.nih.gov/16341279/ [cited 2024 Mar 20].
- R. L. Shew and D. W. Deamer, A novel method for encapsulation of macromolecules in liposomes, Biochim. Biophys. Acta, Biomembr., 1985, 816(1), 1–8 CrossRef CAS PubMed.
- H. Hauser and N. Gains, Spontaneous vesiculation of phospholipids:
a simple and quick method of forming unilamellar vesicles, Proc. Natl. Acad. Sci. U. S. A., 1982, 79(6), 1683–1687 CrossRef CAS PubMed . Available from: https://www.pnas.org/doi/abs/10.1073/pnas.79.6.1683 [cited 2024 Mar 20].
- R. Genç and M. Ortiz, O'Sullivan CK. Curvature-tuned preparation of nanoliposomes, Langmuir, 2009, 25(21), 12604–12613 CrossRef PubMed . Available from: https://pubs.acs.org/doi/abs/10.1021/la901789h [cited 2024 Mar 20].
- B. William, P. Noémie, E. Brigitte and P. Géraldine, Supercritical fluid methods: An alternative to conventional methods to prepare liposomes, Chem. Eng. J., 2020, 383, 123106 CrossRef CAS.
- E. C. A. Van Winden, Freeze-Drying of Liposomes: Theory and Practice, Methods Enzymol., 2003, 367, 99–110 CAS.
- O. H. El-Nesr, S. A. Yahiya and O. N. El-Gazayerly, Effect of formulation design and freeze-drying on properties of fluconazole multilamellar liposomes, Saudi Pharm. J., 2010, 18(4), 217–224 CrossRef CAS PubMed.
- S. Pisani, D. Di Martino, S. Cerri, I. Genta, R. Dorati and G. Bertino,
et al., Investigation and Comparison of Active and Passive Encapsulation Methods for Loading Proteins into Liposomes, Int. J. Mol. Sci., 2023, 24(17), 13542 CrossRef CAS PubMed . Available from: https://www.mdpi.com/1422-0067/24/17/13542/htm [cited 2024 Mar 29].
- P. Liu, G. Chen and J. Zhang, A Review of Liposomes as a Drug Delivery System: Current Status of Approved Products, Regulatory Environments, and Future Perspectives, Molecules, 2022, 27(4), 1372 CrossRef CAS PubMed . Available from: https://pmc/articles/PMC8879473/ [cited 2024 Mar 29].
- A. Fritze, F. Hens, A. Kimpfler, R. Schubert and R. Peschka-Süss, Remote loading of doxorubicin into liposomes driven by a transmembrane phosphate gradient, Biochim. Biophys. Acta, Biomembr., 2006, 1758(10), 1633–1640 CrossRef CAS PubMed.
- E. V. Tazina, K. V. Kostin and N. A. Oborotova, Specific features of drug encapsulation in liposomes (A review), Pharm. Chem. J., 2011, 45(8), 481–490 CrossRef CAS . Available from: https://link.springer.com/article/10.1007/s11094-011-0661-4 [cited 2024 Mar 29].
- B. Maherani and O. Wattraint, Liposomal structure: A comparative study on light scattering and chromatography techniques, J. Dispersion Sci. Technol., 2017, 38(11), 1633–1639 CrossRef CAS . Available from: https://www.tandfonline.com/doi/abs/10.1080/01932691.2016.1269651 [cited 2024 Mar 31].
- S. Mahmood, U. K. Mandal, B. Chatterjee and M. Taher, Advanced characterizations of nanoparticles for drug delivery: Investigating their properties through the techniques used in their evaluations, Nanotechnol. Rev., 2017, 6(4), 355–372 CAS . Available from: https://www.degruyter.com/document/doi/10.1515/ntrev-2016-0050/html [cited 2024 Mar 31].
-
V. S. Kulkarni and C. Shaw, Microscopy Techniques, in Essential Chemistry for Formulators of Semisolid and Liquid Dosages, 2016, pp. 183–192 Search PubMed.
- S. Giordani, V. Marassi, A. Zattoni, B. Roda and P. Reschiglian, Liposomes characterization for market approval as pharmaceutical products: Analytical methods, guidelines and standardized protocols, J. Pharm. Biomed. Anal., 2023, 236, 115751 CrossRef CAS PubMed.
- A. L. Robson, P. C. Dastoor, J. Flynn, W. Palmer, A. Martin and D. W. Smith,
et al., Advantages and limitations of current imaging techniques for characterizing liposome morphology, Front. Pharmacol., 2018, 9, 328115 Search PubMed . Available from: https://www.frontiersin.org [cited 2024 Mar 31].
- J. Bian, J. Girotti, Y. Fan, E. S. Levy, N. Zang and V. Sethuraman,
et al., Fast and versatile analysis of liposome encapsulation efficiency by nanoParticle exclusion chromatography, J. Chromatogr. A, 2022, 1662, 462688 CrossRef CAS PubMed.
- S. J. Wallace, J. Li, R. L. Nation and B. J. Boyd, Drug release from nanomedicines: Selection of appropriate encapsulation and release methodology, Drug Delivery Transl. Res., 2012, 2(4), 284 CrossRef CAS PubMed . Available from: https://pmc/articles/PMC3482165/ [cited 2024 May 2].
- S. Mourdikoudis, R. M. Pallares and N. T. K. Thanh, Characterization techniques for nanoparticles: comparison and complementarity upon studying nanoparticle properties, Nanoscale, 2018, 10(27), 12871–12934 RSC . Available from: https://pubs.rsc.org/en/content/articlehtml/2018/nr/c8nr02278j [cited 2024 Apr 1].
- C. S. Velez-Saboyá, J. R. Guzmán-Sepúlveda and J. C. Ruiz-Suárez, Phase transitions of liposomes: when light meets heat, J. Phys.: Condens. Matter, 2022, 34(12), 124002 CrossRef PubMed . Available from: https://iopscience.iop.org/article/10.1088/1361-<?pdb_no 648X?>648X<?pdb END?>/ac45b7 [cited 2024 Apr 1].
- M. Kanásová and K. Nesměrák, Systematic review of liposomes’ characterization methods, Monatsh. Chem., 2017, 148(9), 1581–1593 CrossRef . Available from: https://link.springer.com/article/10.1007/s00706-017-1994-9 [cited 2024 Apr 1].
- R. L. Siegel, A. N. Giaquinto, J. Ahmedin and R. L. Siegel, Cancer statistics, 2024, CA Cancer J. Clin., 2024, 74(1), 12–49 CrossRef PubMed . Available from: https://onlinelibrary.wiley.com/doi/full/10.3322/caac.21820 [cited 2024 May 1].
- What Causes Cancer? | Stanford Health Care. Available from: https://stanfordhealthcare.org/medical-conditions/cancer/cancer/cancer-causes.html [cited 2024 Feb 10].
- F. Rommasi and N. Esfandiari, Liposomal Nanomedicine: Applications for Drug Delivery in Cancer Therapy, Nanoscale Res. Lett., 2021, 16, 95, DOI:10.1186/s11671-021-03553-8 . Available from.
- M. Sarbaz, F. Manouchehri Monazah, S. Eslami, K. Kimiafar and S. F. Mousavi Baigi, Effect of mobile health interventions for side effects management in patients undergoing chemotherapy: A systematic review, Health Policy Technol., 2022, 11(4), 100680 CrossRef.
- C. K. Sahoo, N. K. Sahoo and M. Sahu, Liposomes for the treatment of prostate cancer therapy: A review, Cancer Treat. Res. Commun., 2024, 39, 100792 CrossRef PubMed . Available from: https://linkinghub.elsevier.com/retrieve/pii/S2468294224000042 [cited 2024 Feb 23].
- M. Kapoor, S. L. Lee and K. M. Tyner, Liposomal Drug Product Development and Quality: Current US Experience and Perspective, AAPS J., 2017, 19(3), 632–641 CrossRef CAS PubMed . Available from: https://link-springer-com.aus.idm.oclc.org/article/10.1208/s12248-017-0049-9 [cited 2024 Feb 23].
- PEGylated Liposomes in the Clinic and Clinical Trials | Biopharma PEG. Available from: https://www.biochempeg.com/article/320.html [cited 2024 Feb 23].
- H. Chou, H. Lin and J. M. Liu, A tale of the two PEGylated liposomal doxorubicins, OncoTargets Ther., 2015, 8, 1719 Search PubMed . Available from: https://pmc/articles/PMC4508070/ [cited 2024 Feb 23].
- A. C. Krauss, X. Gao, L. Li, M. L. Manning, P. Patel and W. Fu,
et al., FDA approval summary: (daunorubicin and cytarabine) liposome for injection for the treatment of adults with high-risk acute myeloid leukemia, Clin. Cancer Res., 2019, 25(9), 2685–2690 CrossRef CAS PubMed . Available from: https://clincancerres/article/25/9/2685/82519/FDA-Approval-Summary-Daunorubicin-and-Cytarabine [cited 2024 Feb 23].
- A Pilot Study of Thermodox and MR-HIFU for Treatment of Relapsed Solid Tumors – Full Text View – ClinicalTrials.gov. Available from: https://classic.clinicaltrials.gov/ct2/show/NCT04791228 [cited 2024 Apr 2].
- Study Details | Study of MBP-426 in Patients With Second Line Gastric, Gastroesophageal, or Esophageal Adenocarcinoma | ClinicalTrials.gov. Available from: https://clinicaltrials.gov/study/NCT00964080 [cited 2024 Apr 2].
- Y. He, W. Zhang, Q. Xiao, L. Fan, D. Huang and W. Chen,
et al., Liposomes and liposome-like nanoparticles: From anti-fungal infection to the COVID-19 pandemic treatment, Asian J. Pharm. Sci., 2022, 17(6), 817–837 CrossRef CAS PubMed.
- A. N. Plotnick, Lipid-based formulations of amphotericin B, J. Am. Vet. Med. Assoc., 2000, 216(6), 838–841 CAS . Available from: https://avmajournals.avma.org/view/journals/javma/216/6/javma.2000.216.838.xml [cited 2024 Feb 23].
- J. Adler-Moore and R. T. Proffitt, AmBisome: liposomal formulation, structure, mechanism of action and pre-clinical experience, J. Antimicrob. Chemother., 2002, 49(suppl_1), 21–30 CrossRef CAS PubMed . Available from: https:///10.1093/jac/49.suppl_1.21 [cited 2024 Feb 23].
- Study Details | Olorofim Aspergillus Infection Study | ClinicalTrials.gov. Available from: https://clinicaltrials.gov/study/NCT05101187?cond=Fungal%20Infection&term=Fungal%20Infection&intr=Liposomes&rank=17 [cited 2024 Apr 2].
- P. Minodier, K. Retornaz, A. Horelt and J. M. Garnier, Liposomal amphotericin B in the treatment of visceral leishmaniasis in immunocompetent patients, Fundam. Clin. Pharmacol., 2003, 17(2), 183–188 CrossRef CAS PubMed . Available from: https://onlinelibrary.wiley.com/doi/full/10.1046/j.1472-8206.2003.00168.x [cited 2024 Feb 23].
- M. S. Angst and D. R. Drover, Pharmacology of drugs formulated with DepoFoamTM: A sustained release drug delivery system for parenteral administration using multivesicular liposome technology, Clin. Pharmacokinet., 2006, 45(12), 1153–1176 CrossRef CAS PubMed . Available from: https://link.springer.com/article/10.2165/00003088-200645120-00002 [cited 2024 Feb 23].
- Liposomal Bupivacaine: A New Option for Postoperative Pain. Available from: https://www.uspharmacist.com/article/liposomal-bupivacaine-a-new-option-for-postoperative-pain [cited 2024 Feb 23].
- Study Details | Pain Management in Response to Exparel vs. Standard Bupivicaine | ClinicalTrials.gov. Available from: https://clinicaltrials.gov/study/NCT02499159?cond=Pain%20Management&term=Pain%20Management&intr=Liposomes&rank=16 [cited 2024 Apr 2].
- N. Filipczak, J. Pan, S. S. K. Yalamarty and V. P. Torchilin, Recent advancements in liposome technology, Adv. Drug Delivery Rev., 2020, 156, 4–22 CrossRef CAS PubMed.
-
M. Rahman, S. Beg, A. Verma, F. Anwar, A. Samad and V. Kumar, Liposomal-Based Therapeutic Carriers for Vaccine and Gene Delivery, in Nanotechnology-Based Approaches for Targeting and Delivery of Drugs and Genes, 2017, pp. 151–166 Search PubMed.
- Study Details | A Vaccine (PDS0101) Alone or in Combination With Pembrolizumab for the Treatment of Locally Advanced Human Papillomavirus-Associated Oropharynx Cancer | ClinicalTrials.gov. Available from: https://www.clinicaltrials.gov/study/NCT05232851 [cited 2024 Feb 25].
- Study Details | A Vaccine (PDS0101) and Chemoradiation for the Treatment of Stage IB3-IVA Cervical Cancer, the IMMUNOCERV Trial | ClinicalTrials.gov. Available from: https://clinicaltrials.gov/study/NCT04580771 [cited 2024 Feb 25].
- Hepatitis A, Available from: https://www.who.int/news-room/fact-sheets/detail/hepatitis-a [cited 2024 Feb 25].
- P. A. Bovier, J. Bock, L. Loutan, T. Farinelli, R. Glueck and C. Herzog, Long-term immunogenicity of an inactivated virosome hepatitis A vaccine, J. Med. Virol., 2002, 68(4), 489–493 CrossRef CAS PubMed . Available from: https://pubmed.ncbi.nlm.nih.gov/12376955/ [cited 2024 Feb 25].
- WHO EMRO | Influenza vaccines | Influenza | Health topics. Available from: https://www.emro.who.int/health-topics/influenza/influenza-vaccines.html [cited 2024 Mar 5].
- R. Glück and I. C. Metcalfe, New technology platforms in the development of vaccines for the future, Vaccine, 2002, 20(SUPPL. 5), B10–B16 CrossRef PubMed.
- U. B. Schaad, U. Bühlmann, R. Burger, A. Ruedeberg, A. Wilder-Smith and M. Rutishauser,
et al., Comparison of immunogenicity and safety of a virosome influenza vaccine with those of a subunit influenza vaccine in pediatric patients with cystic fibrosis, Antimicrob. Agents Chemother., 2000, 44(5), 1163–1167 CrossRef CAS PubMed . Available from: https://journals.asm.org/doi/10.1128/aac.44.5.1163-1167.2000 [cited 2024 Mar 5].
- R. Tenchov, R. Bird, A. E. Curtze and Q. Zhou, Lipid Nanoparticles from Liposomes to mRNA Vaccine Delivery, a Landscape of Research Diversity and Advancement, ACS Nano, 2021, 15(11), 16982–17015 CrossRef CAS PubMed . Available from: https://pubs.acs.org/doi/full/10.1021/acsnano.1c04996 [cited 2024 Mar 6].
- G. Gregoriadis, Liposomes and mRNA: Two technologies together create a COVID-19 vaccine, Med. Drug Discovery, 2021, 12, 100104 CrossRef CAS.
- Study Details | Study to Describe the Safety, Tolerability, Immunogenicity, and Efficacy of RNA Vaccine Candidates Against COVID-19 in Healthy Individuals | ClinicalTrials.gov. Available from: https://clinicaltrials.gov/study/NCT04368728 [cited 2024 Mar 6].
- Study Details | A Study to Evaluate Efficacy, Safety, and Immunogenicity of mRNA-1273 Vaccine in Adults Aged 18 Years and Older to Prevent COVID-19 | ClinicalTrials.gov. Available from: https://clinicaltrials.gov/study/NCT04470427 [cited 2024 Mar 6].
- F. Juhairiyah and E. C. M. de Lange, Understanding Drug Delivery to the Brain Using Liposome-Based Strategies: Studies that Provide Mechanistic Insights Are Essential, AAPS J., 2021, 23(6), 114 CrossRef CAS PubMed . Available from: https://pmc/articles/PMC8553706/ [cited 2024 Sep 8].
- D. B. Vieira and L. F. Gamarra, Getting into the brain: liposome-based strategies for effective drug delivery across the blood–brain barrier, Int. J. Nanomed., 2016, 11, 5381 CrossRef CAS PubMed . Available from: https://pmc/articles/PMC5077137/ [cited 2024 Sep 8].
- M. Rahman, K. Alam, S. Beg, F. Anwar and V. Kumar, Liposomes as topical drug delivery systems: State of the arts, Biomed. Appl. Nanopart., 2019, 149–161 CAS.
- M. F. M. Ali, M. Salah, M. Rafea and N. Saleh, Liposomal methotrexate hydrogel for treatment of localized psoriasis: preparation, characterization and laser targeting, Med. Sci. Monit., 2008, 14(12), PI66–PI74 Search PubMed . Available from: https://europepmc.org/article/med/19043379 [cited 2024 Sep 8].
- F. Frézard, M. M. G. Aguiar, L. A. M. Ferreira, G. S. Ramos, T. T. Santos and G. S. M. Borges,
et al., Liposomal Amphotericin B for Treatment of Leishmaniasis: From the Identification of Critical Physicochemical Attributes to the Design of Effective Topical and Oral Formulations, Pharmaceutics, 2023, 15(1), 99 CrossRef PubMed . Available from: https://www.mdpi.com/1999-4923/15/1/99/htm [cited 2024 Sep 8].
- Study Details | Clinical Study to Evaluate the Efficacy of Ectoin® Containing Eye Spray for Treatment of Dry Eye Disease | ClinicalTrials.gov. Available from: https://clinicaltrials.gov/study/NCT03519815 [cited 2024 Sep 8].
- Study Details | Safety and Efficacy of Liposomal Latanoprost in Ocular Hypertension | ClinicalTrials.gov. Available from: https://clinicaltrials.gov/study/NCT01987323 [cited 2024 Sep 8].
- R. Suri, S. Beg and K. Kohli, Target strategies for drug delivery bypassing ocular barriers, J. Drug Delivery Sci. Technol., 2020, 55, 101389 CrossRef CAS.
- US Patent for Plectin-targeted liposomes/PARP inhibitor in the treatment of cancer Patent (Patent # 11
890
352 issued February 6, 2024) – Justia Patents Search. Available from: https://patents.justia.com/patent/11890352 [cited 2024 Sep 14].
- American university of sharjahPatents | PatentGuru. Available from: https://www.patentguru.com/assignee/american-university-of-sharjah [cited 2024 Sep 14].
- US10213385B2 – Combinational liposome compositions for cancer therapy – Google Patents. Available from: https://patents.google.com/patent/US10213385B2/en [cited 2024 Sep 14].
- US-9895313-B2 – Combination liposomal pharmaceutical formulations | Unified Patents. Available from: https://portal.unifiedpatents.com/patents/patent/US-9895313-B2 [cited 2024 Sep 14].
- US20210113465A1 – Systems and methods for targeted breast cancer therapies – Google Patents. Available from: https://patents.google.com/patent/US20210113465A1/en [cited 2024 Sep 14].
- M. Anderson and A. Omri, The Effect of Different Lipid Components on the In Vitro Stability and Release Kinetics of Liposome Formulations, Drug Delivery, 2004, 11(1), 33–39 CrossRef CAS PubMed . Available from: https://www.tandfonline.com/doi/abs/10.1080/10717540490265243 [cited 2024 Apr 1].
- D. Zhang, H. Xu, M. N. Hu and Y. H. Deng, “PEG dilemma” for liposomes and its solving approaches, Yaoxue Xuebao, 2015, 50(3), 252–260 CAS . Available from: https://europepmc.org/article/med/26118102 [cited 2024 Sep 18].
- S. A. Moosavian, V. Bianconi, M. Pirro and A. Sahebkar, Challenges and pitfalls in the development of liposomal delivery systems for cancer therapy, Semin. Cancer Biol., 2021, 69, 337–348 CrossRef CAS PubMed.
- R. Saadati, S. Dadashzadeh, Z. Abbasian and H. Soleimanjahi, Accelerated blood clearance of PEGylated PLGA nanoparticles following repeated injections: Effects of polymer dose, PEG coating, and encapsulated anticancer drug, Pharm. Res., 2013, 30(4), 985–995 CrossRef CAS PubMed . Available from: https://link.springer.com/article/10.1007/s11095-012-0934-y [cited 2024 Sep 16].
- T. Ishida, M. Harada, Y. W. Xin, M. Ichihara, K. Irimura and H. Kiwada, Accelerated blood clearance of PEGylated liposomes following preceding liposome injection: Effects of lipid dose and PEG surface-density and chain length of the first-dose liposomes, J. Controlled Release, 2005, 105(3), 305–317 CrossRef CAS PubMed.
- Interstitial Pressure Gradients in Tissue-isolated and Subcutaneous Tumors: Implications for Therapy1 | Cancer Research | American Association for Cancer Research. Available from: https://aacrjournals.org/cancerres/article/50/15/4478/495616/Interstitial-Pressure-Gradients-in-Tissue-isolated [cited 2024 Sep 16].
- J. Chen, S. Hu, M. Sun, J. Shi, H. Zhang and H. Yu,
et al., Recent advances and clinical translation of liposomal delivery systems in cancer therapy, Eur. J. Pharm. Sci., 2024, 193, 106688 CrossRef CAS PubMed.
- S. Shah, V. Dhawan, R. Holm, M. S. Nagarsenker and Y. Perrie, Liposomes: Advancements and innovation in the manufacturing process, Adv. Drug Delivery Rev., 2020, 154–155, 102–122 CrossRef CAS PubMed.
|
This journal is © The Royal Society of Chemistry 2024 |
Click here to see how this site uses Cookies. View our privacy policy here.