DOI:
10.1039/D3RA07170G
(Paper)
RSC Adv., 2023,
13, 35621-35628
Effect of nano-Al2O3 and multi-walled carbon nanotubes on the anaerobic mono-digestion of sludge and the co-digestion of tobacco waste and sludge
Received
21st October 2023
, Accepted 1st December 2023
First published on 6th December 2023
Abstract
Anaerobic digestion can help mitigate tobacco waste (TW) pollution. Both the mono-digestion of sludge and the co-digestion of TW and sludge were considered in this study. Additionally, the effects of nano-Al2O3 and multi-walled carbon nanotubes (MWCNTs) on these two digestion systems were investigated through a 35 day digestion experiment. The microbial communities in the control reactors and the nano-Al2O3 reactors were also examined. Kinetic analysis revealed that the Rm values for the mono- and co-digestion nano-Al2O3 reactors increased by 8.88% and 13.5% compared with that of the MWCNTs reactor, respectively. Furthermore, the co-digestion system exhibited a 34.8% higher Rm than the mono-digestion system when nano-Al2O3 was added to both systems. Nano-Al2O3 was found to shorten the lag phase, while MWCNTs prolonged the lag phase time. Furthermore, 16S RNA amplicon sequencing results indicated that microbial species such as Methanobacterium sp., Hydrogenispora sp., Lutispora sp., and Ruminiclostridium sp. were more abundant in the nano-Al2O3 reactor. These results demonstrated that biogas production in co-digestion systems was improved. Moreover, nano-Al2O3 addition enhanced biogas production.
1 Introduction
Tobacco, as an important non-edible economic crop, and has been widely cultivated in many countries.1,2 A lot of tobacco waste (TW) is produced every year.3 TW is harmful to the environment, and it is usually incinerated, pyrolyzed, composted, or sent to landfills.4,5
Anaerobic digestion (AD) is the most effective renewable and sustainable method to convert waste into biomass energy. There are several advantages of AD: (1) it is characterized by a high organic loading rate, high treatment efficiency, and low sludge growth rate; (2) it can convert organic matter in waste into biogas; and (3) biogas slurry and residues from AD are valuable fertilizers in agriculture.6–9 Owing to these benefits, some researchers have focused on AD. In recent years, the conversion of TW into biomass energy through AD has been studied. Liu et al. obtained the maximum methane yield of 0.163 m3 CH4 per kg VS from co-digestion of tobacco stalks, wheat stalks and pig manure.9 González-González et al. obtained a methane yield of 53.84 ± 14.48 Nm3 CH4 per t from fresh tobacco under mesophilic conditions with a degradation period of 16 days.10,11 Ye et al. studied the cellulose content of TW, and the results showed that TW was suitable for AD owing to its lower cellulose content.12
However, TW is characterized by a typical lignocellulosic structure and lower biodegradability during the AD process.9 Moreover, AD could be inhibited because TW also has a higher alkaloid nicotine content. For example, the effect of nicotine on AD was investigated by Wang et al.13 They found that methane production decreased when nicotine content was added to the systems.13 According to a previous study, nicotine mainly affected microbial growth. To improve methane production and enhance microbial activity, some researchers have added additives, particularly nanomaterials additives, to the AD systems for TW. He et al. added biochar to the AD of flue-cured TW and studied its effects. Biochar enhanced methane production and shortened the lag period.14 This finding suggests that biochar can promote direct interspecific electron transfer.15–21 This interesting discovery provides an important reference for the AD process of TW. However, no studies have focused on the effects of other nanoporous materials, such as nano-Al2O3 or multi-walled carbon nanotubes (MWCNTs), on the co-digestion of TW and sludge.
Therefore, in this study, the changes in biogas production and microbial community from air-cured tobacco leaf waste and sludge using a dry AD system were investigated. To improve biogas production, the effects of nano-Al2O3 and MWCNT addition on AD performance were studied. The purposes of this study were as follows: (a) to compare biogas production, coenzyme F420 activities, chemical oxygen demand (COD) concentrations, and volatile fatty acid (VFA) concentrations during the dry AD process, and to explain the effects of two nanomaterials in mono-digestion and co-digestion systems; (b) to investigate the microbial community structure when nano-Al2O3 was added to the mono-digestion system and the co-digestion system.
2 Materials and methods
2.1 Materials
The TW used in the experiment was obtained from Yunnan Agricultural University, Yunnan Province. The samples were cut into small pieces (1–2 cm). The dehydrated sludge was collected from the Fifth Water Purification Plant in Kunming, Yunnan Province, and it appeared brown in color. The total solid (TS) content and the volatile solid (VS) content of tobacco were tested (Table 1). The C/N ratio of tobacco is presented in Table 2. The C/N ratio of TW was relatively low, and this ratio plays an important role in the AD process.22 According to the literature, a low C/N ratio can restrict VFA generation.25 C/N ratios between 20% and 30% are considered appropriate.23
Table 1 TS and VS of waste tobacco and sludge
Raw materials |
TS (%) |
VS (%) |
Tobacco waste |
90.17 ± 0.25 |
77.0 ± 2.59 |
Sludge |
44.07 ± 7.51 |
77.87 ± 3.45 |
Table 2 Elemental content analysis of TW
Raw materials |
Element content |
C (%) |
N (%) |
C/N (%) |
Tobacco waste |
37.20 ± 0.092 |
3.30 ± 0.021 |
11.26 ± 0.05 |
Sludge |
36.51 ± 0.08 |
0.74 ± 0.01 |
49.33 ± 0.045 |
2.2 Experimental setup
The experimental setup and the synthesis of nano-Al2O3 were based on our previous research.26 We set up six dry AD reactors: three reactors with sludge (the control [C], 300 mg per kg Al2O3 [CA], 300 mg per kg MWCNTs [CC]) and three reactors with TW and sludge (the control [CT], 300 mg per kg Al2O3 [CTA], 300 mg per kg MWCNTs [CTC]). All experiments were conducted at (35 ± 1) °C for 35 days (Table 3).
Table 3 Design of experiments in dry AD systems
Reactors |
Tobacco waste (g) |
Sludge (g) |
Water (g) |
Amount of additives (mg kg−1) |
C |
0 |
20 |
200 |
0 |
CA |
30 |
20 |
200 |
300 |
CC |
30 |
20 |
200 |
300 |
CT |
0 |
20 |
200 |
0 |
CTA |
30 |
20 |
200 |
300 |
CTC |
30 |
20 |
200 |
300 |
2.3 Analytical methods
The pH was measured using a pH meter (OHAUS, ST2100C), and the COD values were determined with a water quality rapid tester (5B-6CU8.3). TS and VS were measured following standard methods.25 The analytical method for F420 has been described in the literature.26 Total carbon and total nitrogen were measured using an elemental analysis instrument (VARIO EL 3, Germany). VFAs were tested using standard methods.27,28
2.4 Kinetic analysis
The modified Gompertz was used to fit the measured biogas production. The modified Gompertz was as follow:29,30
P = Pm exp(−exp(Rme/Pm(λ − t) + 1)) |
where “P” is the cumulative biogas production at time, “Pm” is the maximum cumulative biogas production. “Rm” is the maximum biogas rate (mL d−1 g−1), “e” is Euler's number (2.71828), “λ”is the lag phase time (d), and t is infinite digestion time (t).
2.5 Microbial community analysis
The test steps were as follows: first, DNA was extracted from 12 samples using the MIO-BIO Power Soil DNA Isolation Kit. Second, polymerase chain reaction (PCR) primers were designed, and the product was recovered. The V4–V5 variable region of the bacterial 16S rRNA gene was amplified using primers 515F (5′-GTGCCAGCMGCCGCGGTAA-3′) and 926R (5′-CCGTCAATTCMTTTGAGTTT-3′). The archaeal 16S rRNA gene was amplified using primers Arch519F (5′-CAGCCGCCGCGGTAA-3′) and Arch915R (5′-GTGCTCCCCCGCCAATTCCT-3′). The 16S V4–V5 PCR system was as follows: 5× PCR buffer 10 μL, dNTP 1 μL, DNA polymerase 1 μL, primer (F/R) 1 μL, template DNA 5–50 ng. The PCR conditions were as follows: 2 min at 94 °C, followed by 22 cycles of 30 s at 94 °C, 30 s at 55 °C, and 30 s at 72 °C, followed by a 5 min final extension at 72 °C. The archaeal PCR system was the same as the 16S V4–V5 PCR system. The PCR conditions were as follows: 2 min at 94 °C, followed by 27 cycles of 30 s at 94 °C, 30 s at 55 °C, and 30 s at 72 °C, followed by a 5 min final extension at 72 °C. Finally, the PCR results were analyzed through high-throughput sequencing using the NGS Illumina platform.
3 Results and discussion
3.1 Effect of the two nanomaterials on AD
3.1.1 AD performance of the single sludge reactors. The daily biogas production and the cumulative biogas production in three reactors were shown in Fig. 1. pH is an important factor affecting acid production and in retaining methanogenesis activity. The pH values in all systems remained in the range of 6.5–7.5 throughout the entire dry AD process. The daily biogas production and the cumulative biogas production in the CA reactor were noticeably higher than those in the other reactors (C and CC reactors). This illustrated that nano-Al2O3 can improve the biogas production of active sludge, while biogas production was suppressed with the addition of MWCNTs.
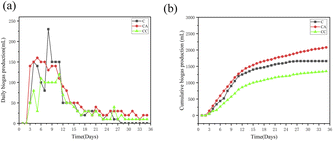 |
| Fig. 1 Effect of daily biogas yield (a) and cumulative biogas production (b) in single sludge reactors (C: control reactor; CA: nano-Al2O3 reactor; CC: MWCNTs reactor). | |
3.1.2 Performance of co-digestion reactors. As shown in Fig. 2, the daily biogas production and the cumulative biogas production in the co-digestion reactors were higher than those in the single sludge reactors. The largest daily biogas production was 760.00 mL when nano-Al2O3 was added. Moreover, the biogas production reached its peak on day 2. After day 15 and day 24, another small peak occurred. The largest daily biogas production for the second peak also came from the nano-Al2O3 reactor (CTA, 180 mL). From day 24, a third peak appeared. The daily biogas production in the CTA reactor was lower than that in the CT and CTC reactors. The effect of nano-Al2O3 on methanogenic activity possibly became smaller in the later stages of the AD process. The cumulative biogas production in the CTA reactor (7310 mL) was higher than that in the CTC reactor (4890 mL). The results illustrate that nano-Al2O3 can improve biogas production in TW and sludge co-digestion systems. As in previous research, nano-Al2O3 has no toxic effects on the methanogenic activity and can provide a good carrier for the AD process.24,31,32
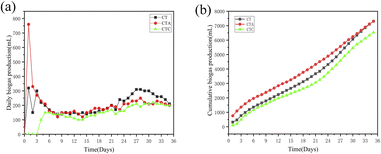 |
| Fig. 2 Effect of daily biogas yield (a) and cumulative biogas production (b) in co-digestion sludge reactors (CT: control reactor; CTA: nano-Al2O3 reactor; CTC: MWCNTs reactor). | |
As discussed above, the cumulative biogas production in co-digestion systems was higher than that in mono-digestion systems. Nano-Al2O3 enhanced biogas production, while MWCNTs inhibited it.
3.1.3 Coenzyme F420. Coenzyme F420, also known as 8-hydroxy-5-deazaflavin, is a low-potential electron carrier and an important enzyme found in methanogens.33 It can be used to reflect methanogenic activity.34–36 Fig. 3 illustrates the variations in coenzyme F420 activities. In mono-digestion systems, the coenzyme F420 activity increased during the first 15 days in the group with nano-Al2O3, while it decreased in the group with MWCNTs. This result shows that the addition of nano-Al2O3 improved the coenzyme F420 activity at the start of the experiment. In the co-digestion systems, no significant difference in coenzyme F420 levels existed between the groups with nano-Al2O3 and MWCNTs throughout the entire AD process. In the nano-Al2O3-containing group, the coenzyme F420 activity increased during the first 18 days, while in the MWCNT-added group, it initially increased and then decreased after day 9.
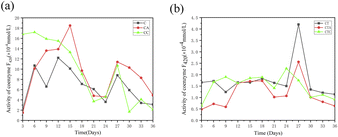 |
| Fig. 3 Variation in coenzyme F420 activity in single sludge systems (a) (C: control reactor; CA: nano-Al2O3 reactor; CC: MWCNTs reactor) and co-digestion systems (b) (CT: control reactor; CTA: nano-Al2O3 reactor; CTC: MWCNTs reactor). | |
3.1.4 Variations in COD concentration during the dry AD process. The variations in COD concentration are shown in Fig. 4. In contrast to the results of previous research, the COD concentration fluctuated significantly. In a previous study, during the AD process, the COD content was the outcome of the balance between hydrolysis, acetogenesis, and methanogenesis stages.37 The COD concentration have changed with different AD time. From the 3rd to the 9th day of the AD, the COD concentration in the CA and CTA reactors were lower, corresponding to the increase of cumulative and daily biogas yields (Fig. 4). This illustrated that organic components in two systems have been efficiently use to produce biogas.37
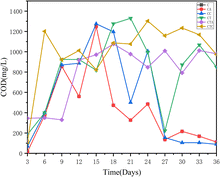 |
| Fig. 4 Changes in COD concentration in different reactors (in mono-digestion C: control reactor; CA: nano-Al2O3 reactor; CC: MWCNTs reactor; in co-digestion CT: control reactor; CTA: nano-Al2O3 reactor; CTC: MWCNTs reactor). | |
3.1.5 Responses of volatile fatty acids. Volatile fatty acids (VFAs) are intermediate products in the methanogenic process of anaerobic digestion and are a key factor in the smooth progress of AD. A sequence of complex biochemical reactions leads to the accumulation of fatty acids during the dry AD process. Fig. 5 illustrates the concentration changes of VFAs in different reactors. In both mono- and co-digestion systems, the VFA concentration in the C and CT reactors increased rapidly in the early stages of digestion, especially in the C reactors. The accumulation of VFAs can lead to the reduction of biogas production.38 In the middle stages of AD (from day 15 to day 21), the VFA concentration was significantly lower in the CTA reactor. Trophic methane bacteria acetate can join in the intermediate transfer of acetic acid. Acetic acid can reduce fatty acid accumulation and the high-activity H2 trophic methane bacteria can promote the degradation of VAFs.39 It was speculated that the methanogenic archaea in the nano-Al2O3 systems were mainly the acetate trophic and H2 trophic. The microbial analysis would confirm this suspicion.
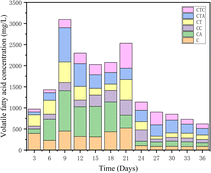 |
| Fig. 5 Changes in VFA concentrations during the AD process in different reactors. | |
3.2 Kinetic study of cumulative biogas production
The Gompertz model was selected to fit cumulative biogas production, and the parameters of the Gompertz model are presented in Table 4. As shown in Table 4, the fitting based on the Gompertz equation resulted in R2 values within the range of 0.95–0.99, indicating an effective fit of the Gompertz equation.
Table 4 Modified Gompertz model parameters of the cumulative biogas production in different reactors
Reactors |
Pa |
Rmb |
λc |
R2d |
Cumulative biogas production. Maximum biogas production. Lag phase time. Correlation coefficients. |
C |
1642.2840 ± 10.2682 |
149.084 ± 4.6235 |
3.0344 ± 0.1783 |
0.995 |
CA |
1973.6793 ± 22.7508 |
135.128 ± 5.9486 |
1.7794 ± 0.3252 |
0.988 |
CC |
1718.3792 ± 23.2571 |
122.628 ± 6.1098 |
3.6927 ± 0.3509 |
0.988 |
CT |
7310.0000 ± 0 |
218.739 ± 12.492 |
2.7250 ± 0.9558 |
0.951 |
CTA |
8230.6428 ± 1044.7869 |
207.141 ± 15.858 |
−0.7999 ± 1.2377 |
0.977 |
CTC |
4890.0000 ± 0 |
187.406 ± 7.790 |
7.7901 ± 0.6653 |
0.971 |
Thus, the AD process can be accurately simulated. The cumulative biogas production (P) is an important parameter used to evaluate biogas production. The P values for the six reactors were as follows: 1642.28401 ± 10.2682 mL (control, C, mono-digestion), 1973.6793 ± 22.7508 mL (nano-Al2O3, CA, mono-digestion), 1718.3792 ± 23.2571 mL (MWCNTs, CC, mono-digestion), 7310.0000 ± 0 mL (control, CT, co-digestion), 8230.6428 ± 1044.7869 mL (nano-Al2O3, CTA, co-digestion), and 4890.0000 ± 0 mL (MWCNTs, CTC, co-digestion). The order of the P values was as follows: CTA > CT > CTC > CA > CC > C. The Rm values for the mono- and co-digestion nano-Al2O3 reactors increased by 8.88% and 13.5% compared with that of the MWCNT reactor, respectively. Moreover, the Rm values of the co-digestion system increased by 34.8% compared with that of the mono-digestion system when nano-Al2O3 was added to both systems. This further illustrates that nano-Al2O3 had a positive impact on both co-digestion and mono-digestion systems. The lag phase time (λ) can also indicate biogas production. The value of λ was lower in the nano-Al2O3-added groups. The results indicated that the lag time was reduced and the reaction rate was improved when nano-Al2O3 was added to both systems.
3.3 Microbial community
3.3.1 Alpha-diversity and beta-diversity analysis. Considering the positive effects on the AD process, the microbial communities of the control reactors and the nano-Al2O3 reactors were selected for analysis. The four alpha-diversity indices (Chao1, ACE, Shannon, and Simpson) are presented in Table 5. In mono-digestion systems, the Chao1, ACE, and Shannon indices of the bacterial community of the CA reactor were higher than those of the CC reactor. This indicates that the bacterial community was richer in the CA group. For archaea, the order of the Chao1, ACE, and Shannon indices was the same as the order of the bacterial community; that is, CA exhibited higher bacterial and archaeal diversities. The Simpson index of the bacterial and archaeal communities in CA was lower, suggesting that the addition of nano-Al2O3 can increase the relative abundance of dominant flora. A comparison of the co-digestion system with the mono-digestion system revealed that the Chao1, ACE, and Shannon indices of the bacterial community in the CTA reactor were lower than those of the CT reactor. The order of the Chao1, ACE, and Shannon indices for archaea was the same as the order for the bacterial community. However, the Simpson index for archaea in CTA was lower. This indicates that nano-Al2O3 addition mainly influenced the succession of archaea in the co-digestion system.
Table 5 Indices of alpha diversity of archaeal and bacterial communities during the AD process
Reactors |
Chao1 |
ACE |
Shannon's |
Simpson's |
Bacterial |
Archaeal |
Bacterial |
Archaeal |
Bacterial |
Archaeal |
Bacterial |
Archaeal |
C |
2097.3920 |
64.7333 |
2127.0200 |
65.22086 |
5.5608 |
2.6572 |
0.01320 |
0.1109 |
CA |
2137.0080 |
87.8846 |
2159.3540 |
88.8773 |
5.7024 |
2.8564 |
0.01009 |
0.08937 |
CT |
2155.7780 |
93.7321 |
2175.6990 |
92.7387 |
5.6066 |
2.5088 |
0.01011 |
0.1966 |
CTA |
1838.9040 |
89.5214 |
1858.5450 |
91.1168 |
5.1785 |
2.3526 |
0.02278 |
0.1631 |
Beta diversity represents the differences in species composition between different reactors. As shown in Fig. 6, principal coordinate analysis (PCoA) illustrates the variations in microbial community composition. The contribution rates of principal components 1 and 2 were 15.51% and 14.38%, respectively. The first and second ordination axes could explain 79.24% and 15.37% of the archaeal community, respectively. Similarly, they could account for 40.6% and 10.69% of the bacterial community, respectively. As shown in Fig. 6, the C and CA reactors were notably separated from the CT and CTA reactors in the PCoA plot. These results indicate that the microbial community structure in mono-digestion and co-digestion systems differs significantly. Furthermore, the nano-Al2O3 reactors were distinctly separated from the control reactors in the bacterial PCoA plot (Fig. 6(b)), showing significant differences in bacterial community structure between the nano-Al2O3 reactors and the control reactors. Therefore, the addition of nano-Al2O3 had a pronounced effect on microbial diversity, richness, and composition during the AD process.
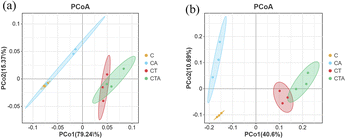 |
| Fig. 6 Archaeal (a) and bacterial (b) PCoA; differently colored points represent different groups (orange: C; blue: CA; red: CT; green: CTA). | |
3.3.2 Analysis of microbial species. For a more in-depth analysis of the effects of nano-Al2O3 addition on microbial community structure, archaeal and bacterial species at the phylum and genus levels were investigated. As shown in Fig. 7(a), there were three phyla: Euryarchaeota, Thaumarchaeota, and Crenarchaeota. Euryarchaeota was the dominant phylum, accounting for 99% of the relative abundance in all reactors. The relative abundance of Crenarchaeota was smaller. Studies have shown that Crenarchaeota played a key role in ammonia oxidation.40,41 The results indicated that the addition of nano-Al2O3 did not enhance the activity of Crenarchaeota. The bacterial community consisted of 17 phyla. The dominant phyla were Firmicutes, Bacteroidetes, Proteobacteria, Chloroflexi, and Synergistetes. Studies have demonstrated that these dominant phyla are primarily involved in the degradation of organic matter (such as proteins, lignocellulose, and fats).42–44 In mono-digestion systems, the nano-Al2O3 reactor exhibited a higher relative abundance of Bacteroidetes than the control reactor. Nano-Al2O3 addition may promote the hydrolysis of polysaccharides owing to the effect of Bacteroidetes in hydrolyzing polysaccharides.45 In co-digestion systems, Firmicutes showed a significant increase in the nano-Al2O3 reactor compared with mono-digestion systems. Firmicutes are an important microbial community with many significant functional microorganisms.46 In summary, nano-Al2O3 had a positive effect on the microbial community. Fig. 7(c) and (d) show the heat maps of archaeal and bacterial genera in different AD systems. The top four archaeal genera were Methanobacterium sp., Methanosarcina sp., Methanosaeta sp., and Methanospirillum sp. Co-digestion resulted in a higher relative abundance of Methanobacterium sp. and Methanosarcina sp. than mono-digestion. Methanobacterium sp. played an important role in the AD process. Methanosarcina sp. is a type of methanogen that produces acetic acid. This suggests that the activities of Methanobacterium sp. and Methanosarcina sp. could be enhanced in co-digestion. Moreover, the relative abundance of Methanobacterium sp. in the nano-Al2O3 reactor was higher than that in the corresponding control reactor. Nano-Al2O3 addition resulted in an increase in the relative abundance of Methanosarcina sp. but a decrease in that of Methanosaeta sp. These results indicated that Methanosarcina sp. and Methanosaeta sp. have a competitive relationship.45 As shown in Fig. 7(d), the dominant bacterial genera in all four groups were Hydrogenispora sp., Lutispora sp., Syntrophomonas sp., Longilinea sp., Hydrogenispora sp., and Ruminiclostridium sp. Hydrogenispora sp., Lutispora sp., and Ruminiclostridium sp. were more abundant in the CTA reactor. Hydrogenispora sp. and Lutispora sp. belong to the Firmicutes phylum and are known to be responsible for the degradation of cellulose and proteins in the AD process.47,48 From the above discussion, it can be seen that nano-Al2O3 addition influenced the abundance and structure of the microbial flora.
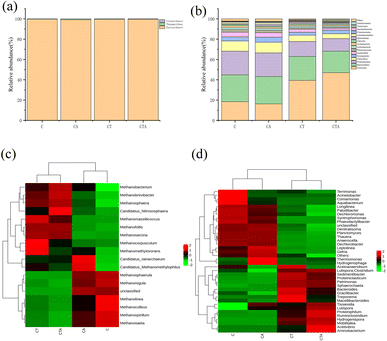 |
| Fig. 7 Archaeal (a) and bacterial community (b) structure at the phylum level. The heat map of the top 18 archaea (c) and 38 bacteria (d) at the genus level in the four reactors during the dry AD process. | |
4 Conclusions
Biogas production in both mono-digestion and co-digestion systems was enhanced with nano-Al2O3 addition to the AD process. Gompertz model analysis demonstrated that nano-Al2O3 nanomaterials shortened the lag phase. Microbial species such as Methanobacterium sp., Hydrogenispora sp., Lutispora sp., and Ruminiclostridium sp. were more abundant in the nano-Al2O3 reactor. Nano-Al2O3 had a positive impact on both mono-digestion and co-digestion systems. Maybe nano-Al2O3 have large specific areas and highly porous structure.49 And nano-Al2O3 is a typical catalyst carrier, which can provide a good carrier for anaerobic medium and promote the occurrence of hydrogenation and redox.50–53 In complex AD process, the effect of Al2O3 as a carrier will be further research in the future.
Author contributions
Hongmei Zhao: conceived and designed the experiments; performed the experiments; analyzed and interpreted the data; contributed reagents, materials, analysis tools or data; wrote the paper. Shibo Chen: conceived and designed the experiments; performed the experiments; analyzed and interpreted the data; contributed reagents, materials, analysis tools or data; wrote the paper. Congqi Zhao; Kejiang Ruan: performed the experiments; analyzed and interpreted the data. Xiaohong Cheng; Junju Xu: analyzed and interpreted the data; contributed reagents, materials, analysis tools or data; wrote the paper.
Conflicts of interest
There are no conflicts of interest to declare.
Acknowledgements
This work was supported by Agricultural Union Youth Program of Yunnan Provincial Science and Technology Department (2018FG001-102) and Scientific Research Fund of Yunnan Education Department (2023J0416). We thank Engineering and Research Center for Industrial Biogas Technology of Yunnan Province University. The archaeal diversity analysis was performed using the Omicshare tools, a free online platform for data analysis (https://www.omicshare.com/tools). We thank LetPub (https://www.letpub.com) for its linguistic assistance during the preparation of this manuscript.
References
- M. M. Hossain, I. M. Scott, F. Berruti and C. Briens, A two-dimensional pyrolysis process to concentrate nicotine during tobacco leaf bio-oil production, Ind. Crops Prod., 2018, 124, 136–141 CrossRef CAS.
- Y. Liu, J. X. Dong, G. J. Liu, H. N. Yang, W. Liu, L. Wang, C. X. Kong, D. Zheng, J. G. Yang, L. W. Deng and S. S. Wang, Co-digestion of tobacco waste with different agricultural biomass feedstocks and the inhibition of tobacco viruses by anaerobic digestion, Bioresour. Technol., 2015, 189, 210–216 CrossRef CAS PubMed.
- J. T. He, N. Yao, Z. Y. Sun, F. Li, H. Q. Cai, L. F. Jin and Y. Q. Tang, Improved biogas production from tobacco processing waste via biochar-assisted thermophilic anaerobic digestion, Ind. Crops Prod., 2021, 202, 117038–117046 CrossRef.
- Z. Yang, S. Zhang, L. Liu, X. Li, H. Chen, H. Yang and X. Wang, Combustion behaviours of tobacco stem in a thermogravimetric analyser and a pilot-scale fluidized bed reactor, Bioresour. Technol., 2012, 110, 595–602 CrossRef CAS PubMed.
- Q. Cheng, W. Huang, M. Jiang, C. Xu, G. Fan, J. Yan, B. Chai, Y. Zhang, Y. Zhang, S. Zhang, B. Xiao and G. Song, Challenges of anaerobic digestion in China, Int. J. Environ. Sci. Technol., 2021, 18, 3685–3696 CrossRef CAS.
- B. T. Bocher, M. T. Agler, M. L. Garcia, A. R. Beers and L. T. Angenent, Anaerobic digestion of secondary residuals from an anaerobic bioreactor at a brewery to enhance bioenergy generation, J. Ind. Microbiol. Biotechnol., 2008, 35, 321–332 CrossRef CAS PubMed.
- F. Tambone, P. Genevini, G. D'Imporzano and F. Adani, Assessing amendment properties of digestate by studying the organic matter composition and the degree of biological stability during the anaerobic digestion of the organic fraction of MSW, Bioresour. Technol., 2009, 100, 3140–3142 CrossRef CAS PubMed.
- M. C. Tomei, Modeling of anaerobic digestion of sludge, Crit. Rev. Environ. Sci. Technol., 2009, 39, 1003–1051 CrossRef CAS.
- Y. Liu, J. X. Dong, G. J. Liu, H. N. Yang, W. Liu, L. Wang, C. X. Kong, D. Zheng, J. G. Yang, L. W. Deng and S. S. Wang, Co-digestion of tobacco waste with different agricultural biomass feedstocks and the inhibition of tobacco viruses by anaerobic digestion, Bioresour. Technol., 2015, 189, 210–216 CrossRef CAS PubMed.
- A. González-González and F. Cuadros, Optimal and cost-effective industrial biomethanation of tobacco, Renewable Energy, 2014, 63, 280–285 CrossRef.
- A. González-González, F. Cuadros, A. Ruiz-Celma and F. López-Rodríguez, Potential application of anaerobic digestion to tobacco plant, Fuel, 2013, 113, 415–419 CrossRef.
- X. Ye, H. Liu, Q. Meng, S. Chen, Z. Hu, S. Sun, J. Ma and X. Yu, Comparison of chemical composition in stalks of different tobaccos, Tob. Sci. Technol., 2013, 76–79 CAS , in Chinese..
- L. G. Wang, H. Y. Zhang, G. Q. Liu, Z. Q. Dai, Y. Liu and C. Chen, Effect of nicotine inhibition on anaerobic digestion and the co-digestion performance of tobacco stalks with different animal manures, Process Saf. Environ. Prot., 2021, 146, 377–382 CrossRef CAS.
- J. T. He, N. Yao, Z. Y. Sun, F. Li, H. Q. Cai, L. F. Jin and Y. Q. Tang, Improved biogas production from tobacco processing waste via biochar-assisted thermophilic anaerobic digestion, Ind. Crops Prod., 2023, 202, 117038 CrossRef CAS.
- C. R. Qi, R. Wang, S. M. Jia, J. Chen, Y. Y. Li, J. X. Zhang, G. X. Li and W. H. Luo, Biochar amendment to advance contaminant removal in anaerobic digestion of organic solid wastes: a review, Bioresour. Technol., 2021, 341, 125827 CrossRef CAS PubMed.
- C. H. Luo, F. Lü, L. M. Shao and P. J. He, Application of eco-compatible biochar in anaerobic digestion to relieve acid stress and promote the selective colonization of functional microbes, Water Res., 2015, 68, 710–718 CrossRef CAS PubMed.
- Q. Li, M. J. Xu, G. J. Wang, R. Chen, W. Qiao and X. C. Wang, Biochar assisted thermophilic co-digestion of food waste and waste activated sludge under high feedstock to seed sludge ratio in batch experiment, Bioresour. Technol., 2018, 249, 1009–1016 CrossRef CAS PubMed.
- S. Barua and B. R. Dhar, Advances towards understanding and engineering direct interspecies electron transfer in anaerobic digestion, Bioresour. Technol., 2017, 244, 698–707 CrossRef CAS PubMed.
- J. Ma, J. Pan, L. Qiu, Q. Wang and Z. Zhang, Biochar triggering multipath methanogenesis and subdued propionic acid accumulation during semicontinuous anaerobic digestion, Bioresour. Technol., 2019, 293, 122026 CrossRef CAS PubMed.
- S. Li, S. Harris, A. Anandhi and G. Chen, Predicting biochar properties and functions based on feedstock and pyrolysis temperature: a review and data syntheses, J. Cleaner Prod., 2019, 215, 890–902 CrossRef CAS.
- J. Pan, J. Ma, L. Zhai, T. Luo, Z. Mei and H. Liu, Achievements of biochar application for enhanced anaerobic digestion: a review, Bioresour. Technol., 2019, 292, 122058 CrossRef CAS PubMed.
- H. B. Nielsen and I. Angelidaki, Strategies for optimizing recovery of the biogas process following ammonia inhibition, Bioresour. Technol., 2008, 99, 7995–8001 CrossRef CAS PubMed.
- L. Y. Feng, Y. G. Chen and X. Zheng, Enhancement of waste activated sludge protein conversion and volatile fatty acids accumulation during waste activated sludge anaerobic fermentation by carbohydrate substrate addition: the effect of pH, Environ. Sci. Technol., 2009, 43, 4373–4380 CrossRef CAS PubMed.
- H. M. Zhao, H. P. Pu and Z. R. Yang, Study on the effect of different additives on the anaerobic digestion of hybrid Pennisetum: Comparison of nano-ZnO, nano-Fe2O3 and nano-Al2O3, Heliyon, 2023, 9, e16313–e16323 CrossRef CAS PubMed.
- A. D. Eaton, A. E. Greenberg, L. S. Cleseri and M. A. H. Franson, Standard Methods for the Examination of Water &Wastewater, 1995 Search PubMed.
- Y. H. Cheng, S. X. Sang, H. Z. Huang, X. J. Liu and J. B. Ouyang, Variation of Coenzyme F420 Activity and Methane Yield in Landfill Simulation of Organic Waste, J. China Univ. Min. Technol., 2007, 17, 403–408 CrossRef CAS.
- R. H. Guan, Studies on the Performance of an Anaerobic Baffled Reactor for Desizing Wastewater, 2010 Search PubMed.
- N. Q. Ren and A. J. Wang, Principles and Applications of Anaerobic Biotechnology, 2004 Search PubMed.
- B. Shamurad, N. Gray, E. Petropoulos, S. Tabraiz, E. Membere and P. Sallis, Predicting the effects of integrating mineral wastes in anaerobic digestion of OFMSW using first-order and Gompertz models from biomethane potential assays, Renewable Energy, 2020, 152, 308–319 CrossRef CAS.
- J. Gu, R. Liu, Y. Cheng, N. Stanisavljevic, L. Li, D. Djatkov, X. Y. Peng and X. M. Wang, Anaerobic co-digestion of food waste and sewage sludge under mesophilic and thermophilic conditions: Focusing on synergistic effects on methane production, Bioresour. Technol., 2020, 301, 122765 CrossRef CAS PubMed.
- Y. Yang, Q. Chen, J. D. Wall and Z. Hu, Potential nanosilver impact on anaerobic digestion at moderate silver concentrations, Water Res., 2012, 46, 1176–1184 CrossRef CAS PubMed.
- E. Kökdemir Ünşar and N. A. Perendeci, What kind of effects do Fe2O3 and Al2O3 nanoparticles have on anaerobic digestion, inhibition or enhancement?, Chemosphere, 2018, 211, 726–735 CrossRef PubMed.
- R. Jaenchen, P. Schönheit and R. K. Thauer, Studies on the biosynthesis of coenzyme F420 in methanogenic bacteria, Arch. Microbiol., 1984, 137, 362–365 CrossRef CAS PubMed.
- Y. Xi, Z. Chang, X. Ye, R. Xu, J. Du and G. Chen, Methane production from wheat straw with anaerobic sludge by heme supplementation, Bioresour. Technol., 2014, 172, 91–96 CrossRef CAS PubMed.
- H. Zhang, Y. Tian, L. Wang, X. Mi and Y. Chai, Effect of ferrous chloride on biogas production and enzymatic activities during anaerobic fermentation of cow dung and Phragmites straw, Biodegradation, 2016, 27, 69–82 CrossRef CAS PubMed.
- Q. Jiang, H. Liu, Y. Zhang, M. H. Cui, B. Fu and H. B. Liu, Insight into sludge anaerobic digestion with granular activated carbon addition: Methanogenic acceleration and methane reduction relief, Bioresour. Technol., 2021, 319, 124131–124140 CrossRef CAS PubMed.
- Y. L. T, H. Y. Zhang, L. Zheng, S. S. Li, H. Hao and H. Huang, Effect of Zn Addition on the Cd-Containing Anaerobic Fermentation Process: Biodegradation and Microbial Communities, Int. J. Environ. Res. Public Health, 2019, 16, 2998 CrossRef PubMed.
- S. Y. Gu, R. J. Wang, H. G. Xing, M. Z. Yu, S. Y. Shen, L. Zhao, J. Y. Sun and Y. Li, Effects of different low temperature conditions on anaerobic digestion efficiency of pig manure and composition of archaea community, Water Sci. Technol., 2022, 86(5), 1181–1193 CrossRef CAS PubMed.
- W. F. Song, Study on the Separation and Promotion of Low-Temperature Functional Microorganisms in Biogas Digestion, Chinese Academy of Agricultural Sciences, Beijing, China, 2011 Search PubMed.
- J. I. Bueno-Lopez, J. R. Rangel-Mendez, F. Alatriste-Mondraon, F. Perez-Rodriguez, V. Hernandez-Montoya and F. J. Cervantes, Graphene oxide triggers mass transfer limitations on the methanogenic activity of an anaerobic consortium with a particulate substrate, Chemosphere, 2018, 211, 709–716 CrossRef CAS PubMed.
- L. Ding, Z. Liu, M. Aggrey, C. Li, J. Chen and L. Tong, Nanotoxicity: the toxicity research progress of metal and metal- containing nanoparticles, Mini-Rev. Med. Chem., 2015, 15, 529–542 CrossRef CAS PubMed.
- Y. W. Chen, Z. H. Yang, Y. R. Zhang, Y. P. Xiang, R. Xu, M. Y. Jia, J. Cao and W. P. Xiong, Effects of different conductive nanomaterials on anaerobic digestion process and microbial community of sludge, Bioresour. Technol., 2020, 304, 123016–123026 CrossRef CAS PubMed.
- S. Gullert, Deep metagenome and metatranscriptome analyses of microbial communities affiliated with an industrial biogas fermenter, a cow rumen, and elephant feces reveal major differences in carbohydrate hydrolysis strategies, Biotechnol. Biofuels, 2016, 9, 121–141 CrossRef PubMed.
- W. Kim, K. H. Wang, S. G. Shin, S. Lee and S. Hwang, Effect of high temperature on bacterial community dynamics in anaerobic acidogenesis using mesophilic sludge inoculum, Bioresour. Technol., 2010, 101, S17–S22 CrossRef CAS PubMed.
- H. U. Cho, Y. M. Kim, Y. N. Choi, H. G. Kim and J. M. Park, Influence of temperature on volatile fatty acid production and microbial community structure during anaerobic fermentation of microalgae, Bioresour. Technol., 2015, 191, 475–480 CrossRef CAS PubMed.
- I. Vanwonterghem, P. D. Jensen, D. P. Ho, D. J. Batstone and G. W. Tyson, Linking microbial community structure, interactions and function in anaerobic digesters using new molecular techniques, Curr. Opin. Biotechnol., 2014, 27, 55–64 CrossRef CAS PubMed.
- W. Y. Chen, Z. H. Yang, Y. R. Zhang, Y. P. Xiang, R. Xu, M. Y. Jia, J. Cao and W. P. Xiong, Effects of different conductive nanomaterials on anaerobic digestion process and microbial community of sludge, Bioresour. Technol., 2020, 304, 123016–123026 CrossRef PubMed.
- Y. Bai, R. Xu, Q. P. Wang, Y. R. Zhang and Z. H. Yang, Sludge anaerobic digestion with high concentrations of tetracyclines and sulfonamides: Dynamics of microbial communities and change of antibiotic resistance genes, Bioresour. Technol., 2019, 276, 51–59 CrossRef CAS PubMed.
- K. Chen, J. Wan, J. Lin and R. Zhou, Comparative study of three-way catalytic performance over Pd/CeO2-ZrO2-Al2O3 and Pd/La-Al2O3 catalysts: New insights into microstructure and thermal stability, Mol. Catal., 2022, 526, 112361–112374 CrossRef CAS.
- Z. Q. Yu, Y. Li, Y. L. Yao, Y. Y. Liu, Z. C. Sun, C. Shi, W. Wang and A. J. Wang, Highly selective hydrogenative ring-rearrangement of furfural to cyclopentanone over a bifunctional Ni3P/γ-Al2O3 catalyst, Mol. Catal., 2022, 522, 112239–112248 CrossRef CAS.
- S. Liang, T. Cai, J. Yuan, Q. Tong and X. J. Hu, Promoting effect of reduction-oxidation strategy on the Co3O4/γ-Al2O3 catalysts for propane total oxidation, Mol. Catal., 2022, 533, 112762–112772 CrossRef CAS.
- S. J. Park, S. H. Kang, H. K. Min, M. G. Seo, S. Kweon, M. B. Park, Y. H. Choi and J. W. Lee, Catalytic pyrolysis of HDPE over WOx/Al2O3: Effect of tungsten content on the acidity and catalytic performance, Mol. Catal., 2022, 528, 112439–112447 CrossRef CAS.
- J. Y. Liang, F. Y. Wang, W. Li, J. L. Zhang and C. L. Guo, Highly dispersed and stabilized Pd species on H2 pre-treated Al2O3 for anthraquinone hydrogenation and H2O2 production, Mol. Catal., 2022, 524, 112264–112274 CrossRef CAS.
Footnote |
† Both authors contributed equally to this work. |
|
This journal is © The Royal Society of Chemistry 2023 |