DOI:
10.1039/D2RA00293K
(Paper)
RSC Adv., 2022,
12, 6876-6880
A novel AIE-active imidazolium macrocyclic ratiometric fluorescence sensor for pyrophosphate anion†
Received
15th January 2022
, Accepted 22nd February 2022
First published on 1st March 2022
Abstract
An imidazolium bridged macrocyclophane was synthesized as a ratiometric fluorescence sensor with aggregation-induced emission (AIE) characteristic to detect pyrophosphate anion with high selectivity among various anions. In the presence of zinc ion, macrocyclophane can form aggregates through complexation with pyrophosphate anion and emit ratiometric fluorescence, resulting from an enhancement in its aggregate-state emission and a reduction in its monomer emission. This AIE-active macrocycle showed great potential as a ratiometric fluorescence receptor.
Introduction
Anions play very important roles in the environment and our health. Among them, phosphate anions have attracted considerable interest owing to their ubiquitous nature in biological systems and strong impact on life processes.1 They participate in energy transduction and metabolic processes.2 Because the concomitant release of pyrophosphate anions during ATP hydrolysis occurs in many relevant biological processes, thus, these anions are considered important targets for monitoring such processes.3 Several diseases, such as arthritis and Mönckeberg's arteriosclerosis, are related to abnormal levels of phosphate anions in the blood serum.4 Up to now, much research effort has been devoted to develop highly selective and sensitive sensors for phosphate anions through coordination chemistry.5 Several techniques, such as colorimetry6 and electrochemiluminescence,7 have been established to detect phosphate anions. Fluorescence-based receptors, in particular, have attracted widespread attention due to their high sensitivity and easy operation in both in vivo and in vitro imaging.8 Although several chemosensors have been reported for phosphate-anion sensing.9–12 However, great difficulties remains to develop highly selective and sensitive fluorescence sensors for phosphate anions especially in aqueous environment because of disrupting of binding of solvents and competitions from other similar anions. An effective strategy to overcome those challenges is to develop chemical sensors that have multiple binding units preorganized for specific targets of interest. Macrocycles are well suited for such purposes. In the past, macrocyclic based host materials have been used for sensing,13 encapsulations14 and self-assemblies.15 Therefore, macrocyclic chemical sensors have great potential for further developing highly selective and sensitive fluorescent probes for various anions. Nevertheless, this type of fluorescence sensors remains scares.
Aggregation-induced emission (AIE) is a unique phenomenon that strong fluorescence arouses in the aggregated state which usually quenches fluorescence.16 This unusual behavior of fluorophores makes them very good candidates for simple fluorescence turn-on or turn-off sensors either induced by aggregation of an analyte or by quenching the aggregate emission with a quencher.17 This type of sensors based on AIEgens has gained great success. With this type of design, false-positive responses can significantly weaken the confidence of detection. A possible way to overcome this problem is by using ratiometric probes.18 This type of design is less likely to produce false-positive responses because it is based on the relative intensities at two different wavelengths. Successful developments of ratiometric fluorescence sensors have been found for some specific analytes.19 Ratiometric probes based on AIEgens are very rare because most AIEgens don't display two emissions simultaneously. In a previous study, a novel imidazolium macrocycle was reported to display two emissions simultaneously.20 Here, we take advantage of this unusual dual emission of this macrocycle and used it as a ratiometric probe to detect pyrophosphate anions in aqueous solution. In the presence of zinc ion, this macrocyclophane showed high selectivity for pyrophosphate anion and showed ratiometric fluorescence changes of enhanced aggregate emission and reduced monomer emission.
Results and discussion
As shown in Scheme S1,† the TPE derivative 4 was synthesized by using 4,4′-dimethoxybenzophenone and 9-fluorenone as a starting material and it was easily transformed into dialdehyde 5 through a Duff reaction. With dialdehyde 5 in hand, dialcohol 6 and dichloride 7 were obtained by reduction with NaBH4 followed by a chlorinating reaction with thionyl chloride. Upon a nucleophilic substitution with imidazole, dichloride 7 was converted into diimidazole 8. Finally, the target product imidazolium macrocycle 1 was obtained in 76% yield by the reaction of dichloride 7 and diimidazole 8 in the presence of a tetrabutylammonium chloride template (Scheme 1).
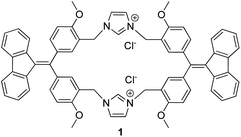 |
| Scheme 1 The chemical structure of macrocyclophane 1. | |
The evolution of the emission spectra of 1 in response to different solvent systems was assessed in DMSO/toluene, CHCl3/hexane, and DMSO/H2O (Fig. S9–S11†). Unlike typical AIEgens that are non-emissive in the soluble state, macrocyclophane 1 showed distinct monomer emission at wavelength of 450 nm in the soluble state. Upon aggregation of 1 with increasing the ration of the poor solvent, the aggregate-state emission became the major emission at 550 nm. The fluorescence intensity of monomer-to-aggregate emission could be tuned by changing the ratio of the solvents. This result encouraged us to apply 1 as a ratiometric probe by changing the aggregation degree via addition of suitable analyte.
The imidazolium motif has been reported as a receptor for a number of anions.6,11 This finding prompted us to examine the sensing performance of 1 toward different anions. Firstly, we added one aliquot of different anions to the solution of 1 in DMSO and water mixture (v/v 50/50).21 Phosphate (Pi) and pyrophosphate (PPi) resulted in a red emission, however, the responsiveness and selectivity observed were discouraging (Fig. S12†). Then one aliquot of zinc ion was added to the solution of 1 and various anions. Surprisingly, only pyrophosphate anions demonstrated a distinct enhancement in their aggregate-state emission as indicated in Fig. 1A. Other anions, such as phosphate and monohydrogen phosphate anions, showed slight enhancements in their emission intensity. The addition of zinc ion to other anions, it had a minimal effect on the emission spectra (Fig. 1B). Further experimentation by titrating pyrophosphate anion into a solution of 1·Zn showed that the emission intensity at 555 nm progressively increased upon addition of the anion solution (Fig. 2). When the concentration increased to 1 × 10−4 M, the fluorescence intensity had no further obvious change, indicating 1·Zn and pyrophosphate anion complexed in a 1
:
1 molar ratio.
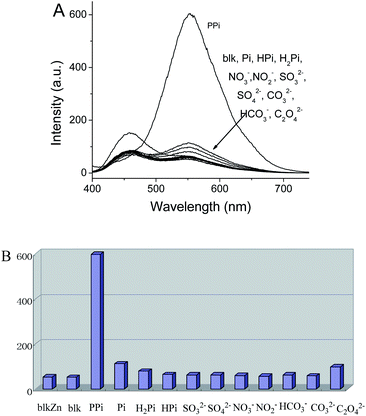 |
| Fig. 1 (A) The fluorescence spectra of 1 in water containing 50% DMSO with addition of different anions in the presence of zinc(II). λex = 378 nm, ex/em slits = 5/10 nm. (B) Diagram of the emission at 550 nm of 1 in the presence of zinc(II) and different anions. [1] = [Zn(OAc)2] = 1/2[anion] = 5.0 × 10−5 M. | |
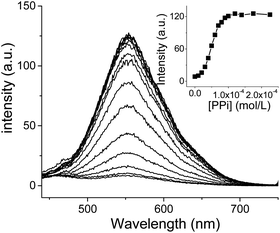 |
| Fig. 2 The fluorescence change of 1 (50 μM) in water containing 50% DMSO with PPi in the presence of 1 equivalent zinc(II). Inset, curve of the intensity at 550 nm vs. the concentration of PPi. λex = 378 nm, ex/em slits = 5/5 nm. | |
After confirming that aggregation could be induced by the addition of pyrophosphate anion in the presence of zinc ion, we then investigated the feasibility of achieving a ratiometric response toward pyrophosphate anion. The selectivity of the resultant sensor was tested, and the results are shown in Fig. 3. Similar to above-mentioned experiments, only pyrophosphate anion showed obvious fluorescence increase. The aggregate-state emission of the anion at 550 nm greatly increased whereas their monomer emission at 443 nm slightly decreased. Meanwhile, other anions showed tiny changes in their emission spectra. The ratiometric change of fluorescence enabled by probe 1 is distinctly different with a similar sensor9c reported before that only simple enhancement of emission is possible.
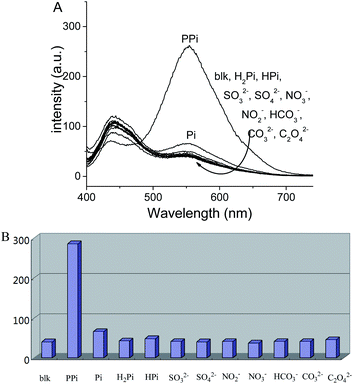 |
| Fig. 3 (A) The fluorescence spectra of 1 in water containing 50% DMSO with addition of different anions in the presence of zinc(II). λex = 378 nm, ex/em slits = 5/10 nm. (B) Diagram of the emission at 472 nm of 1 in the presence of zinc(II) and different anions. [1] = [Zn(OAc)2] = 1/2[anion] = 1.0 × 10−5 M. | |
Then, a fluorescent titration experiment was conducted by using pyrophosphate anion as the analyte. As shown in Fig. 4A, changes in the intensity of the monomer and aggregate-state emissions were observed. Specifically, the aggregate emission progressively increased whereas the monomer emission decreased. A clear isomeric point was noted at about 490 nm. Moreover, UV-vis titration of 1·Zn and pyrophosphate anion was also implemented. With increasing of concentration of PPi, the absorption intensity at 253 nm gradually (Fig. 4B). When the concentration of PPi increased to 1 × 10−4 M, the absorption spectra showed little changes, which is consistent with the result of fluorescence titration. The detection limit of ppi with probe 1 was estimated as 67 nM which is among the range of best similar probes. (ESI, Table S1†).
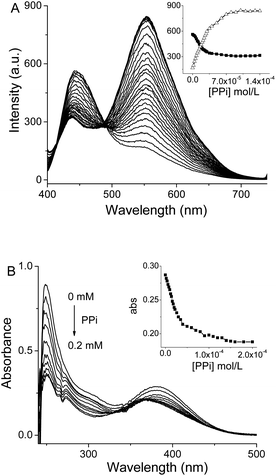 |
| Fig. 4 (A) The fluorescence change of 1 in water containing 50% DMSO with PPi in the presence of zinc(II). Inset, curve of the intensity at 550 nm vs. the concentration of PPi. λex = 378 nm, ex/em slits = 10/10 nm. (B) Change of the absorption spectra of 1 in water containing 50% DMSO with PPi. Inset, curve of the absorbance at 253 nm vs. the concentration of PPi. [1] = [Zn(OAc)2] = 1.0 × 10−5 M. | |
The binding affinity of macrocycle 1 with pyrophosphate anion was obtained by fitting the UV-Vis titration traces at 253 nm using 1
:
1 binding model (Fig. S13†). The binding constant was found to be 4.3 × 105 M−1 which indicates strong binding with PPI anion. The associated complex with PPi anion can also be detected by ESI+ mass spectroscopy (Fig. S14†). A mass peak at m/z 1209.2904 (z = 1) matched with the formation of 1
:
1 complex of 1 and PPi. The inclusion complex of 1-PPi alone in aqueous solution does not show significant changes in solubility. However, in the presence of Zn(II), the inclusion complex of 1-PPi was further connected possibly through the coordination of zinc cation with pyrophosphate anions. It is through this coordination, the solubility of the complex changed and aggregates formed. The size of the aggregation in aqueous solution was found to be around 2.5 μm by dynamic light scattering (DLS) measurement (Fig. S15†). Therefore, a possible mechanism of the selective sensing of pyrophosphate anion can be drawn as below. The macrocycle, which bearing two imidazolium units, is able to binding a pyrophosphate anion into its cavity possibly through electrostatic and multiple dipole–dipole interactions. The inclusion complex of 1-PPi can coordinated with Zn2+ which results in aggregation and hence changes the fluorescence from monomer emission to aggregate emission that gives a ratiometric fluorescence change (more discussion of mechanism see ESI Note 1†).
Conclusions
In conclusion, we have used an AIE-active macrocyclophane bearing imidazolium linkers as a selective ratiometric fluorescence sensor for pyrophosphate anion in aqueous solution in the presence of zinc cation. A selective binding pocket for pyrophosphate anion is provided by the imidazolium macrocycle with its well suited size and cooperative binding forces. Through coordination with zinc cation, ratiometric fluorescence change of this system offers a unique example among AIEgens based sensors. The present study provides a new strategy for development of ratiometric fluorescence sensors based on AIEgens. Further developments of ratiometric sensors using this strategy for various targets such as other anions, environment pollutants and health hazards are pursued in our labs and will be reported in due course.
Conflicts of interest
There are no conflicts to declare.
Acknowledgements
This work was financially supported by the National Natural Science Foundation of China (No. 21901264 and 21902189), Project funded by China Postdoctoral Science Foundation (2021M692907), Young Backbone Teacher of Zhongyuan University of Technology (2020XQG10, 2020XQG09), the Natural Science Basic Research Plan in Shaanxi Province of China (2019JQ-302 and 2021JQ-801) and the Research Foundation of Education Department of Shaanxi Province (18JS009).
Notes and references
-
(a) F. H. Westheimer, Science, 1987, 235, 1173–1178 CrossRef CAS PubMed;
(b) T. Berndt and R. Kumar, Physiology, 2009, 24, 17–25 CrossRef CAS PubMed.
-
(a) W. N. Lipscomb and N. Strater, Chem. Rev., 1996, 96, 2375 CrossRef CAS PubMed;
(b) M. J. Ryle and L. C. Seefeldt, J. Biol. Chem., 2000, 275, 6214 CrossRef CAS PubMed.
- M. Ronaghi, S. Karamohamed, B. Petterson, M. Uhlem and P. Nyren, Anal. Biochem., 1996, 242, 84 CrossRef CAS PubMed.
-
(a) A. I. Al-Absi, B. M. Wall and C. R. Cooke, Am. J. Kidney Dis., 2004, 44, 37 CrossRef;
(b) C. E. B. Couri, G. A. da Silva, J. A. B. Martinez, F. D. A. Pereira and F. J. A. de Paula, Cardiovasc. Disor., 2005, 5, 34 CrossRef PubMed.
-
(a) S. Bhowmik, B. N. Ghosh, V. Marjomäki and K. Rissanen, J. Am. Chem. Soc., 2014, 136, 5543–5546 CrossRef CAS PubMed;
(b) G. Sanchez, A. Espinosa, D. Curiel, A. Tarraga and P. Molina, J. Org. Chem., 2013, 78(19), 9725–9737 CrossRef CAS PubMed.
-
(a) X. Liu, H. T. Ngo, Z. Ge, S. J. Butler and K. A. Jolliffe, Chem. Sci., 2013, 4, 1680–1686 RSC;
(b) C. R. Lohani, J. M. Kim, S. Y. Chung, J. Yoon and K. H. Lee, Analyst, 2010, 135, 2079–2084 RSC;
(c) E. Climent, R. Casasus, M. D. Marcos, R. Martinez-Manez, F. Sancenon and J. Soto, Dalton Trans., 2009, 4806–4814 RSC;
(d) M. S. Han and D. H. Kim, Angew. Chem., 2002, 114, 3963–3965 CrossRef;
(e) Y. J. Jang, E. J. Jun, Y. J. Lee, Y. S. Kim, J. S. Kim and J. Yoon, J. Org. Chem., 2005, 70, 9603–9606 CrossRef CAS PubMed;
(f) X. Huang, Z. Guo, W. Zhu, Y. Xie and H. Tian, Chem. Commun., 2008, 5143–5145 RSC.
-
(a) I. S. Shin, S. W. Bae, H. Kim and J. I. Hong, Anal. Chem., 2010, 82, 8259–8265 CrossRef CAS PubMed;
(b) P. Anzenbacher, M. A. Palacios, K. Jursíková and M. Marquez, Org. Lett., 2005, 7, 5027–5030 CrossRef CAS PubMed.
-
(a) D. H. Lee, S. Y. Kim and J. I. Hong, Angew. Chem., Int. Ed., 2004, 43, 4777 CrossRef CAS PubMed;
(b) S. Anbu, S. Kamalraj, C. Jayabaskaran and P. S. Mukherjee, Inorg. Chem., 2013, 52, 8294 CrossRef CAS PubMed;
(c) C. Li, M. Numata, M. Takeuchi and S. Shinkai, Angew. Chem., Int. Ed., 2005, 44, 6371 CrossRef CAS PubMed;
(d) M. Schäferling, Angew. Chem., Int. Ed., 2012, 51, 3532 CrossRef PubMed.
-
(a) K. M. K. Swamy, S. K. Kwon, H. N. Lee, S. M. Shantha Kumar, J. S. Kim and J. Yoon, Tetrahedron Lett., 2007, 48, 8683–8686 CrossRef CAS;
(b) Y. X. Yuan, J. H. Wang and Y. S. Zheng, Chem.–Asian J., 2019, 14, 760–764 CrossRef CAS PubMed;
(c) J. H. Wang, J. B. Xiong, X. Zhang, S. Song, Z. H. Zhu and Y. S. Zheng, RSC Adv., 2015, 5, 60096–60100 RSC.
-
(a) J. F. Zhang, S. Kim, J. H. Han, S. J. Lee, T. Pradhan, Q. Y. Cao, S. J. Lee, C. Kang and J. S. Kim, Org. Lett., 2011, 13, 5294–5297 CrossRef CAS PubMed;
(b) S. Bhowmik, B. N. Ghosh, V. Marjomäki and K. Rissanen, J. Am. Chem. Soc., 2014, 136, 5543–5546 CrossRef CAS PubMed;
(c) H. N. Lee, K. M. K. Swamy, S. K. Kim, J. Y. Kwon, Y. Kim, S. J. Kim, Y. J. Yoon and J. Yoon, Org. Lett., 2006, 9, 243–246 CrossRef PubMed.
-
(a) J. Gao, T. Riis-Johannessen, R. Scopelliti, X. Qian and K. Severin, Dalton Trans., 2010, 39, 7114–7118 RSC;
(b) V. Suresh, N. Ahmed, I. S. Youn and K. S. Kim, Chem.–Asian J., 2012, 7, 658–663 CrossRef CAS PubMed;
(c) N. Ahmed, B. Shirinfar, I. S. Youn, M. Yousuf and K. S. Kim, Org. Biomol. Chem., 2013, 11, 6407–6413 RSC;
(d) N. Ahmed, B. Shirinfar, I. Geronimo and K. S. Kim, Org. Lett., 2011, 13, 5476–5479 CrossRef CAS PubMed;
(e) L. X. Huang, H. Y. Bai, H. Tao, G. Cheng and Q. Y. Cao, Dyes Pigm., 2020, 181, 108553 CrossRef CAS;
(f) L. He, R. P. Zuo, B. Wei, H. Tao and Q. Y. Cao, Dyes Pigm., 2019, 168, 205–211 CrossRef CAS;
(g) H. Tao, L. He, G. Cheng and Q. Y. Cao, Dyes Pigm., 2019, 166, 233–238 CrossRef CAS;
(h) C. T. Li, Y. L. Xu, J. G. Yang, Y. Chen, H. S. Kim, Q. Y. Cao and J. S. Kim, Sens. Actuators, B, 2017, 251, 617–623 CrossRef CAS;
(i) J. H. Zhu, C. Yu, Y. Chen, J. Shin, Q. Y. Cao and J. S. Kim, Chem. Comm., 2017, 53, 4342–4345 RSC.
-
(a) K. H. Chen, J. H. Liao, H. Y. Chan and J. M. Fang, J. Org. Chem., 2009, 74, 895–898 CrossRef CAS PubMed;
(b) T. Gunnlaugsson, A. P. Davis, J. E. O'Brien and M. Glynn, Org. Lett., 2002, 4, 2449–2452 CrossRef CAS PubMed;
(c) S. K. Kim, N. J. Singh, S. J. Kim, H. G. Kim, J. K. Kim, J. W. Lee, K. S. Kim and J. Yoon, Org. Lett., 2003, 5, 2083–2086 CrossRef CAS PubMed;
(d) T. Romero, A. Caballero, A. Tárraga and P. Molina, Org. Lett., 2009, 11, 3466–3469 CrossRef CAS PubMed.
-
(a) H. M. Tay and P. Beer, Org. Biomol. Chem., 2021, 19, 4652 RSC;
(b) W. B. Chen, P. Chen, G. Zhang, G. L. Xing, Y. Feng, Y. W. Yang and L. Chen, Chem. Soc. Rev., 2021, 50, 11684 RSC.
-
(a) M. J. W. R. Langer, Chem. Soc. Rev., 2017, 46, 6600 RSC;
(b) Z. C. Liu, S. K. M. Nalluri and J. F. Stoddart, Chem. Soc. Rev., 2017, 46, 2459–2478 RSC.
-
(a) K. Velmurugan, M. Mohan, B. Li, K. Wang, M. Z. Zuo and X.-Y. Hu, Mater. Adv., 2020, 1(8), 2646–2662 RSC;
(b) S. Chakraborty and G. R. Newkome, Chem. Soc. Rev., 2018, 47, 3991 RSC;
(c) F. Aparicio, M. J. Mayoral, C. Montoro-Garcıá and D. González-Rodríguez, Chem. Commun., 2019, 55, 7277 RSC.
-
(a) J. Mei, N. L. C. Leung, R. T. K. Kwok, J. W. Y. Lam and B. Z. Tang, Chem. Rev., 2015, 115, 11718–11940 CrossRef CAS PubMed;
(b) Y. Hong, J. W. Y. Lam and B. Z. Tang, Chem. Commun., 2009, 4332–4353 RSC;
(c) J. Wu, W. Liu, J. Ge, H. Zhang and P. Wang, Chem. Soc. Rev., 2011, 40, 3483–3495 RSC;
(d) R. T. K. Kwok, C. W. T. Leung, J. W. Y. Lam and B. Z. Tang, Chem. Soc. Rev., 2015, 44, 4228–4238 RSC.
-
(a) H. T. Feng, Y. X. Yuan, J. B. Xiong, Y. S. Zheng and B. Z. Tang, Chem. Soc. Rev., 2018, 47, 7452–7476 RSC;
(b) J. Li, J. Wang, H. Li, N. Song, D. Wang and B. Z. Tang, Chem. Soc. Rev., 2020, 49, 1144–1172 RSC;
(c) H. B. Cheng, Y. Li, B. Z. Tang and J. Yoon, Chem. Soc. Rev., 2020, 49, 21–31 RSC.
-
(a) X. l. Huang, J. B. Song, B. C. Yung, X. H. Huang, Y. H. Xiong and X. Y. Chen, Chem. Soc. Rev., 2018, 47, 2873 RSC;
(b) S. H. Park, N. Kwon, J. H. Lee, J. Yoon and I. Shin, Chem. Soc. Rev., 2020, 49, 143–179 RSC.
-
(a) Q. Z. Du, P. Wu, P. Dramou, R. Chen and H. He, New J. Chem., 2019, 43, 1291 RSC;
(b) S. Q. Zhang, X. Liu, Q. X. Sun, M. L. Chen and J. H. Wang, Chem. Commun., 2021, 57, 8746 RSC;
(c) X. F. Wu, L. H. Li, W. Shi, Q. Y. Gong, X. H. Li and H. M. Ma, Anal. Chem., 2016, 88, 1440–1446 CrossRef CAS PubMed.
- J. H. Wang, H. T. Feng, J. Luo and Y. S. Zheng, J. Org. Chem., 2014, 79, 5746–5751 CrossRef CAS PubMed.
- The measurements were performed without buffer solutions to avoid complication by the buffer itself.
Footnote |
† Electronic supplementary information (ESI) available. See DOI: 10.1039/d2ra00293k |
|
This journal is © The Royal Society of Chemistry 2022 |
Click here to see how this site uses Cookies. View our privacy policy here.