DOI:
10.1039/D1RA08751G
(Paper)
RSC Adv., 2022,
12, 6881-6887
Highly efficient separation and enrichment of polyphenols by 6-aminopyridine-3-boronic acid-functionalized magnetic nanoparticles assisted by polyethylenimine
Received
1st December 2021
, Accepted 21st February 2022
First published on 1st March 2022
Abstract
Polyphenols have found a lot of therapeutic effects and potential applications such as antioxidant, anti-inflammatory, mutant resistance, immunosuppressant and anti-tumor properties. They can be divided into five main classes, namely flavonoids, phenolic acids, stilbenes, lignans, and others. Thus, the content detection of polyphenols in real samples such as fruit juice and tea is of great significance. Due to the presence of complex interfering components in actual samples, separation and enrichment of polyphenols prior to analysis is key. Therefore, it is quite necessary to establish a simple, low-cost and efficient purification method for cis-diol-containing polyphenols from real samples. Boronate affinity materials are able to reversibly bind cis-diol-containing compounds by forming a five- or six-membered boronic cyclic ester in aqueous media. However, conventional boronate affinity materials exhibited low binding capacity and high binding pH. In this study, the polyethyleneimine (PEI)-assisted 6-aminopyridine-3-boronic acid functionalized magnetic nanoparticles (MNPs) were developed to capture efficiently cis-diol-containing polyphenols under neutral condition. PEI was applied as a scaffold to amplify the number of boronic acid moieties. While 6-aminopyridine-3-boronic acid was used as an affinity ligand due to low pKa value and excellent water solubility toward polyphenols. The results indicated that the prepared boronic acid-functionalized MNPs provided high binding capacity and fast binding kinetics under neutral conditions. In addition, the obtained MNPs exhibited relatively high binding affinity (Kd ≈ 10−4 M), low binding pH (pH ≥ 6.0) and tolerance of the interference of abundant sugars.
Introduction
Polyphenols are a class of important compounds naturally present in plants including vegetables, fruits and teas. And they are gaining more and more attention due to their therapeutic effects such as antioxidant, anti-inflammatory, mutant resistance, immunosuppressant and anti-tumor properties.1 In addition, polyphenolic compounds also can be applied in many terminal products such as food dyes, bioactive packaging, paints, fertilizers, surfactants, rubber, plastics and curing agents.2 Polyphenols have aromatic rings with two or more hydroxyl groups. They can be divided into five main classes, namely flavonoids, phenolic acids, stilbenes, lignans, and others.3,4 Among them, flavonoids are the most abundant compounds because they are mainly synthesized through the phenylpropanoid pathway in plants.5 As derivatives of benzoic acid and cinnamic acid, phenolic acids are characterized by a high antioxidant activity.6 In addition, stilbenes are present in low abundance in the human diet in foods such as grapes, berries, peanuts, red wine, etc.7 The lignans are formed by two units of a phenylpropane derivative and found in wheat, citrus fruits, onions, etc. Therefore, the content detection of the above mentioned polyphenols, especially in fruit juice, beverage, tea, and Chinese medicine related drinks, is of great significance. Due to the complex interfering components in actual sample, separation and enrichment of polyphenols prior to analysis is of vital importance.8
Fortunately, most polyphenols contain cis-diol structure.9 Thus, they can be selectively separated and enriched by boronic acid-functionalized MNPs. Boronic acid-functionalized materials have been widely applied for selective extraction and separation of cis-diol-containing compounds.10–36 However, the binding capacity of conventional boronic acids functionalized materials toward cis-diols is relatively low. The number of boronic acid sites can be amplified using highly branched polyethyleneimine (PEI). PEI has several advantages including flexible chains, hydrophilic properties, easy post-modification, plentiful amino groups and low cost.37 Therefore, the PEI-assisted boronic acid functionalized MNPs are able to provide higher binding capacity. In addition, the use of alkaline solution exhibits two disadvantages. First, it is easy to oxidize the cis-diols of polyphenols.38 Second, the discharge of alkaline solution may cause secondary pollution in water. To this end, Li and co-workers prepared PEI-assisted 3-carboxybenzoboroxole functionalized MNPs for the specific capture of cis-diol-containing flavonoids under neutral conditions.39 However, due to its complex and difficult preparation, the boronic acid ligand 3-carboxybenzoboroxole is not suitable for industrial production. Furthermore, 3-carboxybenzoboroxole is not especially advantageous to acidic pH conditions for high binding capacity. Compared with the boronic acid ligand 3-carboxybenzoboroxole, 6-aminopyridine-3-boronic acid is more advantageous to acidic pH conditions due to the presence of a nitrogen atom in the heterocyclic ring. And 3-carboxybenzoboroxole require difficult preparation while 6-aminopyridine-3-boronic acid is commercially available.40 Thus, it is necessary to develop PEI-assisted 6-aminopyridine-3-boronic acid functionalized MNPs in order to address the issues of 3-carboxybenzoboroxole. Although 6-aminopyridine-3-boronic acid had been used as affinity ligands to prepare boronic acid functionalized MNPs,40 PEI was not applied in this reference, which would affect the binding properties to some extent. In addition, this is first report on boronic acid functionalized materials using polyphenols as the research targets.
In the study, we applied 6-aminopyridine-3-boronic acid and PEI as affinity ligands and a scaffold to prepare PEI-assisted 6-aminopyridine-3-boronic acid-functionalized MNPs for highly efficient capture of cis-diol-containing polyphenols under neutral condition. In this work, MNPs were used as supporting nanomaterials due to their good biocompatibility, superparamagnetic property, low toxicity and easy preparation.30,41 The preparation process includes the following several steps. First, NH2-functionalized MNPs were synthesized and then PEI was modified to MNPs through Schiff base reaction. Subsequently, PEI modified MNPs were functionalized with 6-aminopyridine-3-boronic acid. Due to the combination of PEI with 6-aminopyridine-3-boronic acid, the prepared boronic acid functionalized MNPs exhibited relatively high binding affinity (Kd ≈ 10−4 M), low binding pH (pH ≥ 6.0) and fast binding kinetics under neutral condition. In addition, the obtained boronate affinity MNPs exhibited high binding capacity and tolerance of the interference to abundant sugars.
Experimental
Reagents and materials
Branched polyethyleneimine (PEI) (Mw = 600, 1800, 10
000 and 70
000), 6-aminopyridine-3-boronic acid (PYBA), adenosine, deoxyadenosine, caffeic acid (CA), quercetin (QUE), catechin (CAT), piceatannol (PIC), p-hydroxy-cinnamic acid (PCA), kaempferol (KAE), epiafzelechin (EPI), resveratrol (RES) were obtained from J&K scientific (Shanghai, China). FeCl3·6H2O, NaOAc, ethylene glycol, sodium cyanoborohydride, anhydrous methanol and glutaraldehyde were purchased from Alfa Aesar (Tianjin, China). All reagents were of analytical grade.
Instruments
Transmission electron microscopy (TEM) was carried out on a JEM-1010 system (JEOL, Japan). UV absorbance data were measured on a U-3010 UV spectrophotometer (Kyoto, Japan).
Preparation of Fe3O4@PEI@PYBA
As depicted in Fig. 1, Fe3O4@PEI@PYBA was prepared according to the below three steps: (1) synthesis of NH2-functionalized MNPs, (2) preparation of PEI modified MNPs (Fe3O4@PEI) via Schiff base reaction of glutaraldehyde, (3) functionalization of 6-aminopyridine-3-boronic acid by the Schiff base reaction of glutaraldehyde. The NH2-functionalized MNPs were synthesized according to a previously reported method.42 The above NH2-functionalized MNPs of 200 mg were added to 40 mL anhydrous methanol containing 10% glutaraldehyde, and the mixture was mechanically stirred for 4 h at room temperature. The glutaraldehyde-activated MNPs were washed three times with anhydrous methanol and then dispersed in 30 mL anhydrous methanol containing 0.8 g PEI by ultrasound. 1% (w/w) sodium cyanoborohydride was added (200 mg every 6 hours) during the course of reaction. The Fe3O4@PEI were collected by a magnet, washed with water and ethanol for 3 times each, and then dried at 40 °C overnight.
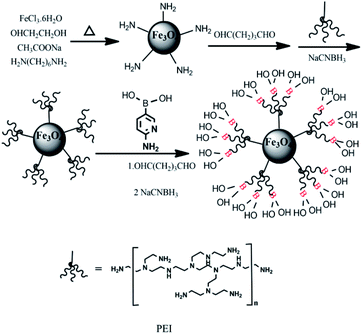 |
| Fig. 1 Synthesis routes of Fe3O4@PEI@PYBA. | |
In addition, the Fe3O4@PEI of 100 mg was added to 20 mL anhydrous methanol containing 10% glutaraldehyde and the mixture was stirred for 4 h at room temperature. Then, the obtained glutaraldehyde-activated NPs were dispersed in 30 mL anhydrous methanol containing 0.6 g PYBA. Finally, cyanoborohydride was added into the above solution (200 mg every 6 hours) for 24 h. The obtained Fe3O4@PEI@PYBA was separated from reaction mixtures using a magnet, and was washed with water and ethanol and dried at 50 °C. The obtained Fe3O4@PEI@PYBA was stored for further use.
Selectivity of Fe3O4@PEI@PYBA
The selectivity of Fe3O4@PEI@PYBA was evaluated using caffeic acid (CA), quercetin (QUE), catechin (CAT) and piceatannol (PIC) as cis-diol-containing polyphenolic samples while p-hydroxy-cinnamic acid (PCA), kaempferol (KAE), epiafzelechin (EPI), resveratrol (RES) were used as non-cis-diol analogue. First, the above mentioned each polyphenol solution of 0.5 mg mL−1 was prepared with 50 mM phosphate buffer (pH 7.0). Then equivalent Fe3O4@PEI@PYBA (5 mg) were added to 500 μL of the polyphenol solutions in centrifugal tubes, respectively. Then, they were shaken on a rotator at room temperature for 2 h. The Fe3O4@PEI@PYBA was then collected and rinsed with 500 μL PBS (pH 7.0) for three times. After washing, the MNPs were eluted in 100 μL acetic acid solution for 1 h on a rotator and the eluates were collected. The eluent containing these polyphenols adsorbed by the Fe3O4@PEI@PYBA were measured with UV-vis spectrophotometer. UV absorbance was adopted at 350 nm, 376 nm, 280 nm, 330 nm, 300 nm, 365 nm, 285 nm and 308 nm for CA, QUE, CAT, PIC, PCA, KAE, EPI and RES, respectively. UV absorbance measurement was repeated three times.
Kinetic adsorption experiment
CA was applied as a representative sample to investigate the kinetic adsorption experiment. Fe3O4@PEI@PYBA of 3 mg was added to 0.5 mL of CA solution (1 mg mL−1) and the obtained suspension was shaken for different time from 1 to 14 min at room temperature. The obtained suspension at different interaction time were collected by magnetic force and rinsed with PBS (pH 7.0) for three times. After washing, CA bound on Fe3O4@PEI@PYBA was eluted with acetic acid solutions (pH 2.7) and the supernatant was measured by absorbance analysis.
Measurement of adsorption isotherm and Scatchard analysis
The measurement of adsorption isotherm and Scatchard analysis was carried out according to the following process. First, an amount of Fe3O4@PEI@PYBA was dispersed to 50 mM PBS to form homogeneous suspension (20 mg mL−1). Then, 300 μL solutions at different concentrations of CA were added to the above Fe3O4@PEI@PYBA suspension of 200 μL and shaken on a rotator for 1 h at room temperature. Thereafter, CA-bound Fe3O4@PEI@PYBA were separated and then washed with PBS (pH 7.0) for 3 times. Finally, the compounds bound on the Fe3O4@PEI@PYBA were eluted by acetic acid solution. The eluent containing CA was measured with absorbance.
The Scatchard analysis was performed according to a previously reported method.43 Dissociation constant (Kd) and binding capacity (Qmax) were evaluated based on the following Scatchard equation:
where
Qmax and
Kd is the saturated binding capacity and the dissociation constant, respectively,
Qe is the binding amount of CA to Fe
3O
4@PEI@PYBA at equilibrium,
Cs is the free concentration at binding equilibrium. The values for
Kd and
Qmax are evaluated based on the slope and the intercept of plots of
Qe/
Cs versus Qe.
Results and discussion
Characterization of the magnetic nanoparticles (MNPs)
Transmission electron microscopy (TEM) was used to investigate the morphology of the prepared MNPs. As depicted in Fig. 2, the obtained Fe3O4@PEI@PYBA exhibited good dispersibility and relatively homogeneous size distribution. The size of the Fe3O4@PEI@PYBA was about 50 nm at diameter, which implied large specific surface area.
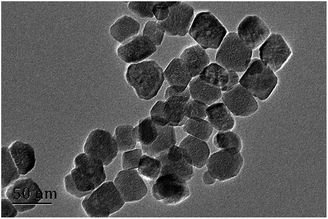 |
| Fig. 2 TEM of Fe3O4@PEI@PYBA. | |
Selectivity
In order to investigate the selectivity of the obtained Fe3O4@PEI@PYBA, adenosine and deoxyadenosine were used as a cis-diol-containing sample and a non-cis-diol sample, respectively. As shown in Fig. 3A, Fe3O4@PEI@PYBA exhibited higher binding capacity with adenosine than that with deoxyadenosine, which indicated excellent selectivity. To further demonstrate the selectivity of Fe3O4@PEI@PYBA toward cis-diol-containing polyphenols, CA, QUE, CAT and PIC were used as cis-diol-containing polyphenols and PCA, KAE, EPI and RES were used as non-cis-diol analogue. It can be observed from Fig. 3B that cis-diols polyphenols exhibited higher binding capacity than non-cis-diol polyphenols for Fe3O4@PEI@PYBA, indicating good selectivity.
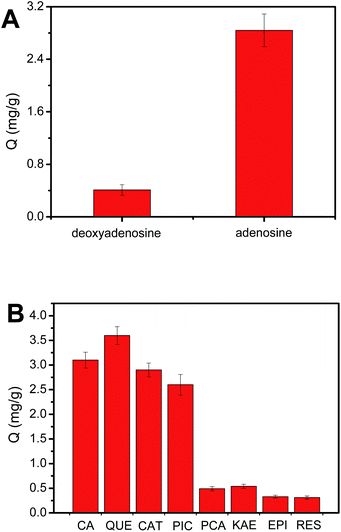 |
| Fig. 3 The binding capacity of Fe3O4@PEI@PYBA for different samples. Binding buffer: 50 mM sodium phosphate buffer (pH 7.0); elution solution: 100 mM HAc (pH 2.7); samples: (A) 0.50 mg mL−1 adenosine or deoxyadenosine; (B) 0.50 mg mL−1 CA, QUE, CAT, PIC, PCA, KAE, EPI or RES dissolved in binding buffer. | |
Optimization of the molecular weight of PEI
Generally, amount of the amine groups of PEI is proportional to the molecular weight of PEI. However, when molecular weight of PEI reaches a certain value, crosslinking reaction would occur and thereby a portion of amine groups may become invalid. Thus, it is necessary to investigate the effect of the molecular weight of PEI on the number of the binding sites, which can be reflected by binding capacity (Q, mg g−1). Based on this, we investigated the effect of the molecular mass of PEI on the binding capacity. It has been noted that the amount of functional monomer must be guaranteed to be excess. As depicted in Fig. 4, for every polyphenol, the binding capacity increased with the increase of molecular weight of PEI from 600 to 10
000. However, when molecular weight of PEI reaches 70
000, the binding capacity becomes lower. Thus, the PEI 10
000-modified PYBA-functionalized MNPs were considered as the optimal boronic acid-functionalized magnetic nanoparticles for further investigations and application.
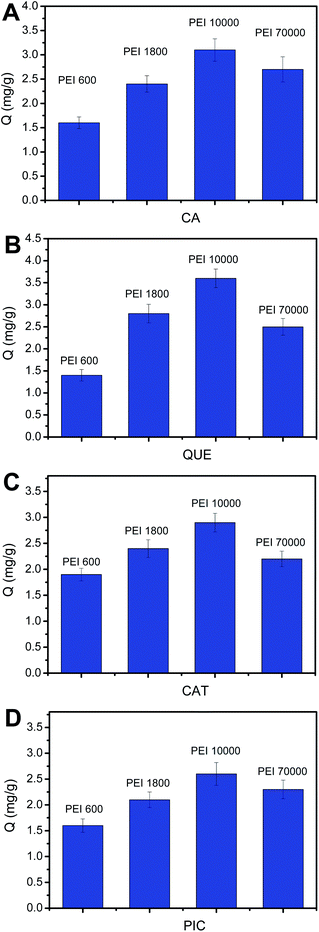 |
| Fig. 4 The binding capacity of CA (A), QUE (B), CAT (C) and PIC (D) samples captured by Fe3O4@PEI@PYBA through changing molecular weight of PEI. Binding buffer: 50 mM sodium phosphate buffer (pH 7.0); elution solution: 100 mM HAc (pH 2.7); the concentration of samples: 0.50 mg mL−1. | |
Determination of Qmax and Kd
Binding capacity is a crucial factor in boronate affinity materials, which determines how high amount of targets would be captured by the affinity material. Binding affinity is one important binding property, which determines how low concentrations of cis-diol-containing polyphenols that Fe3O4@PEI@PYBA can enrich. Thus, it is quite important to evaluate the binding capacity and binding affinity. The maximum binding capacity (Qmax) and dissociation constant (Kd) of Fe3O4@PEI@PYBA with CA were investigated using UV-vis spectrophotometry.
As shown in Fig. 5, according to binding isotherms and Scatchard plots analyses, Qmax and Kd values of Fe3O4@PEI@PYBA were calculated to be (3.56 ± 0.22) mg g−1 and (4.30 ± 0.35) × 10−4 M, respectively. Clearly, the obtained binding capacity value was a little higher than that the previous boronate affinity materials provided.39,40 Thus, the amplified 6-aminopyridine-3-boronic acid moieties by using PEI is advantageous for the binding affinity and binding capacity of Fe3O4@PEI@PYBA.
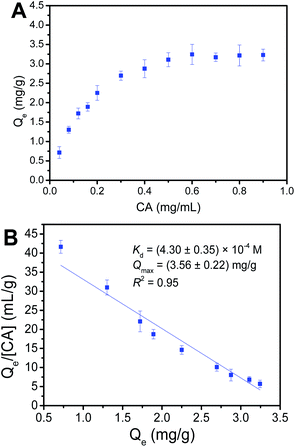 |
| Fig. 5 Binding isotherms (A) and Scatchard plots (B) for the binding of Fe3O4@PEI@PYBA with caffeic acid (CA). | |
Adsorption time
An appropriate adsorption time is required to obtain the highest binding capacity during the extraction procedure. To this end, the effect of extraction time on the binding capacity of polyphenols was investigated by varying time from 1 min to 8 min (Fig. 6). It can be observed from Fig. 6, the binding capacity of Fe3O4@PEI@PYBA for CA exhibited a fast adsorption rate within the first 3 min. After 3 min, the binding capacity nearly kept essentially constant. This possible explanation is that amount of binding sites at the beginning made CA be easily bound to Fe3O4@PEI@PYBA and the binding reaction would reach equilibrium at last. The results indicated that most of the binding sites had been occupied by CA in 3 min situation, which was compared to that PEI-assisted 3-carboxybenzoboroxole functionalized MNPs exhibited.39 This adsorption time at chemical equilibrium for Fe3O4@PEI@PYBA is much shorter than that for other boronate affinity materials (30–90 min).44–48 This may result from its high binding force of polyphenols during the extraction. In the light of these results, 3 min was chosen as the optimum adsorption time for extraction.
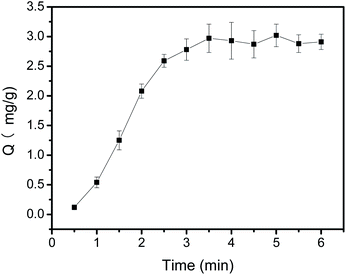 |
| Fig. 6 Binding equilibrium of Fe3O4@PEI@PYBA with CA. Sample: 0.50 mg mL−1 CA containing 50 mM phosphate, pH 7.0. | |
Effect of competing saccharides on the extraction of polyphenols by Fe3O4@PEI@PYBA
Sugars is also a kind of cis-diol-containing compounds. Sugars and polyphenols co-exists in many actual samples such as in fruits, vegetables, tea, honey and coffee. The presence of sugars may greatly affect the binding capacity of Fe3O4@PEI@PYBA for polyphenols. Thus, the investigation on their competing binding with Fe3O4@PEI@PYBA is necessary. As depicted in Fig. 7, the binding capacity of Fe3O4@PEI@PYBA for CA in the presence of a competing monosaccharide at 10-fold higher concentration was only a little less than that in the absence of sugar. Clearly, the presence of the competing saccharide has no apparent effect on the binding of CA to Fe3O4@PEI@PYBA. This possible explanation is that the Fe3O4@PEI@PYBA exhibited much higher binding affinity towards polyphenols than towards saccharides because polyphenols contain phenols while saccharides contain aliphatic alcohol. Thus, the Fe3O4@PEI@PYBA was very tolerant of the interference of competing saccharides.
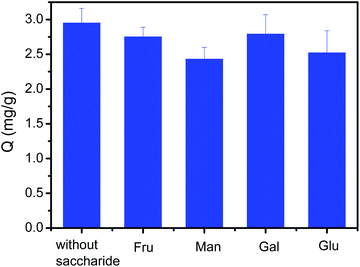 |
| Fig. 7 Effect of competing saccharides on the binding capacity of Fe3O4@PEI@PYBA for CA. Samples: 0.1 mg mL−1 CA without or with 1 mg mL−1 saccharide, fructose (Fru), mannose (Man), galactose (Gal) and glucose (Glu). | |
Binding pH
Binding pH value is an important binding property of boronic acid-functionalized materials. As we know, conventional boronate affinity materials used boronic acid with high pKa value as affinity ligand, which requires a basic pH condition. That's to say, the binding affinity is higher at alkaline condition than neutral or acidic condition. Unfortunately, the basic pH condition is easy to oxidize the polyphenols. It is quite necessary to prepare boronic acid-functionalized materials that can work at neutral or acidic condition. To address this issue, PYBA with electron-withdrawing group can be used to reduce the binding pH to neutral or acidic conditions. Thus, the prepared PYBA-functionalized MNPs can provide a lower binding pH value. As depicted in Fig. 8, the Fe3O4@PEI@PYBA NPs exhibited high binding capacity for CA at pH ≥ 6.0. The Fe3O4@PEI@PYBA are still able to well capture CA even at pH 4.0 and 5.0, which was superior to PEI-assisted 3-carboxybenzoboroxole functionalized MNPs.39 The above results implied that Fe3O4@PEI@PYBA can work under neutral or acidic conditions.
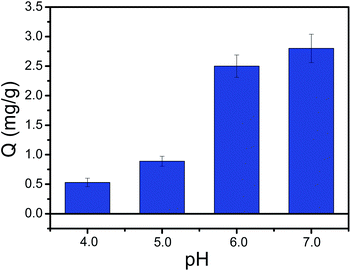 |
| Fig. 8 pH on the effect of the binding capacity of Fe3O4@PEI@PYBA towards CA. Binding buffer: 50 mM sodium phosphate buffer at pH 4.0–7.0. | |
Reproducibility and re-usability
In order to investigate reproducibility of the obtained Fe3O4@PEI@PYBA, the prepared six batches of Fe3O4@PEI@PYBA on different days were applied and the measurements replicated three times in parallel. By calculation, the average Q of the total Fe3O4@PEI@PYBA for CA was 2.82 mg g−1, and the relative standard deviations (RSD) were less than 7.3% for CA, which indicated that the reproducibility of the Fe3O4@PEI@PYBA was satisfactory.
Re-usability is one of the most important properties of boronate affinity materials in practical application. As far as we know, the Fe3O4@PEI@PYBA can be reused after washing with acid solution. In order to investigate the re-usability of Fe3O4@PEI@PYBA, the adsorption–regeneration cycle was repeated ten times with the same Fe3O4@PEI@PYBA. The results indicated that Fe3O4@PEI@PYBA are very stable and adsorption capacity still maintain 91.4% of the first cycle after five adsorption–desorption cycles. That's, the Fe3O4@PEI@PYBA could be well reused at least five times.
Conclusions
In this study, the PEI-assisted boronic acid-functionalized MNPs were prepared for the selective enrichment of polyphenols. By using branched PEI as the scaffold for amplification of boronic acid sites and 6-aminopyridine-3-boronic acid as the affinity ligand, PEI-assisted boronic acid-functionalized MNPs can obtain high binding capacity. The obtained dissociation constant is 10−4 M. In addition, the combination of PEI-assisted synergistic effect and novel boronic acid ligands can lead to lower binding pH. Due to the high binding strength, high binding capacity and the low binding pH, the boronate affinity MNPs can be directly applied to selective enrichment of polyphenols in real samples.
Conflicts of interest
There are no conflicts to declare.
References
- D. P. Farias, I. A. Neri-Numa, F. F. Araújo and G. M. Pastore, Food Chem., 2019, 306, 125630 CrossRef PubMed.
- Y. Farrag, W. Ide, B. Montero, M. Rico, S. Rodríguez-Llamazares, L. Barral and R. Bouza, Int. J. Biol. Macromol., 2018, 118, 2201–2207 CrossRef CAS PubMed.
- M. Larrauri, M. P. Zunino, J. A. Zygadlo, N. R. Grosso and V. Nepote, Ind. Crops Prod., 2016, 94, 964–971 CrossRef CAS.
- G. Grosso, U. Stepaniak, R. Topor-Madry, K. Szafraniec and A. Pajak, Nutrition, 2014, 30, 1398–1403 CrossRef CAS PubMed.
- P. C. H. Hollman, J. Sci. Food Agric., 2001, 81, 842–852 CrossRef CAS.
- C. S. Yang, J. M. Landau, M. T. Huang and H. L. Newmark, Annu. Rev. Nutr., 2001, 21, 381–406 CrossRef CAS PubMed.
- J. Chong, A. Poutaraud and P. Hugueney, Plant Sci., 2009, 177, 143–155 CrossRef CAS.
- F. D. Romagnolo and O. I. Selmin, Journal of Nutrition in Gerontology and Geriatrics, 2012, 31, 206–238 CrossRef PubMed.
- S. T. Zhang, X. W. He, L. X. Chen and Y. K. Zhang, New J. Chem., 2014, 38, 4212–4218 RSC.
- W. Zhou, N. Yao, G. P. Yao, C. H. Deng, X. M. Zhang and P. Y. Yang, Chem. Commun., 2008, 557, 7–5579 Search PubMed.
- M. Chen, Y. Lu, Q. Ma, L. Guo and Y. Q. Feng, Analyst, 2009, 134, 2158–2164 RSC.
- J. Tang, Y. Liu, P. Yin, G. P. Yao, G. Q. Yan, C. H. Deng and X. M. Zhang, Proteomics, 2010, 10, 2000–2014 CrossRef CAS PubMed.
- Z. A. Lin, J. L. Pang, H. H. Yang, Z. W. Cai, L. Zhang and G. N. Chen, Chem. Commun., 2011, 47, 9675–9677 RSC.
- F. Yang, Z. A. Lin, X. W. He, L. X. Chen and Y. K. Zhang, J. Chromatogr. A, 2011, 1218, 9194–9201 CrossRef CAS PubMed.
- Z. A. Lin, J. L. Pang, Y. Lin, H. Huang, Z. W. Cai, L. Zhang and G. N. Chen, Analyst, 2011, 136, 3281–3288 RSC.
- Z. J. Liu, K. Ullah, L. P. Su, F. Lv, Y. L. Deng, R. J. Dai, Y. J. Li and Y. K. Zhang, J. Mater. Chem., 2012, 22, 18753–18756 RSC.
- L. T. Liu, Y. Zhang, L. Zhang, G. Q. Yan, J. Yao, P. Y. Yang and H. J. Lu, Anal. Chim. Acta, 2012, 753, 64–72 CrossRef CAS PubMed.
- Y. Y. Qu, X. Liu, Z. Liang, L. H. Zhang and Y. K. Zhang, Chem.–Eur. J., 2012, 18, 9056 CrossRef CAS PubMed.
- Z. F. Xu, K. M. A. Uddin and L. Ye, Macromolecules, 2012, 45, 6464 CrossRef CAS.
- X. T. Shen, C. G. Xu, K. M. A. Uddin, P. O. Larsson and L. Ye, J. Mater. Chem. B, 2013, 1, 4612–4618 RSC.
- F. Yang, J. Mao, X. W. He, L. X. Chen and Y. K. Zhang, Anal. Bioanal. Chem., 2013, 405, 5321–5331 CrossRef CAS PubMed.
- H. Li, Y. H. Shan, L. Z. Qiao, A. Dou, X. Z. Shi and G. W. Xu, Anal. Chem., 2013, 85, 11585–11592 CrossRef CAS PubMed.
- G. B. Xu, W. Zhang, L. M. Wei, H. J. Lu and P. Y. Yang, Analyst, 2013, 138, 1876–1885 RSC.
- S. T. Zhang, X. W. He, L. X. Chen and Y. K. Zhang, New J. Chem., 2014, 38, 4212–4218 RSC.
- X. H. Zhang, J. W. Wang, X. W. He, L. X. Chen and Y. K. Zhang, ACS Appl. Mater. Interfaces, 2015, 7, 24576–24584 CrossRef CAS PubMed.
- J. W. Wang, X. W. He, L. X. Chen and Y. K. Zhang, RSC Adv., 2016, 6, 47055–47061 RSC.
- X. Y. An, X. W. He, L. X. Chen and Y. K. Zhang, J. Mater. Chem. B, 2016, 4, 6125–6133 RSC.
- J. A. Yang, X. W. He, L. X. Chen and Y. K. Zhang, J. Chromatogr. A, 2017, 1513, 118–125 CrossRef CAS PubMed.
- L. F. Chang, X. W. He, L. X. Chen and Y. K. Zhang, Sens. Actuators, B, 2017, 243, 72–77 CrossRef CAS.
- D. J. Li, Y. Chen and Z. Liu, Chem. Soc. Rev., 2015, 44, 8097–8123 RSC.
- D. J. Li, Z. J. Bie, F. F. Wang and E. H. Guo, Analyst, 2018, 143, 4936–4943 RSC.
- D. J. Li, H. J. Xia and L. Wang, Talanta, 2018, 184, 235–243 CrossRef CAS PubMed.
- D. J. Li, T. Y. Tu, M. K. Yang and C. Xu, Talanta, 2018, 184, 316–324 CrossRef CAS PubMed.
- D. J. Li and Z. J. Bie, Analyst, 2017, 142, 4494–4502 RSC.
- D. J. Li, Q. Yuan, W. L. Yang, M. K. Yang, S. H. Li and T. Y. Tu, Anal. Biochem., 2018, 561–562, 18–26 CrossRef CAS PubMed.
- D. J. Li, T. Y. Tu and X. Y. Wu, Anal. Methods, 2018, 10, 4419–4429 RSC.
- D. J. Li, Y. Li, X. L. Li, Z. J. Bie, X. H. Pan, Q. Zhang and Z. Liu, J. Chromatogr. A, 2015, 1384, 88–96 CrossRef CAS PubMed.
- S. C. Liu, J. M. Pan, H. J. Zhu, G. Q. Pan, F. X. Qiu, M. J. Meng, J. T. Yao and D. Yuan, Chem. Eng. J., 2016, 290, 220–231 CrossRef CAS.
- D. J. Li, Z. Y. Liu, R. M. Song, W. L. Yang, S. M. Zhai and W. H. Wang, RSC Adv., 2019, 9, 38038–38046 RSC.
- D. J. Li and S. H. Dong, Anal. Methods, 2021, 13, 2331–2337 RSC.
- V. Matsura, Y. Guari, J. Larionova, C. Guerin, A. Caneschi, C. Sangregorio, E. Lancelle-Beltran, A. Mehdi and R. J. P. Corriu, J. Mater. Chem., 2004, 14, 3026–3033 RSC.
- L. Y. Wang, J. Bao, L. Wang, F. Zhang and Y. D. Li, Chem.–Eur. J., 2006, 12, 6341–6347 CrossRef CAS PubMed.
- M. Dowlut and D. G. Hall, J. Am. Chem. Soc., 2006, 128, 4226–4227 CrossRef CAS PubMed.
- X. D. Bi and Z. Liu, Anal. Chem., 2014, 86, 959–966 CrossRef CAS PubMed.
- X. D. Bi and Z. Liu, Anal. Chem., 2014, 86, 12382–12389 CrossRef CAS PubMed.
- Z. A. Lin, L. X. Sun, W. Liu, Z. W. Xia, H. H. Yang and G. N. Chen, J. Mater. Chem. B, 2014, 2, 637–643 RSC.
- Y. Hao, R. X. Gao, D. C. Liu, G. Y. He, Y. H. Tang and Z. J. Guo, Talanta, 2016, 153, 211–220 CrossRef CAS PubMed.
- Y. X. Li, M. Hong, M. Miao, Q. Bin, Z. Y. Lin, Z. W. Cai and G. N. Chen, J. Mater. Chem. B, 2013, 1, 1044–1051 RSC.
|
This journal is © The Royal Society of Chemistry 2022 |
Click here to see how this site uses Cookies. View our privacy policy here.