DOI:
10.1039/D2QM01008A
(Research Article)
Mater. Chem. Front., 2023,
7, 85-95
Regulation of excited-state properties of dibenzothiophene-based fluorophores for realizing efficient deep-blue and HLCT-sensitized OLEDs†
Received
4th October 2022
, Accepted 4th November 2022
First published on 5th November 2022
Abstract
Efficient and multifunctional deep-blue organic luminescent materials are in great demand for the development of organic light-emitting diodes (OLEDs) in the application of ultrahigh-definition displays with a wide color gamut and highly saturated color. Herein, two deep-blue hybridized local and charge-transfer (HLCT) dibenzothiophene-phenanthroimidazole fluorophores were designed and synthesized by regulating the electron-withdrawing abilities of anchoring groups (phenyl and cyanophenyl) at the phenanthroimidazole unit. Computational and photophysical investigations reveal that the introduction of a cyano-group can finely modulate the component of excited states while maintaining similar luminescence colors. Consequently, the resulting non-doped devices show electroluminescence peaks at 451 and 452 nm with the Commission Internationale de l’Éclairage (CIE) coordinates of (0.152, 0.067) and (0.153, 0.064). Based on HLCT-sensitized fluorescence and phosphorescence, high external quantum efficiencies (EQEs) of 7.08% and 27.30% and low efficiency roll-off are achieved concurrently at low doping concentrations. Moreover, a high-performance hybrid warm-white OLED with an EQE of 26.56% is obtained using the incomplete energy transfer process.
Introduction
Organic luminescent materials (OLMs) have attracted widespread attention in the application of organic light-emitting diodes (OLEDs), organic lasers, organic light-emitting transistors (OFETs), biological imaging devices, chemical sensors, and so on.1–4 Especially in recent years, the rapid development of OLEDs in full-color displays (televisions, smartphones, smart watches, etc.) has pushed up demand for OLMs. In terms of three primary-color (red, green, and blue) OLEDs, red and green OLEDs based on phosphorescent metallic complexes have met the market demand in the device efficiency and operating lifetime. However, blue OLEDs, especially deep-blue OLEDs that matched the Commission international de I'Eclairage (CIE) coordinate of CIEy < 0.08 are far behind them,5–7 resulting from their inherent wide bandgap feature. Though traditional blue fluorescent emitters possess excellent device stability, only 25 percent of electrogenerated excitons (singlet excitons) can be harvested for emitting light, and others (triplet excitons) are turned into heat and other forms of energy to be dissipated, leading to a low electroluminescence (EL) performance with the upper limit of external quantum efficiency (EQE) of ∼5%. Blue phosphorescent and thermally activated delayed fluorescent (TADF) emitters can harvest both singlet and triplet excitons, realizing 100% theoretical internal quantum efficiency, but they have to be doped into a suitable host with a triplet state energy higher than that of emitters for weakening concentration quenching and triplet exciton annihilation effects.8–11 In addition, the dependable deep-blue emission and color purity of phosphorescent and TADF emitters still remain unsatisfactory owing to their relatively strong metal–ligand charge-transfer (MLCT) and charge-transfer (CT) excited states. What is more, an efficient deep-blue material should not only serve as an emitter for full-color displays to decrease the power loss but also be used as a host for white OLEDs (WOLEDs) to generate longer wavelength emissions in lighting applications.12,13 Until now, it is still an urgent demand for the development and application of OLED technology to develop such multifunctional deep-blue materials with CIEy < 0.08.
The dibenzothiophene (DBT) group with a rigid and planar molecular structure possesses flexible structural modification, excellent thermal stability, a narrow emission spectrum, a high triplet energy level, and somewhat bipolar-transporting properties,14,15 and has been utilized in a wide variety of optoelectronic applications, including OLEDs, OFETs, and polymer solar cells (PSCs). Moreover, the heavy atom effect of sulfur can enhance the spin–orbit coupling (SOC), which can effectively modulate the intersystem crossing (ISC) and reversed intersystem crossing (RISC) processes. However, most of the reported DBT derivatives were used as the host for phosphorescent and TADF dopants16–27 and connected with acceptor or donor units to construct electron- or hole-transporting materials,28–30 and only a few of them were used as yellow, green, and blue emitters in OLEDs.26,31,32 For example, Guo et al. developed a series of green and yellow TADF emitters with DBT as a donor unit, achieving high EQEs of over 9% in the non-doped devices.33,34 Tang and co-workers synthesized a dual-emitting-core compound (2DBT-BZ-2Cz) with green emission, demonstrating a current efficiency of 20.7 cd A−1 in a solution-processed OLED.35 Xia et al. reported a dibenzothiophene-triphenylamine derivative (ST-G1) with an EL peak at 445 nm, realizing a current efficiency of 0.48 cd A−1.36 Sim et al. achieved pyrene derivatives (DDPI and DPPI) including the dibenzothiophene moiety, realizing an EQE of 3.07% and CIE coordinates of (0.16, 0.16).37 Lee and co-workers developed an N,N-diphenyl aminofluorene styryl emitter with DBT as the end group, showing a current efficiency of 8.11 cd A−1 and CIE coordinates of (0.154, 0.163) in the doped device with typical triplet–triplet annihilation (TTA) molecule ADN as the host.38 Yao and Tang reported two blue fluorescent DBT-bridged molecules (D1 and PISF) with EQE values of 1.7% and 2.60% in non-doped devices, showing the CIE coordinates of (0.17, 0.09) and (0.16, 0.09).39,40 The DBT-based fluorophores with CIEy < 0.08 have not been reported previously in non-doped OLEDs. Moreover, there is still much room for further improving the EL efficiency and multifunction through molecular and device engineering.
It is known that phenanthroimidazole (PI) with suitable energy levels, high luminous efficiency, and bipolar-transporting characteristics is a representative building block for blue materials. In this work, two novel deep-blue DBT-PI fluorophores (PIS and CNPIS) were designed and synthesized by regulating the electron-withdrawing ability of anchoring groups (phenyl and cyanophenyl) at the N1 position of the PI unit. Because of the large dihedral angles, cyano modification has a small effect on the emission color, but it reduces the lowest-excited singlet–triplet (S1–T1) energy gap (ΔEST) and enhances the CT state components in the S1 state. As a result, utilizing the phenyl-substituted PIS as the sensitizer in OLEDs, high EQEs of 7.08%, 27.30%, and 26.56% and low efficiency roll-off at high luminance are achieved simultaneously.
Experimental methods
Synthesis and characterization of materials
Synthesis of 2-(4-(dibenzo[b,d]thiophen-4-yl)phenyl)-1-phenyl-1H-phenanthro[9,10-d]imidazole (PIS).
Under a nitrogen atmosphere, 2-(4-bromophenyl)-1-phenyl-1H-phenanthro[9,10-d] imidazole (2.25 g, 5 mmol) dibenzo[b,d]thiophen-4-ylboronic acid (1.37 g, 6 mmol), Pd(PPh3)4 (0.23 g, 0.2 mmol), CH3CH2OH (10 mL), toluene (30 mL) and 2 M solution of K2CO3 in water (10 mL) were added to a 100 mL two-neck round-bottom flask and refluxed at 110 °C for 24 h. After cooling down, the reaction mixture was extracted three times using CH2Cl2 and water. The organic layer was dried with anhydrous MgSO4, filtered, and evaporated under reduced pressure. The crude product was purified by silica-gel column chromatography using petroleum/CH2Cl2 (1/1, v/v) as the solvent and finally further purified by a multi-temperature-zone sublimator to give a white solid (1.65 g, 60%). 1H NMR (500 MHz, CDCl3, δ ppm): 8.96 (d, J = 7.9 Hz, 1H), 8.75 (dd, J = 28.5, 8.3 Hz, 2H), 8.24–8.03 (m, 2H), 7.86–7.81 (m, 1H), 7.81–7.72 (m, 3H), 7.67 (dd, J = 20.0, 8.1 Hz, 6H), 7.58 (d, J = 7.2 Hz, 2H), 7.52 (t, J = 7.6 Hz, 2H), 7.49–7.42 (m, 3H), 7.28 (t, J = 8.1 Hz, 1H), 7.21 (d, J = 8.2 Hz, 1H). 13C NMR (126 MHz, CDCl3, δ ppm): 150.53, 141.04, 139.64, 138.91, 138.55, 136.46, 136.46, 136.32, 135.80, 130.38, 130.03, 129.81, 129.48, 129.29, 128.47, 128.23, 127.45, 126.98, 126.41, 125.80, 125.20, 125.06, 124.53, 124.25, 123.26, 123.18, 122.96, 122.72, 121.83, 121.01, 120.82. TOF-MS(ESI) m/z calcd. for C39H24N2S: 552.1660; [M + H]+ found: 553.1734.
Synthesis of 4-(2-(4-(dibenzo[b,d]thiophen-4-yl)phenyl)-1H-phenanthro[9,10-d]imidazol-1-yl)benzonitrile (CNPIS).
Under a nitrogen atmosphere, 4-(2-(4-bromophenyl)-1H-phenanthro[9,10-d]imidazol-1-yl)benzonitrile (2.37 g, 5 mmol) dibenzo[b,d]thiophen-4-ylboronic acid (1.37 g, 6 mmol), Pd(PPh3)4 (0.23 g, 0.2 mmol), CH3CH2OH (10 mL), toluene (30 mL) and 2 M solution of K2CO3 in water (10 mL) were added to a 100 mL two-neck round-bottom flask and refluxed at 110 °C for 24 h. After cooling down, the reaction mixture was extracted using CH2Cl2 and water. The organic layer was dried using anhydrous MgSO4, filtered, and evaporated under reduced pressure. The crude product was purified by silica-gel column chromatography using petroleum/CH2Cl2 (2/3, v/v) as the solvent and finally further purified by a multi-temperature-zone sublimator to give a white solid (1.51 g, 52%). 1H NMR (400 MHz, CDCl3, δ ppm): 8.92 (d, J = 7.9 Hz, 1H), 8.84–8.77 (m, 1H), 8.71 (d, J = 8.2 Hz, 1H), 8.22–8.11 (m, 2H), 8.00–7.92 (m, 2H), 7.89–7.82 (m, 1H), 7.80–7.61 (m, 8H), 7.61–7.51 (m, 2H), 7.51–7.42 (m, 3H), 7.33 (ddd, J = 8.3, 7.0, 1.2 Hz, 1H), 7.13 (dd, J = 8.3, 1.3 Hz, 1H). 13C NMR (101 MHz, CDCl3, δ ppm): 158.10, 153.52, 148.58, 143.11, 139.54, 138.49, 136.55, 134.26, 131.74, 130.89, 130.44, 130.07, 129.71, 128.59, 128.55, 127.77, 127.11, 127.04, 126.74, 126.36, 125.62, 125.29, 124.64, 124.61, 123.30, 123.15, 122.81, 122.45, 121.89, 121.07, 120.67, 117.83. TOF-MS(ESI) m/z calcd. for C40H23N3S: 577.1613; [M + H]+ found: 578.1668.
Results and discussion
Synthesis and thermal properties
As shown in Scheme 1, the target compounds (PIS and CNPIS) were synthesized from the Suzuki-coupling procedure between dibenzo[b,d]thiophen-4-yl boronic acid (DBT-B) and 2-(4-bromophenyl)-1-phenyl-1H-phenanthro[9,10-d]imidazole (PI-Br) and 4-(2-(4-bromophenyl)-1H-phenanthro[9,10-d]imidazol-1-yl)benzonitrile (CNPI-Br), where PI-Br and CNPI-Br were obtained based on different literature studies.41–43 After purifying by vacuum sublimation, the structures of PIS and CNPIS were confirmed by NMR spectra and mass spectrometry. Their detailed synthesis and characterization are shown in the Experimental Section. Thermal gravimetric analysis (TGA) and differential scanning calorimetry (DSC) were performed to investigate the thermal stability of PIS and CNPIS. As depicted in Fig. S1 (ESI†) and Table 1, decomposition temperatures (Tds) with a 5% weight loss were 404 and 423 °C for PIS and CNPIS, respectively. The glass-transition temperature (Tg) of PIS was measured to be 127 °C, while no obvious Tg was found upon second heating for CNPIS.
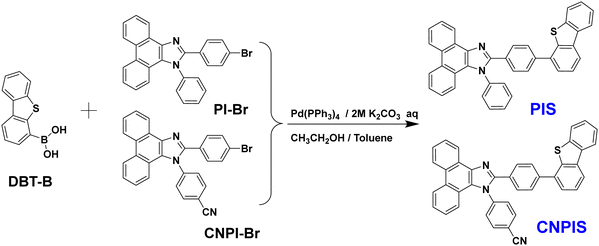 |
| Scheme 1 The chemical equations for the synthesis of PIS and CNPIS. | |
Table 1 Photophysical, thermal and electrochemical properties of PIS and CNPIS are summarized
Compound |
λ
F,S
[nm] |
λ
F,F
[nm] |
E
S/ET/ΔESTc [eV] |
HOMO/LUMO/Egd [eV] |
T
g/Tde [°C] |
τ
F
[ns] |
Φ
PL
[%] |
Measured in the THF solution (10−5 M) at room temperature.
Measured in the neat film at room temperature.
Measured in the DCM solution (10−5 M) at 77 K.
HOMO obtained from CV; LUMO energy calculated by adding the optical band gap to HOMO; Eg calculated form the absorption onset.
Measured by DSC and TGA.
|
PIS
|
408 |
432 |
2.94/2.38/0.56 |
−5.46/−2.23/3.23 |
127/404 |
1.19 |
49.70 |
CNPIS
|
423 |
438 |
2.95/2.39/0.56 |
−5.47/−2.25/3.22 |
—/423 |
2.26 |
30.37 |
Photophysical properties
Ultraviolet-visible (UV-vis) absorption and photoluminescence (PL) spectra of target materials were recorded to investigate their photophysical properties. As shown in Fig. 1 and Table 1, PIS and CNPIS show similar absorption peaks’ location at ∼337 and ∼362 nm in the dilute tetrahydrofuran solution, corresponding to the π–π* transition of the substituent on the 2-imidazole position to the PI unit and π–π* transition of the PI unit.31,44 The optical bandgaps (Egs) of PIS and CNPIS can be calculated to be 3.23 and 3.22 eV from the absorption onset, respectively. The PL emission peak of PIS is located at 408 nm, while CNPIS shows an obvious red-shift emission with a maximum peak of 423 nm due to the intramolecular charge transfer (ICT) effect induced by cyano-substitution. In the neat film, due to intermolecular interactions, both of them exhibit red-shifted emission but are still located at the deep-blue region with peaks of 432 and 438 nm for PIS and CNPIS, respectively. The absolute PL quantum yield (PLQY) of the PIS film is 49.70%, higher than that of CNPIS (30.37%).
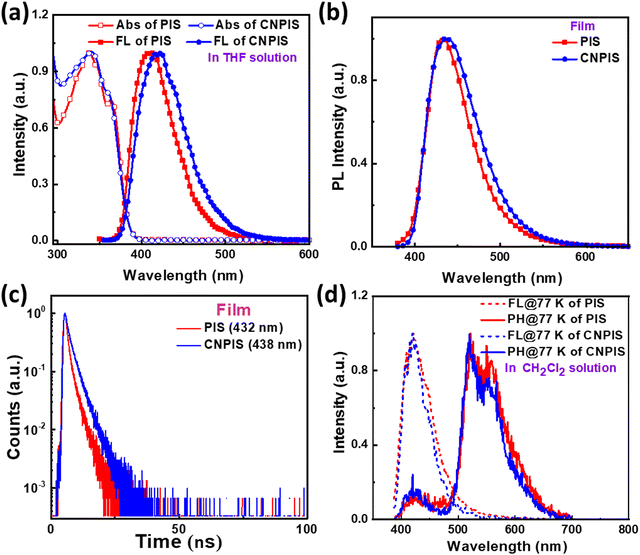 |
| Fig. 1 (a) UV-vis absorption and emission spectra of PIS and CNPIS in dilute THF solution (10 μM) at room temperature. (b) Emission spectra of PIS and CNPIS thin films. (c) Transient PL decay spectra of PIS and CNPIS thin films. (d) Low-temperature fluorescence and phosphorescence spectra of PIS and CNPIS in the dichloromethane solution. | |
To further study the property of excited states of PIS and CNPIS, their solvatochromic effects in different solvents were measured. As shown in Fig. S2, S3, and Tables S1, S2 (ESI†), by increasing the solvent polarity from hexane to acetonitrile, the absorption spectra are insensitive to the solvent polarity, while the PL spectra show a notable red shift from 386 to 418 nm for PIS and from 404 to 434 nm for CNPIS, manifesting the presence of the ICT excited state. For PIS with phenyl modification at the N1 of the PI unit, the PL spectra feature a vibrational fine emission in low-polar solvents and convert to broad featureless emission in high-polar solvents. However, the cyanophenyl-substitution changes the spectral pattern that CNPIS displays broad and structureless characteristics in all the solvents, demonstrating that the introduction of a cyano-group can effectively regulate the component of excited states. As shown in Fig. S4 (ESI†), Stokes shift (νa − νPL) versus orientation polarizability (f) characteristics of PIS and CNPIS were fitted by the Lippert–Mataga model. PIS shows two section lines with the excited-state dipole moments (μes) of 8.66 and 17.72 D in low- and high-polar solvents, belonging to typical hybridized local and charge-transfer (HLCT) characteristic. The well-fitted linear relationship with the μe of 12.43 D was achieved based on CNPIS, smaller than that of the typical CT molecule DMABN (23 D),45 which may be regarded as a quasi-equivalent HLCT excited state.42,46–50
As depicted in Fig. S5 and Table S3 (ESI†), the mono-exponential decays with nanosecond-scale lifetimes in different solvents, further illustrating that there is only one excited state rather than a mixture of the LE and CT states, which is proved in the theoretical calculation section. The decay lifetimes of 1.19 and 2.26 ns for PIS and CNPIS were observed in the neat film (Fig. 1(c)). According to the PLQYs, the radiative transition rates (krs) of PIS and CNPIS can be calculated to be 4.18 and 1.34 × 108 s−1. And non-radiative transition rates (knrs) were calculated to be 4.23 and 3.08 × 108 s−1, respectively (summarized in Table S3, ESI†). From low-temperature fluorescence and phosphorescence spectra in the dichloromethane solution (Fig. 1(d)), the values of S1 and T1 energy levels were estimated to be 2.94/2.38 eV and 2.95/2.39 eV for PIS and CNPIS. Their ΔEST values are as high as 0.56 and 0.56 eV (Table 1). These results signify that the TADF process can be eliminated in this molecular system.
Theoretical calculation and electrochemical properties
The molecular geometries and electronic structures of PIS and CNPIS were investigated by density functional theory calculations using Gaussian 09 at the B3LYP/6-31G(d,p) level. As displayed in Fig. 2, both of them show similar twist angles (47.89° and 46.38°) between the PI unit and the DBT group, while the cyano-substituted CNPIS shows a relatively low twist angle between the cyanophenyl and imidazole ring. The introduction of the DBT group has a small effect on the twist angles between imidazole and adjacent benzene rings in the PI unit (Fig. S6, ESI†). It is noteworthy that both PIS and CNPIS show quite similar highest occupied molecular orbital (HOMO) distributions that are mainly distributed on phenanthrene, imidazole, and adjacent benzene rings and to a certain extent remain on the DBT unit. Nevertheless, their lowest unoccupied molecular orbital (LUMO) distributions are markedly different. The LUMO of PIS is delocalized almost on the entire molecular skeleton, while that of CNPIS is mainly located on the cyanophenyl and adjacent imidazole ring due to the strong electron-withdrawing ability of the cyano group. Fascinatingly, LUMO+1 of CNPIS is located on the whole PI-DBT framework, which is similar to the LUMO of PIS (Fig. S7, ESI†). The energy gap between HOMO and LUMO+1 of CNPIS (3.96 eV) is close to that of PIS (3.86 eV) from the HOMO to the LUMO. Due to efficient overlaps in the molecular orbitals for PIS, the transition from the HOMO to the LUMO is allowed. As for CNPIS, as a result of spatial separation between the HOMO and the LUMO, it is nearly forbidden in the photo-absorption process, whereas the transition from the HOMO to LUMO+1 should be allowed.51 These results agree well with the approximately equal Eg between PIS and CNPIS. Their electrochemical properties were performed using cyclic voltammetry (CV) measurements. The HOMO levels of PIS and CNPIS were deduced to be −5.46 and −5.47 eV (Fig. S8, ESI†), and the LUMO levels were computed to be −2.23 and −2.25 eV according to the formula: ELUMO = EHOMO + Eg.
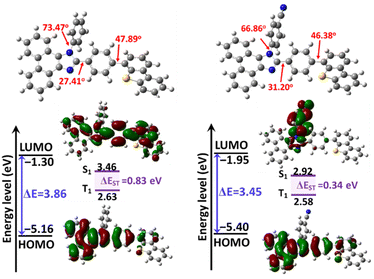 |
| Fig. 2 Optimized geometries, HOMO and LUMO distributions and their energy levels of PIS and CNPIS computed at the B3LYP/6-31G (d,p) level. | |
For gaining an in-depth understanding of the influences of the cyano-group on excited state properties, calculation of natural transition orbitals (NTOs) of PIS and CNPIS was further carried out. As shown in Fig. S9 and S10 (ESI†), during the S0 → S1 transition of PIS, the hole is mainly located on the PI unit and to some extent dispersed in the DBT group, while the particle is mainly distributed in DBT and the adjacent benzene moieties. There are obvious spatial separations and partial overlaps between the hole and particle NTOs, belonging to the typical HLCT state features. Additionally, it is also observed in the S0 → S2 transition. For CN-modified CNPIS, the obvious CT transition with the particle located on benzonitrile and hole mainly delocalized in phenanthrene, imidazole, and adjacent benzene rings can be found in the S0 → S1 transition, but the S0 → S2 transition shows the distinct HLCT state. It can be seen from the calculated first-ten singlet and triplet excited states that a large ΔEST of 0.831 eV and the small energy gaps (0.0381/−0.0941 eV) between S1 and fifth/sixth triplet (T5/T6) excited states in molecule PIS can facilitate the high-lying reverse intersystem crossing (hRISC) process from T5/T6 to S1. Though T5 and T6 exhibit LE-dominant character (Fig. S9, ESI†), according to Fermi's golden rule,52 the large spin–orbit coupling (SOC) strengths of 0.636/0.709 cm−1 between S1 and T5/T6 can effectively enhance the hRISC process (Fig. 3(a) and Table S4, S6, ESI†). As for CNPIS, S1 and second triplet (T2) states with prominent CT and HLCT characters (Fig. S10, ESI†) have the same transition configurations of 98.6% and 22.8% in HOMO → LUMO, respectively, according to El-Sayed’ rule, which is beneficial to establish the transformation channel from triplet to singlet states.53,54 In addition, the low energy gap of 0.0238 eV and a considerable SOC strength of 0.412 cm−1 between S1 and T2 can further accelerate the hRISC process (Fig. 3(b) and Table S5, ESI†). Therefore, the OLEDs with PIS and CNPIS as emitters are expected to exceed the theoretical upper bound of exciton utilization efficiency (EUE) based on traditional fluorescent OLEDs. By contrast, due to the larger ΔEST and SOC strength, the hRISC process of PIS should be more effective than that of CNPIS.
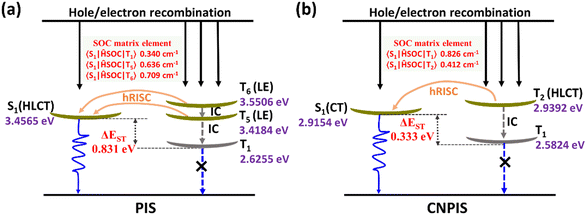 |
| Fig. 3 Probable emission mechanism based on the HLCT state for PIS (a) and CNPIS (b). S and T are singlet and triplet excited states; LE and CT represent local excited and charge-transfer states; ΔEST is the singlet–triplet energy splitting between S1 and T1; IC and hRISC are the internal conversion and the high-lying reverse intersystem crossing. | |
Deep-blue non-doped OLEDs
Encouraged by the conspicuous excited state properties of PIS and CNPIS, we further studied the effect of CN modification on EL performance. Thus, the non-doped OLEDs (D1 and D2) with the configuration of indium tin oxide (ITO)/1,4,5,8,9,11-hexaazatriphenylene-hexacarbonitrile (HATCN, 10 nm)/4,4′-cyclohexylidenebis[N,N-bis(4-methylphenyl)benzenamine] (TAPC, 50 nm)/4,4′,4is(4-methylphenyl)benzenamine] (TCTA, 10 nm)/PIS or CNPIS (20 nm)/1,3,5-tri(m-pyrid-3-yl-phenyl)benzene (TmPyPB, 40 nm)/lithium fluoride (LiF, 1 nm)/aluminium (Al, 100 nm) were fabricated and tested. Here, ITO and Al were used as the anode and the cathode; HATCN and LiF served as hole- and electron-injection layers; TAPC and TCTA acted as hole-transporting and exciton-blocking layers; TmPyPB was used as electron-transporting and hole-blocking layers, respectively. Energy level diagram and chemical structures of organic materials used in the devices are shown in Fig. S11 (ESI†). As shown in Fig. 4, Fig. S12, S13, ESI† and Table 2, owing to efficient hole/electron injection, transporting, and recombination, devices D1 and D2 realize the low turn-on voltages of 2.9 and 3.0 eV, lower than the values of Eg. As for D1, the luminance of 1000 cd m−2 and its maximum value (13260 cd m−2) are achieved at the voltages of 4.2 and 7.4 V. The maximum current efficiency (CE), power efficiency (PE), and EQE are 2.87 cd A−1, 2.95 lm W−1, and 4.59%, respectively. However, device D2 with CN-modified CNPIS as an emissive layer shows a low EQE of 2.59%, which should be attributed to the poor PLQY in its film. More interestingly, both of the devices exhibit stable deep-blue EL spectra with close CIE coordinates of (0.152, 0.067) and (0.153, 0.064) for D1 and D2, further testifying that cyano-substitution in the PI unit has very little effect on emission.
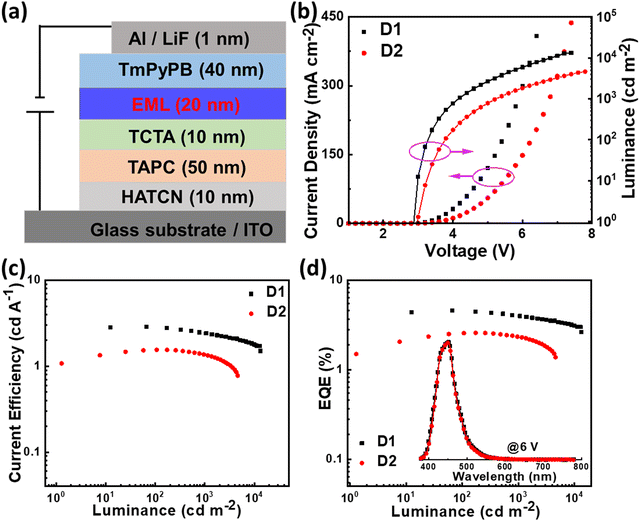 |
| Fig. 4 (a) Device configuration diagram. (b) Current–density–voltage–luminance characteristics of the fabricated OLEDs (D1 and D2). (c) Current efficiency versus luminance curves of devices D1 and D2. (d) EQE versus luminance curves of devices D1 and D2. Insert: Normalized EL spectra at the voltage of 6 V. | |
Table 2 Summary of key EL parameters for the devices
Devices |
V
on
[V] |
CEmax/1000a [cd A−1] |
PEmax/1000a [lm W−1] |
EQEmax/1000a [%] |
CIEb (x, y) |
Maximum efficiencies and the values taken at 1000 cd m−2.
Measured at the voltage of 6 V.
Efficiencies taken at 10 000 cd m−2.
At 1 and 1000 cd m−2.
Efficiencies taken at 5000 cd m−2.
|
D1
|
2.9/4.2 |
2.87/2.42 |
2.95/1.81 |
4.59/3.99 |
(0.152, 0.067) |
D2
|
3.0/5.3 |
1.55/1.36 |
1.36/0.78 |
2.59/2.33 |
(0.153, 0.064) |
D3
|
2.8/3.8 |
8.37/6.71/5.82c |
9.38/5.60 |
7.08/6.35/5.64c |
(0.136, 0.129) |
D4
|
2.9/4.2 |
7.29/6.43/4.81c |
6.78/4.90 |
5.55/4.95/3.82c |
(0.132, 0.170) |
Y1
|
2.7/3.6 |
87.77/80.26 |
98.43/70.73 |
27.30/24.65/22.15e |
(0.482, 0.505) |
Y2
|
2.7/3.6 |
85.17/77.10 |
95.51/68.10 |
26.73/24.25/21.90e |
(0.497, 0.502) |
Y3
|
2.6/3.5 |
79.42/72.50 |
95.91/65.90 |
25.41/23.43/21.80e |
(0.509, 0.494) |
W
|
2.7/3.6 |
85.85/76.60 |
96.27/67.60 |
26.56/23.94/20.08e |
(0.459, 0.487) |
The EUE of OLEDs can be described by eqn (1):
| 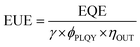 | (1) |
Here,
ϕPLQY is the PLQY of the neat film (49.70% for
PIS; 30.37% for
CNPIS);
γ is the holes and electrons recombination efficiency (≤ 1);
ηout is the light out-coupling efficiency (∼ 20%). The EUEs can be calculated to be 46.18% and 42.64% for
PIS and
CNPIS, exceeding the theoretical limit of traditional fluorescent emitters. Notwithstanding, the phenomenon that cyano-substitution leads to a slight reduction of EUE is distinguished from other reports,
42,51 which may be caused by the special properties of the DBT unit with the third-row sulfur (ZN = 16) atom.
OLEDs based on HLCT-sensitized fluorophore and phosphor
Though the OLEDs (D1 and D2) with PIS and CNPIS as pure emissive layers have achieved a high EUE of over 25%, the EQEs are less than 5% owing to the poor PLQYs of their neat films. Inspired by the TADF-sensitized fluorescent and TADF-sensitized phosphorescence mechanisms reported by Duan et al., they can simultaneously achieve high efficiency and low roll-off OLEDs even at low doping concentrations.55–57 HLCT emitters with the separated exciton radiative transition and triplet–singlet conversion channels should be a promising class of host materials for fluorescence and phosphorescence emitters. Taking PIS as an example, in the HLCT-sensitized fluorescence (HSF) mechanism (Fig. 5(a)), electrons and holes recombine on PIS molecules and form singlet and triplet excitons. Triplet excitons can be effectively converted into singlet excitons in the hRISC process. And the singlet excitons transfer their energy to the fluorophore by Förster resonance energy transfer (FET), resulting in emitting light from the fluorophore. As for the HLCT-sensitized phosphorescent (HSP) mechanism (Fig. 5(b)), phosphorescence emission mainly comes from the FET process from the initial and formed singlet excitons up-converted from triplet excitons on the PIS host. Some lowest T1 excitons generated from internal conversion can also transfer their energy to phosphor by Dexter energy transfer (DET) due to the T1 of PIS being higher than that of phosphor.
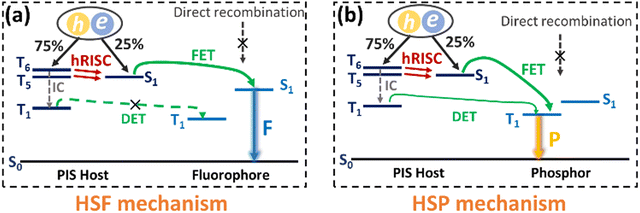 |
| Fig. 5 (a) HLCT-sensitized fluorescence mechanism. (b) HLCT-sensitized phosphorescence mechanism. S and T are singlet and triplet excited states; IC and hRISC are the internal conversion and the high-lying reverse intersystem crossing; FET and DET represent the Förster resonance energy transfer and the Dexter energy transfer, respectively. | |
Based on the above discussion, the blue HSF OLEDs (D3 and D4) with the optimized structure of ITO/HATCN (10 nm)/TAPC (50 nm)/TCTA (10 nm)/PIS: 3 wt% BD (20 nm) for D3 or CNPIS: 3 wt% BD (20 nm) for D4/TmPyPB (40 nm)/LiF (1 nm)/Al (100 nm) have been fabricated, where N,N′-bis(3-methylphenyl)-N,N′-bis[3-(9-phenyl-9H-fluoren-9-yl)phenyl]-pyrene-1,6-diamine (BD) is a blue conventional fluorescent material with the S1 and T1 of 2.68 and 1.81 eV.58 As shown in Fig. S11c (ESI†), the absorption spectrum of BD (in film state) has large overlaps with the PL spectra of PIS and CNPIS, implying that the FET process to BD molecules should be very efficient. EL performance is shown in Fig. 6(a) and Fig. S12 (ESI†), and summarized in Table 2. Compared with deep-blue devices (D1 and D2), HLCT-sensitized fluorescent devices realize higher luminance and superior performance with the maximum CE, PE, and EQE of 8.37 cd A−1, 9.38 lm W−1, and 7.08% for D3, and 7.29 cd A−1, 6.78 lm W−1, and 5.55% for D4. The EQEs have exceeded the limit of 5% in conventional fluorescent OLEDs. More surprisingly, the EQE of device D3 with PIS as the host remains as high as 6.35% at the luminance of 1000 cd m−2, showing a low efficiency roll-off. Even at 10
000 cd m−2, the value remains above 5.6%. The higher EL efficiency achieved in device D3 may be ascribed to a more effective hRISC process. Meanwhile, devices D3 and D4 realize the stable blue emission with the EL peaks at 462 and 468 nm and the narrow full-width at half-maximums (FWHMs) of 42 and 43 nm (Fig. S13c and S12d, ESI†), corresponding to the CIE coordinates of (0.136, 0.129) and (0.132, 0.170) at the voltage of 6 V.
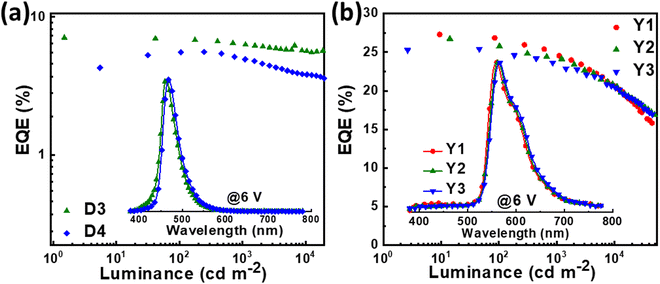 |
| Fig. 6 (a) EQE versus luminance curves of the blue OLEDs (D3 and D4). Insert: Normalized EL spectra at the voltage of 6 V. (b) EQE versus luminance curves of the yellow OLEDs (Y1, Y2 and Y3). Insert: Normalized EL spectra at the voltage of 6 V. | |
Due to the better EL performance of PIS, HSP OLEDs were further fabricated with PIS as a host for phosphor. Given the T1 of PIS, yellow phosphorescence emitter iridium(III) bis(4-phenylthieno[3,2-c]pyridinato-N,C2′)acetylacetonate (PO-01) with the T1 of 2.2 eV was selected as the dopant. The FET process should be also efficient from PIS to PO-01, due to the considerable overlap between the absorption spectrum of PO-01 and the emission spectrum of PIS. The optimized device structure is ITO/HATCN (20 nm)/TAPC (50 nm)/TCTA (5 nm)/PIS: XX wt% PO-01 (15 nm)/1,3,5-tri(phenyl-2-benzimidazolyl)-benzene (TPBi, 40 nm)/LiF (1 nm)/Al (100 nm), where the doping concentrations are 1, 5 and 10 wt% for the devices Y1, Y2 and Y3, respectively. The maximum forward-viewing CEs, PEs, and EQEs are 87.77 cd A−1, 98.43 lm W−1, and 27.30% for device Y1, 85.15 cd A−1, 95.51 lm W−1, and 26.73% for device Y2, 79.42 cd A−1, 95.91 lm W−1, and 25.41% for device Y3, respectively. Significantly, the best EL performance (in device Y1) is achieved when the low doping concentration of the phosphorescence emitter was merely 1 wt%, which differs from the traditional host-based devices that need a high dopant concentration of phosphors (5–20 wt%) for guaranteeing effective energy transfer from the host to the emitter.59–61 Such low phosphor concentration can reduce the high cost of PHOLEDs.62 In addition, the EQEs of device Y1 remain as high as 24.65% and 22.15% at the high luminance of 1000 and 5000 cd m−2. These results confirmed that HSP and HSF can be regarded as promising pathways for developing high efficiency and low roll-off OLEDs.
It is known that white light can be generated by integrating blue with yellow emission. In the HSP mechanism, blue emission from the PIS host and yellow emission from complementary phosphor can be realized simultaneously by managing the incomplete FET process from the host to phosphor (Fig. 7(a)). Based on this, a simplified hybrid warm-white OLED (W) with a single-emissive-layer of PIS: 0.5 wt% PO-01 (15 nm) was obtained. As shown in Fig. 7, device W achieves the highest forward-viewing CE, PE, and EQE of 85.85 cd A−1, 96.27 lm W−1, and 26.56%. At 1000 cd m−2, the EQE and PE still remain as high as 23.94% and 67.67 lm W−1, respectively. At a voltage of 6 V, the device shows the CIE coordinates of (0.459, 0.487), corresponding to the correlated color temperature of 3227 K. These results confirmed that HSP and HSF can be regarded as the promising pathways to develop high efficiency and low roll-off OLEDs.
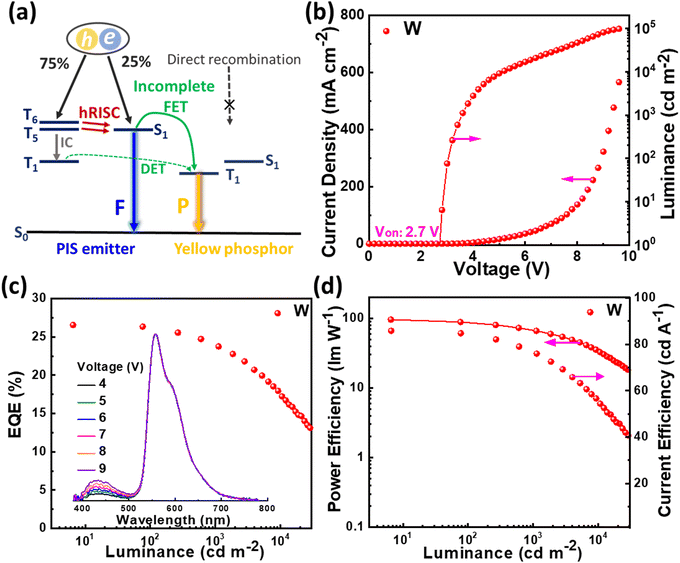 |
| Fig. 7 (a) Emission mechanism of hybrid white device W with PIS as the host and emitter. (b) Current–density–voltage–luminance characteristics of device W. (c) EQE versus luminance curves of device W. Insert: Normalized EL spectra at different applied voltages. (d) Power efficiency and current efficiency versus luminance curves of device W. | |
Conclusions
In conclusion, we have successfully developed two novel deep-blue HLCT emitters PIS and CNPIS by managing the anchoring groups at the PI unit. It turns out that cyano modification has an insignificant effect on emission colors but decelerates the hRISC process. Therefore, a higher EQE of 4.59% and CIE coordinates of (0.152, 0.067) are achieved in the non-doped device with PIS as an emitter. Based on this, HLCT-sensitized fluorescent and phosphorescent devices with PIS as a sensitizer not only realize high efficiencies of 7.08% and 27.30%, 9.38 and 98.43 lm W−1 for EQEs and PEs but also exhibit low efficiency roll-off (6.35% and 24.65% @1000 cd m−2), respectively. Furthermore, a hybrid white OLED with PIS as the emitter and host shows a PE and an EQE of 96.27 lm W−1 and 26.56%, respectively, and retains as high as 67.67 lm W−1 and 23.94% at the luminance of 1000 cd m−2. This work may open a new avenue to develop high efficiency and low roll-off OLEDs.
Conflicts of interest
There are no conflicts to declare.
Acknowledgements
This work was supported by grants from the National Natural Science Foundation of China (no. 52002804, 52103220, 52103017 and 22022501), the Shandong Provincial Natural Science Foundation (ZR2019ZD50) and the Open Fund of the State Key Laboratory of Luminescent Materials and Devices (2020-skllmd-12, South China University of Technology).
Notes and references
- C. W. Tang and S. A. VanSlyke, Organic electroluminescent diodes, Appl. Phys. Lett., 1987, 51, 913–915 CrossRef CAS.
- M. A. Baldo, D. F. O'Brien, Y. You, A. Shoustikov, S. Sibley, M. E. Thompson and S. R. Forrest, Highly efficient phosphorescent emission from organic electroluminescent devices, Nature, 1998, 395, 151–154 CrossRef CAS.
- H. Uoyama, K. Goushi, K. Shizu, H. Nomura and C. Adachi, Highly efficient organic light-emitting diodes from delayed fluorescence, Nature, 2012, 492, 234–238 CrossRef CAS PubMed.
- Y. Xu, P. Xu, D. Hu and Y. Ma, Recent progress in hot exciton materials for organic light-emitting diodes, Chem. Soc. Rev., 2021, 50, 1030–1069 RSC.
- C. Du, T. Lu, Z. Cheng, Y. Chang, H. Liu, J. Wang, L. Wan, Y. Lv and P. Lu, Rational molecular design of phenanthroimidazole-based fluorescent materials towards high-efficiency non-doped deep blue OLEDs, J. Mater. Chem. C, 2022, 10, 14186–14193 RSC.
- J. Zhao, B. Liu, Z. Wang, Q. Tong, X. Du, C. Zheng, H. Lin, S. Tao and X. Zhang, EQE climbing over 6% at high brightness of 14
350 cd m−2 in deep-blue OLEDs based on hybridized local and charge-transfer fluorescence, ACS Appl. Mater. Interfaces, 2018, 10, 9629–9637 CrossRef CAS.
- F. Liu, Z. Cheng, L. Wan, L. Gao, Z. Yan, D. Hu, L. Ying, P. Lu and Y. Ma, Anthracene-based emitters for highly efficient deep blue organic light-emitting diodes with narrow emission spectrum, Chem. Eng. J., 2021, 426, 131351 CrossRef CAS.
- H. L. Lee, S. O. Jeon, I. Kim, S. C. Kim, J. Lim, J. Kim, S. Park, J. Chwae, W.-J. Son, H. Choi and J. Y. Lee, Multiple-resonance extension and spin-vibronic-coupling-based narrowband blue organic fluorescence emitters with over 30% quantum efficiency, Adv. Mater., 2022, 34, 2202464 CrossRef CAS.
- F. Huang, K. Wang, Y.-Z. Shi, X.-C. Fan, X. Zhang, J. Yu, C.-S. Lee and X.-H. Zhang, Approaching efficient and narrow RGB electroluminescence from D–A-type TADF emitters containing an identical multiple resonance backbone as the acceptor, ACS Appl. Mater. Interfaces, 2021, 13, 36089–36097 CrossRef CAS.
- X. Cai, J. Xue, C. Li, B. Liang, A. Ying, Y. Tan, S. Gong and Y. Wang, Achieving 37.1% green electroluminescent efficiency and 0.09 ev full width at half maximum based on a ternary boron-oxygen-nitrogen embedded polycyclic aromatic system, Angew. Chem. Int. Ed., 2022, 61, e202200337 CAS.
- F. Liu, Z. Cheng, L. Wan, Z. Feng, H. Liu, H. Jin, L. Gao, P. Lu and W. Yang, Highly efficient multi-resonance thermally activated delayed fluorescence material with a narrow full width at half-maximum of 0.14 eV, Small, 2022, 18, 2106462 CrossRef CAS PubMed.
- Y. Chen, J. Zhu, Y. Wu, J. Yao, D. Yang, X. Qiao, Y. Dai, Q. Tong and D. Ma, Highly efficient fluorescence/phosphorescence hybrid white organic light-emitting devices based on a bipolar blue emitter to precisely control charges and excitons, J. Mater. Chem. C, 2020, 8, 7543–7551 RSC.
- S. Ying, W. Liu, L. Peng, Y. Dai, D. Yang, X. Qiao, J. Chen, L. Wang and D. Ma, A Promising multifunctional deep-blue fluorophor for high-performance monochromatic and hybrid white OLEDs with superior efficiency/color stability and low efficiency roll-off, Adv. Opt. Mater., 2022, 10, 2101920 CrossRef CAS.
- S.-C. Dong, L. Zhang, J. Liang, L.-S. Cui, Q. Li, Z.-Q. Jiang and L.-S. Liao, Rational design of dibenzothiophene-based host materials for PHOLEDs, J. Phys. Chem. C, 2014, 118, 2375–2384 CrossRef CAS.
- R. Huang, N. A. Kukhta, J. S. Ward, A. Danos, A. S. Batsanov, M. R. Bryce and F. B. Dias, Balancing charge-transfer strength and triplet states for deep-blue thermally activated delayed fluorescence with an unconventional electron rich dibenzothiophene acceptor, J. Mater. Chem. C, 2019, 7, 13224–13234 RSC.
- X.-Y. Liu, F. Liang, L.-S. Cui, X.-D. Yuan, Z.-Q. Jiang and L.-S. Liao, Effective host materials for blue/white organic light-emitting diodes by utilizing the twisted conjugation structure in 10-phenyl-9,10-dihydroacridine block, Chem. – Asian J., 2015, 10, 1402–1409 CrossRef CAS.
- Y.-J. Na, W. Song, J. Y. Lee and S.-H. Hwang, Synthesis of dibenzothiophene-based host materials and their blue phosphorescent device performances, Org. Electron., 2015, 22, 92–97 CrossRef CAS.
- C. W. Lee and J. Y. Lee, Structure-property relationship of pyridoindole-type host materials for high-efficiency blue phosphorescent organic light-emitting diodes, Chem. Mater., 2014, 26, 1616–1621 CrossRef CAS.
- J. H. Kim, S. H. Han and J. Y. Lee, Dibenzothiophene derived hosts with CN substituted carbazole for blue thermally activated delayed fluorescent organic light-emitting diodes, Synth. Met., 2017, 232, 152–158 CrossRef CAS.
- L. Ding, W.-Z. Fo, X.-Y. Liu, H. Chen, F. Igbari, J.-H. Wu and Z.-Q. Jiang, Highly efficient green phosphorescent organic light-emitting diodes based on tetraphenyl silicon derivative host materials, Org. Electron., 2020, 78, 105581 CrossRef CAS.
- S.-C. Dong, C.-H. Gao, X.-D. Yuan, L.-S. Cui, Z.-Q. Jiang, S.-T. Lee and L.-S. Liao, Novel dibenzothiophene based host materials incorporating spirobifluorene for high-efficiency white phosphorescent organic light-emitting diodes, Org. Electron., 2013, 14, 902–908 CrossRef CAS.
- S. H. Jeong and J. Y. Lee, Dibenzothiophene derivatives as host materials for high efficiency in deep blue phosphorescent organic light emitting diodes, J. Mater. Chem., 2011, 21, 14604–14609 RSC.
- L. Ding, J.-N. Wang, X.-Y. Liu, H. Chen, F. Igbari and L.-S. Liao, Dibenzothiophene, dibenzofuran and pyridine substituted tetraphenyl silicon derivatives hosts for green phosphorescent organic light-emitting diodes, Org. Electron., 2019, 71, 258–265 CrossRef CAS.
- S. K. Shin, S. Y. Byeon, S. H. Han and J. Y. Lee, novel host materials based on dibenzothiophene and carbazolylcarbazole for extended lifetime in blue phosphorescent organic light-emitting diodes, Adv. Opt. Mater., 2018, 6, 1701007 CrossRef.
- C. Han, Z. Zhang, H. Xu, S. Yue, J. Li, P. Yan, Z. Deng, Y. Zhao, P. Yan and S. Liu, Short-axis substitution approach selectively optimizes electrical properties of dibenzothiophene-based phosphine oxide hosts, J. Am. Chem. Soc., 2012, 134, 19179–19188 CrossRef CAS PubMed.
- S. Y. Byeon, S. H. Han and J. Y. Lee, Carbazole-dibenzothiophene core as a building block of host materials for blue phosphorescent organic light-emitting diodes, Dyes Pigm., 2018, 155, 114–120 CrossRef CAS.
- F. Wang, J. Sun, M. Liu, H. Shi, H. Ma, W. Ye, H. Wang, H. Zhang, Z. An and W. Huang, D–A–D-type bipolar host materials with room temperature phosphorescence for high-efficiency green phosphorescent organic light-emitting diodes, J. Mater. Chem. C, 2020, 8, 1871–1878 RSC.
- Y. J. Kang and J. Y. Lee, P-170: Synthesis and device performances of high triplet energy electron transport materials, Dig. Tech. Pap. – Soc. Inf. Disp. Int. Symp., 2016, 47, 1757–1759 CrossRef CAS.
- H. Fukagawa, T. Shimizu, H. Kawano, S. Yui, T. Shinnai, A. Iwai, K. Tsuchiya and T. Yamamoto, Novel Hole-transporting materials with high triplet energy for highly efficient and stable organic light-emitting diodes, J. Phys. Chem. C, 2016, 120, 18748–18755 CrossRef CAS.
- H. Fukagawa, T. Shimizu, Y. Kiribayashi, Y. Osada, T. Kamada, T. Yamamoto, N. Shimidzu and T. Kurita, Molecular design of hole-transporting material for efficient and stable green phosphorescent organic light-emitting diodes, Appl. Phys. Lett., 2013, 103, 143306 CrossRef.
- S. Chen, Y. Wu, Y. Zhao and D. Fang, Deep blue organic light-emitting devices enabled by bipolar phenanthro[9,10-d]imidazole derivatives, RSC Adv., 2015, 5, 72009–72018 RSC.
- J. Ye, Y. Gao, L. He, T. Tan, W. Chen, Y. Liu, Y. Wang and G. Ning, Efficient blue-emitting molecules by incorporating sulfur-containing moieties into triarylcyclopentadiene: Synthesis, crystal structures and photophysical properties, Dyes Pigm., 2016, 124, 145–155 CrossRef CAS.
- J. Guo, X.-L. Li, H. Nie, W. Luo, S. Gan, S. Hu, R. Hu, A. Qin, Z. Zhao, S.-J. Su and B. Z. Tang, Achieving high-performance nondoped OLEDs with extremely small efficiency roll-off by combining aggregation-induced emission and thermally activated delayed fluorescence, Adv. Funct. Mater., 2017, 27, 1606458 CrossRef.
- J. Guo, X.-L. Li, H. Nie, W. Luo, R. Hu, A. Qin, Z. Zhao, S.-J. Su and B. Z. Tang, Robust luminescent materials with prominent aggregation-induced emission and thermally activated delayed fluorescence for high-performance organic light-emitting diodes, Chem. Mater., 2017, 29, 3623–3631 CrossRef CAS.
- J. Tang, Y. Sun, Q. Tang and Y. Sun, Molecular engineering of A–D–D–A dual-emitting-cores emitters with thermally activated delayed fluorescence and aggregation-induced emission characteristics, Org. Electron., 2021, 96, 106199 CrossRef CAS.
- C. Xia, X. Wang, J. Lin, W. Jiang, Y. Ni and W. Huang, Organic light-emitting devices (OLED) based on new triphenylamine derivatives, Synth. Met., 2009, 159, 194–200 CrossRef CAS.
- Y. Sim, H. Lee, J. Park, D. Shin, M. Park and K.-Y. Kay, Synthesis and electroluminescence property of pyrene derivatives including dibenzothiophene and imidazole moiety, J. Nanosci. Nanotechnol., 2019, 19, 4710–4714 CrossRef CAS PubMed.
- K. H. Lee, J. H. Seo, Y. K. Kim and S. S. Yoon,
N,N-(Diphenylamino)fluorenylstyrene derivatives with the various heteroatom-containing moieties for blue organic light-emitting diodes, J. Nanosci. Nanotechnol., 2013, 13, 1808–1811 CrossRef CAS.
- L. Yao, S. Sun, S. Xue, S. Zhang, X. Wu, H. Zhang, Y. Pan, C. Gu, F. Li and Y. Ma, Aromatic S-heterocycle and fluorene derivatives as solution-processed blue fluorescent emitters: Structure-property relationships for different sulfur oxidation states, J. Phys. Chem. C, 2013, 117, 14189–14196 CrossRef CAS.
- X. Tang, T. Shan, Q. Bai, H. Ma, X. He and P. Lu, Efficient deep-blue electroluminescence based on phenanthroimidazole-dibenzothiophene derivatives with different oxidation states of the sulfur atom, Chem. – Asian J., 2017, 12, 552–560 CrossRef CAS.
- X. Tang, Q. Bai, T. Shan, J. Li, Y. Gao, F. Liu, H. Liu, Q. Peng, B. Yang, F. Li and P. Lu, Efficient nondoped blue fluorescent organic light-emitting diodes (OLEDs) with a high external quantum efficiency of 9.4% @ 1000 cd m−2 based on phenanthroimidazole-anthracene derivative, Adv. Funct. Mater., 2018, 28, 1705813 CrossRef.
- S. Zhang, L. Yao, Q. Peng, W. Li, Y. Pan, R. Xiao, Y. Gao, C. Gu, Z. Wang, P. Lu, F. Li, S. Su, B. Yang and Y. Ma, Achieving a significantly increased efficiency in nondoped pure blue fluorescent OLED: A quasi-equivalent hybridized excited state, Adv. Funct. Mater., 2015, 25, 1755–1762 CrossRef CAS.
- S. Ying, J. Lv, Y. Li, Y. Huo, Y. Liu, D. Ma, L. Peng and S. Yan, A large-scale deep-blue tetraphenylbenzene-bridged hybridized local and charge transfer fluorophore exhibiting small efficiency roll-off and low amplified spontaneous emission threshold, Mater. Chem. Front., 2022, 6, 2085–2094 RSC.
- Z. Gao, Z. Wang, T. Shan, Y. Liu, F. Shen, Y. Pan, H. Zhang, X. He, P. Lu, B. Yang and Y. Ma, High-efficiency deep blue fluorescent emitters based on phenanthro 9,10-d imidazole substituted carbazole and their applications in organic light emitting diodes, Org. Electron., 2014, 15, 2667–2676 CrossRef CAS.
- K. A. Zachariasse, T. v d Haar, A. Hebecker, U. Leinhos and W. Kuhnle, Intramolecular charge transfer in aminobenzonitriles: Requirements for dual fluorescence, Pure Appl. Chem., 1993, 65, 1745–1750 CrossRef CAS.
- H. Zhou, R. Wang, S. T. Zhang, W. Cui, S. Ying, Q. Sun, B. Yang, S. Xue, W. Yang and Y. Ma, Highly efficient blue-emissive electroluminescence: Nondestructive color regulation effect of orthogonal cyano-substitution in hybrid locally-excited and charge-transfer (HLCT) backbone emitters, Mater. Today Chem., 2022, 24, 100785 CrossRef CAS.
- J. Chen, H. Liu, J. Guo, J. Wang, N. Qiu, S. Xiao, J. Chi, D. Yang, D. Ma, Z. Zhao and B. Z. Tang, Robust Luminescent molecules with high-level reverse intersystem crossing for efficient near ultraviolet organic light-emitting diodes, Angew. Chem., Int. Ed., 2022, 61, e202116810 CAS.
- Y. Liu, X. Man, Q. Bai, H. Liu, P. Liu, Y. Fu, D. Hu, P. Lu and Y. Ma, Highly efficient blue organic light-emitting diode based on a pyrene[4,5-d]imidazole-pyrene molecule, CCS Chem., 2022, 4, 214–227 CrossRef CAS.
- S. Xiao, S.-T. Zhang, Y. Gao, X. Yang, H. Liu, W. Li and B. Yang, Efficient and stable deep-blue narrow-spectrum electroluminescence based on hybridized local and charge-transfer (HLCT) state, Dyes Pigm., 2021, 193, 109482 CrossRef CAS.
- H. Zhang, B. Zhang, Y. Zhang, Z. Xu, H. Wu, P.-A. Yin, Z. Wang, Z. Zhao, D. Ma and B. Z. Tang, A Multifunctional blue-emitting material designed via tuning distribution of hybridized excited-state for high-performance blue and host-sensitized OLEDs, Adv. Funct. Mater., 2020, 30, 2002323 CrossRef CAS.
- H. Zhang, J. Zeng, W. Luo, H. Wu, C. Zeng, K. Zhang, W. Feng, Z. Wang, Z. Zhao and B. Z. Tang, Synergistic tuning of the optical and electrical performance of AIEgens with a hybridized local and charge-transfer excited state, J. Mater. Chem. C, 2019, 7, 6359–6368 RSC.
- J. U. Kim, I. S. Park, C.-Y. Chan, M. Tanaka, Y. Tsuchiya, H. Nakanotani and C. Adachi, Nanosecond-time-scale delayed fluorescence molecule for deep-blue OLEDs with small efficiency rolloff, Nat. Commun., 2020, 11, 1765 CrossRef CAS.
- M. A. El-Sayed, Spin–orbit coupling and the radiationless processes in nitrogen heterocyclics, J. Chem. Phys., 1963, 38, 2834–2838 CrossRef CAS.
- R. Chen, Y. Tang, Y. Wan, T. Chen, C. Zheng, Y. Qi, Y. Cheng and W. Huang, Promoting singlet/triplet exciton transformation in organic optoelectronic molecules: Role of excited state transition configuration, Sci. Rep., 2017, 7, 6225 CrossRef.
- M. Cai, D. Zhang and L. Duan, High Performance thermally activated delayed fluorescence sensitized organic light-emitting diodes, Chem. Rec., 2019, 19, 1611–1623 CrossRef CAS.
- D. Zhang, L. Duan, C. Li, Y. Li, H. Li, D. Zhang and Y. Qiu, High-efficiency fluorescent organic light-emitting devices using sensitizing hosts with a small singlet–triplet exchange energy, Adv. Mater., 2014, 26, 5050–5055 CrossRef CAS PubMed.
- D. Zhang, L. Duan, D. Zhang, J. Qiao, G. Dong, L. Wang and Y. Qiu, Extremely low driving voltage electrophosphorescent green organic light-emitting diodes based on a host material with small singlet–triplet exchange energy without p- or n-doping layer, Org. Electron., 2013, 14, 260–266 CrossRef CAS.
- H. Lim, H. J. Cheon, G. S. Lee, M. Kim, Y.-H. Kim and J.-J. Kim, Enhanced triplet–triplet annihilation of blue fluorescent organic light-emitting diodes by generating excitons in trapped charge-free regions, ACS Appl. Mater. Interfaces, 2019, 11, 48121–48127 CrossRef CAS PubMed.
- H. Sasabe, H. Nakanishi, Y. Watanabe, S. Yano, M. Hirasawa, Y.-J. Pu and J. Kido, Extremely low operating voltage green phosphorescent organic light-emitting devices, Adv. Funct. Mater., 2013, 23, 5550–5555 CrossRef CAS.
- H. Liu, Q. Bai, L. Yao, D. Hu, X. Tang, F. Shen, H. Zhang, Y. Gao, P. Lu, B. Yang and Y. Ma, Solution-processable hosts constructed by carbazole/PO substituted tetraphenylsilanes for efficient blue electrophosphorescent devices, Adv. Funct. Mater., 2014, 24, 5881–5888 CrossRef CAS.
- X.-D. Yuan, J. Liang, Y.-C. He, Q. Li, C. Zhong, Z.-Q. Jiang and L.-S. Liao, A rational design of carbazole-based host materials with suitable spacer group towards highly-efficient blue phosphorescence, J. Mater. Chem. C, 2014, 2, 6387–6394 RSC.
- D. Zhang, L. Duan, D. Zhang and Y. Qiu, Towards ideal electrophosphorescent devices with low dopant concentrations: The key role of triplet up-conversion, J. Mater. Chem. C, 2014, 2, 8983–8989 RSC.
|
This journal is © the Partner Organisations 2023 |
Click here to see how this site uses Cookies. View our privacy policy here.