DOI:
10.1039/D0RA10236A
(Paper)
RSC Adv., 2021,
11, 9303-9314
Phase separation affects the rheological properties of starch dough fortified with fish actomyosin†
Received
4th December 2020
, Accepted 11th February 2021
First published on 1st March 2021
Abstract
Starch and protein are common polymers in food, and their phase separation often occurs during food processing. Protein-fortified starch dough can be considered as a triple phase separation system, and the effect of phase separation on dough rheology warrants further research. In this study, starch doughs fortified with fish actomyosin were used, and their rheological properties were researched and explained with respect to phase separation. The results suggested that the phase separation of actomyosin-binder-starch granules in the raw dough affected the quality of dough. The addition of actomyosin significantly decreased stiffness and shear sensitivity but increased the fluidity of the blended dough. Moreover, it was found that the interaction between mung bean starch and actomyosin was very weak. The polymer molecules were connected by physical links. Owing to phase separation, it was presumed that “wall slip” occurred between the binder, starch granule, and actomyosin. The blended dough containing 30% of the added actomyosin (R3) showed the best recovery ability and the weakest molecular interaction (interaction type Z′ = 0.40 for storage modulus G′ and 0.31 for loss modulus G′′). Additionally, the phase structure of the model doughs was investigated. It was found that the starch network played a dominant role when 10% (R1) actomyosin was added. With the addition of actomyosin, the protein network formed gradually. A bicontinuous phase structure with interpenetrating network was observed in R3 (actomyosin = 30%). In summary, our findings demonstrate the feasibility to make blended doughs by mixing fish actomyosin and mung bean starch. Moreover, in terms of use in traditional noodle making, the blended R3 dough was found to be the best in terms of recovery ability and flow characteristics.
1. Introduction
Consumption of starch noodles is one of the most distinguished cultures prevalent in Asia. Mung bean starch is considered one of the best raw materials available for noodle making. It is preferred over other starches and imparts desirable attributes to noodles such as transparency, a fine thread-like structure, high tensile strength, and low cooking loss.1,2 In recent years, the need for mung bean starch noodles has gradually increased worldwide, especially in Asia; however, the production of mung bean starch is limited and is more expensive compared to other available starches. This makes it difficult to meet the market needs and bridge the gap between demand and supply. Therefore, it is worthwhile to identify substitutes for mung beans to help reduce production costs. Hydrocolloids are the most common and most studied food additives. They are useful in enhancing the texture and the rheological properties of starch noodles.3–5 Apart from hydrocolloids, chitosan, glycerol monostearate, and other modified starches are also good choices for noodles making.1,2,6 However, studies suggest that the use of these compounds leads consumers to perceive the end products as “artificial foods,” thus rendering them less desirable.7 Therefore, the use of natural proteins as additives is a more suitable alternative. The addition of protein to starch noodles has two other benefits. First, starch is a polysaccharide that rapidly hydrolyzes into glucose upon consumption, rendering it unsuitable to obese individuals or those with diabetes. Protein addition can effectively reduce the relative intake levels of starch. Moreover, some studies have shown that protein addition to starch-based foods can effectively delay the release of glucose.8–10 Secondly, from a nutritional point of view, foods containing pure starch are inferior owing to the lack of protein, micronutrients, vitamins, and other nutrients; therefore, fortification with protein can address this deficit.
The most common proteins used as additives are soybean protein, egg white protein, and whey protein. Studies show that the addition of these proteins has a significant effect on the rheological properties of starch dough.11–14 Actomyosin extracted from fish has appreciable gel-forming abilities and is one of the main components in the manufacture of solid surimi gels.15,16 Therefore, fish actomyosin has the potential to improve the quality of starch-containing foods. To the best of our knowledge, few studies have explored the effect of fish actomyosin on the quality of mung bean starch-containing foods; moreover, relevant research studying their rheological behavior is even less. Rheology is mainly concerned with the force and deformation of materials and is an important parameter in the comprehensive evaluation of starch–protein dough blends. A rheometer can simulate many processing methods. For instance, using the steady shear test simulates the “dropping” step to characterize its thixotropy, the “mixing” step characterizes the flow behavior, and the dynamic frequency sweep can simulate the high-frequency vibration to evaluate the interaction strength of the polymers.
Phase separation of proteins and polysaccharides is a common occurrence in foods. Understanding their phase behavior may, therefore, help improve the quality of gelatinous foods.17 Several studies focus on phase separation, which has resulted in improving the feasibility of the production of foods with special textural properties;18,19 therefore, this field is currently attracting significant attention.20–22 There are few studies that explore the phase separation of surimi protein and starch blends. Compared to the doughs made using gluten-containing grains, one of the biggest differences in non-gluten containing dough is that a binder is used to replace the gluten for the formation of a gel network in the latter, which results in a significant weakening of the network in the dough. The protein–starch dough blend can be considered a triple-phase system consisting of a binder, starch granules, and protein. The phase-separation behavior of this system is more complex; therefore, the effect of phase separation on the rheological characteristics of the blend is worthy of further exploration.
In this study, we determined the effect of phase separation on the rheological properties of starch dough fortified with fish actomyosin. Rheological characteristics, including flow, thixotropy, viscoelasticity, interaction strength and creep and recovery, were analyzed and explained from the perspective of phase separation. This investigation could provide a better understanding of the mechanism of blended doughs and the theoretical knowledge required for the manufacture of protein-fortified starch products.
2. Materials and methods
2.1 Materials
Fresh sea bass (Lateolabrax japonicus) was purchased from a local aquatic products market. The white meat on the back was collected and rinsed 2 times using cold deionized water, then stored in a −80 °C freezer until using. High purity mung bean starch (ingredients: protein 0%, amylose 31.8%, fat 0%, carbohydrates 0.13%) was provided by Chengdongwang food Co., Ltd (Chengdu, China).
2.2 Preparation of actomyosin paste
Actomyosin was prepared according to the method of Donald and Lanier with some modifications.23 Sea bass muscle (50 g) was minced for 2 min using a meat chopper (TJE17A-300, Supor, Zhejiang, China) in 500 mL extraction solution (0.6 M KCl, 4 °C) then homogenized for 4 min at 10
000 rpm using a homogenizer (FJH-300, Shanghai specimen and model factory, China). The beaker containing the sample was placed in ice and each 20 s of blending was followed by a 20 s rest interval to avoid overheating during extraction. The extract was centrifuged at 5000g for 30 min at 4 °C. Three volumes of chilled deionized water were added to precipitate actomyosin. Actomyosin was collected by centrifuging at 5000g for 20 min at 4 °C, and the pellet was dissolved by stirring for 30 min at 4 °C in an equal volume of chilled 1.2 M KCl, pH 7.0. Undissolved material was removed from the preparation by centrifugation at 5000g for 20 min at 4 °C. Then the actomyosin was collected by precipitating with three volumes of chilled deionized water and centrifugation (5000g, 20 min, 4 °C) again. The content of protein was measured as 60.54 ± 0.41 mg mL−1 (approximately 6 wt%), according to the Biuret method.24
2.3 Preparation of raw actomyosin–starch dough
Starch dough was prepared based on the methods of Wang with some modifications combined with traditional handmade procedure of China.25 30 g dry starch was added with 70 g deionized water and stirred for 2 min with a rod, then 200 g boiling water was slowly pour in while stirring to form a binder, after that 300 g dry starch was added in and mixed with a blender (HM740, HanShang, Shandong, China) at 100 rpm for 5 min to form a starch dough. Waiting for the temperature of the starch dough cooling down to 40 °C, raw starch–actomyosin dough of five different mixing ratios (9
:
1, 8
:
2, 7
:
3, 6
:
4, 5
:
5) were prepared separately by adding actomyosin to starch dough (w/w) and mixed with the blender at 100 rpm for 10 min, the samples were marked sequentially as R1–R5. The starch dough without adding actomyosin was set as control and marked R0. All samples were stored in a 4 °C refrigerator for 6 h before testing.
2.4 Rheological properties of doughs
The rheological behaviors of the doughs were measured using a rotational rheometer (DHR1, TA instruments, USA) fitted with parallel-plate geometry (40 mm diameter, 400 μm gap). Before testing, each sample was placed between plates and rested for 10 min. The rim of the dough sample was coated with silicone oil to prevent water evaporation.
2.4.1 Steady shear tests. For assessing the flow characters of doughs, each sample was tested with the “flow ramp” mode of rheometer. The temperature was set as 25 °C, and the shear rate increased stepwise from 0.01 s−1 to 300 s−1 (upward) and immediately decreased from 300 s−1 to 0.01 s−1 (downward). The data of shear stress (τ, Pa) and shear rate (γ, s−1) from upward which corresponding to the beginning to the visible fluctuation point were recorded and fitted the Herschel–Bulkey model (eqn (1)) to characterize the yield stress (τ0, Pa), flow behavior index (n, dimensionless) and consistency coefficient (K, Pa sn).26The degree of thixotropy (Dt) of the doughs were expressed by the area of the hysteresis loop (s−1 Pa s) between the upward and downward curves. The calculation of Dt was performed by using software Origin 8.0. To ensure valid comparisons between the obtained Dt results, they were acquired across the shear thixotropic region from 0.6 s−1 to 148 s−1 of all the doughs.27
2.4.2 Dynamic strain sweep. Dynamic strain sweep was performed over a strain range from 0.01% to 200% at 25 °C, and the angular frequency was 10 s−1. The strain sweep gave the information of relative strength of junction zone formed within the doughs (characterized by storage modulus G′) and the relative resistance to flow (characterized by loss modulus G′′).
2.4.3 Dynamic frequency sweep. In the sweep procedure, the G′ and G′′ were recorded as functions of frequency, which preformed from 0.1 to 100 s−1 at a strain of 0.5% and temperature of 25 °C. To calculate and express the type (Z′) of molecular interactions and strength (K) of the dough, the G′ and frequency values (ω) were fitted to the power law model (logarithmic form) as flowing.33
2.4.4 Temperature sweep. In order to understand the viscoelastic change during the cooling of doughs, the temperature sweep was performed from 25 °C to 90 °C at 5 °C min, and then cooling down from 90 °C to 25 °C at the same rate as before immediately. The G′ and G′′ of the samples were recorded at a constant frequency of 1 Hz and strain of 0.5%. The loss factor (tan
δ) was also recorded during the temperature sweep. |
tan δ = G′′/G′
| (3) |
2.4.5 Creep and recovery measurements. Creep and recovery measurement of doughs were performed at a constant temperature of 25 °C, the creep phase a shear stress of 50 Pa was applied for 50 s, then a recovery phase followed by that, the stress was removed immediately and lasted for 150 s. The data of stain (γ) and stress (σ) was collected and analyzed by the Trios (software, version 5.0). For a better understanding the relationship of and stain and stress, compliance J = f(t) = γ/σ was introduced, and the parameters including the maximum creep compliance (Jmax), zero shear viscosity (η0), mean retard time (λ) relative elastic part of the Jmax (Je/Jmax) and the relative viscous part of the Jmax (Jv/Jmax) was obtained by fitting the Burger model as followed equations:28For the creep phase
|
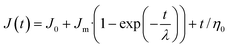 | (4) |
For the recovery phase
|
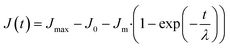 | (5) |
2.5 Phase structure
15 g starch dough was prepared according to the method in Section 2.3, and then put into a steamer (SZ26B5, Supor, Zhejiang, China) to cooking for 15 min. Following that the cooked doughs were removed into cold water for 10 min and drained for 5 min. About 1 cm3 of samples were cut off and stuck to the sample holder using glue, after that, the samples were frozen and sliced into 15 μm sheets using a frozen slicer (CM1850, Leica, Germany), and then put on the slide and stained with 1/5 concentration of Lugol solution (I2 = 0.33%, KI = 0.67%, w/v) for 1 min, Fast Green (0.1%, w/v) for 1 min. Following that, the samples were rinsed with ironless water for 1 min, covered with glass and observed under a 100× optical microscope (80i, Nikon, Japan).
2.6 Statistical analysis
All tests were performed in triplicate unless specified and the mean scores were taken as the result. The data was evaluated by an analysis of variance (ANOVA), and a comparison of means was carried out with Duncan's test. Differences were considered significant at p < 0.05. Statistical computation and analysis were conducted using SPSS (18.0).
3. Result and discussion
3.1 Flow characteristics of blended dough
The steady shear curves of the five different mixed starch–protein doughs and the control R0 are shown in Fig. 1. With an increase in the amount of added actomyosin, the shear stress (τ) of dough decreased significantly at the same shear rate, which can likely be ascribed to the relative decrease in the addition of pregelatinized starch. Mung bean starch is rich in amylose (≈30.9–34.3%) compared to other native starch and plays a crucial role in gelation.29,30 In the starch dough, part of the starch was gelatinized and served as a binder. Amylose is the main component that forms the gel network. It has a strong influence on the flow characteristics of the entire dough. Moreover, the shear stress of the doughs was found to decrease sequentially with a decrease in the addition of starch. Presumably, actomyosin has a fairly weak interaction with mung bean starch in the dough. The gel network between the starch and protein was probably formed independently and interpenetrated the microstructure. Several studies have confirmed this assumption.31 Wu et al. researched the thermal properties of eight types of dough made using a varying composition of starch and actomyosin and found that the thermal transition proceeds independently.32 Kong et al. have reported similar findings.
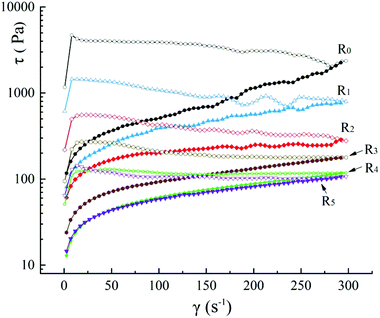 |
| Fig. 1 Steady shear curves of the actomyosin–starch doughs (note: τ: shear stress; γ: shear rate. R1–R5: dough blends prepared from mung bean starch and actomyosin using mixing ratios ranging from 9 : 1 to 5 : 5. R0: dough prepared from mung bean starch without the addition of actomyosin. The hollow and solid points represent the upward and downward curves, respectively). | |
Traditionally, “dropping” is a crucial step in noodle making, which is induced by continuous patting of the mold that loads the dough, thereby imposing shear stress on the dough.33 A steady shear test using a rheometer can simulate this condition. In the upward curves, it can be thought of as a light pat changing to a heavy pat, wherein the shear stress of the dough starts with a quick rise and then exhibits a slow fall. In the downward curves, the pat motion and intensity follow a reverse trend and the shear force drops rapidly. The shear stress can be used to represent the resistance force to the deformation of the dough caused by the shear rate.34 Based on the findings in Fig. 1, we can conclude that for the production of starch noodles using mung bean starch–actomyosin doughs, the ideal stress applied should be reduced from strong to weak (downward curves), but not increased from weak to strong (upward curves). This pattern provided better fluidity to the doughs. The addition of actomyosin gradually decreased the extent of shear thinning of the different model doughs.
The Herschel–Bulkey model was used to accurately characterize the flow properties of the doughs. Owing to severe fluctuations, most of the upward curves of the starch doughs cannot be suitably fitted to the Herschel–Bulkley model to obtain rheological parameters.25 Our results show a similar pattern. Therefore, only the doughs that exhibited a shear rate between 0 s−1 and 100 s−1 of downward curves, as indicated in Fig. 1, were fitted to the Herschel–Bulkey model. The results are listed in Table 1 (sample fitted plots are shown in ESI Fig. S1†). It can be seen that the values of n (flow behavior index) for all samples are less than 1, and that there are no significant differences between them, indicating that these samples are pseudoplastic fluids. All samples exhibited a shear-thinning tendency, which is consistent with the results listed in Section 3.1. The yield stress τ0, represents the mechanical energy that breaks the structure of the dough and enables the flow.35 A higher τ0 implies a higher resistance experienced in the flow of the dough. The τ0 decreased from 124.274 Pa to 14.453 Pa, suggesting that the addition of actomyosin considerably increased the fluidity of the dough. This phenomenon may be related to the relative decrease in amylose content as the weak gel structure exhibited by starch is mainly formed by amylose. The consistency coefficient (K) can be used to characterize the stiffness of the dough and shows a positive proportional relationship. In our study, K showed a decreased trend, indicating that the doughs had a tendency of softening after the addition of actomyosin. The observed trends are consistent with the results listed in Section 3.1.
Table 1 Herschel–Bulkley and thixotropy parameters for actomyosin–mung bean starch doughsa
Treatment |
τ0 (Pa) |
K (Pa sn) |
n |
R2 |
Dt (s−1 Pa s) |
Note: τ0: yield stress. K: consistency coefficient. n: flow behavior index. R1–R5: doughs prepared form mung bean starch and actomyosin with mixing ratio from 9 : 1 to 5 : 5, R0: dough prepared from mung bean starch as control. Results in the table represent the mean of triplicate measurements. Mean ± standard deviation. Different letters in each column indicate significant differences (p < 0.05). |
R0 |
124.274 ± 10.711a |
81.383 ± 3.741a |
0.420 ± 0.063a |
0.996 |
19 696 ± 879a |
R1 |
87.089 ± 9.607b |
56.889 ± 4.576b |
0.438 ± 0.072a |
0.994 |
4843 ± 208b |
R2 |
64.834 ± 6.240c |
40.726 ± 3.460c |
0.394 ± 0.113a |
0.998 |
1538 ± 96c |
R3 |
26.757 ± 5.248d |
16.382 ± 3.554d |
0.365 ± 0.133a |
0.999 |
745 ± 43d |
R4 |
16.409 ± 2.331e |
9.736 ± 1.744e |
0.378 ± 0.092a |
0.998 |
380 ± 19e |
R5 |
14.453 ± 3.182e |
8.210 ± 1.987f |
0.433 ± 0.052a |
0.999 |
349 ± 11f |
3.2 Thixotropy of blended doughs
Thixotropy is used to reflect the shear sensitivity of the dough and can be measured using the areas of the hysteresis loop (Dt) of the flow curves. A higher Dt value indicates that the dough has a better ability to change its viscosity. The hysteresis loops of all six model doughs are shown in Fig. 2 separately and the value of Dts are listed in Table 1. It can be seen that as the shear rate increases, the viscosity starts to decline sharply and continues to reduce gradually.36 These results are similar to the results obtained using wheat dough. A sharp decrease in the viscosity of doughs at a low shear rate (<150 s−1) suggested that the structure of dough was considerably destroyed. The slow decrease under a high shear rate (>150 s−1) indicated that “wall slip” may exist between the dough molecules.37 Wang et al. reported the “wall slip” phenomenon when they researched the interaction between the binder and starch granules in starch dough.25 They found that the binder forms a “low-shear-strength film” under a continuous shear action; the film acts as a continuous phase, whereas the starch granules form the dispersed phase. Moreover, the molecules in starch dough have shown to undergo rearrangement at high shear rates.38 In our study, the doughs can be considered to constitute a triple-phase system (actomyosin-binder-starch granules). We inferred that the wall slip was introduced by the binder in the samples containing low levels of protein, which served as the continuous phase, whereas the proteins and starch granules constituted the dispersed phase. In samples containing high levels of added protein, wall slip was introduced by actomyosin, the protein constituted the continuous phase, and the binder and starch particles formed the dispersed phase. When the ratio of protein to starch is appropriate, the protein and binder may form a bicontinuous phase with the starch granules acting as fillers.35 The study of Crawford et al. supports our conclusion. With regard to R0 to R2, significant fluctuations in viscosity were observed at a high shear rate, which perhaps explains the shear destruction of the film formed by the binder.39
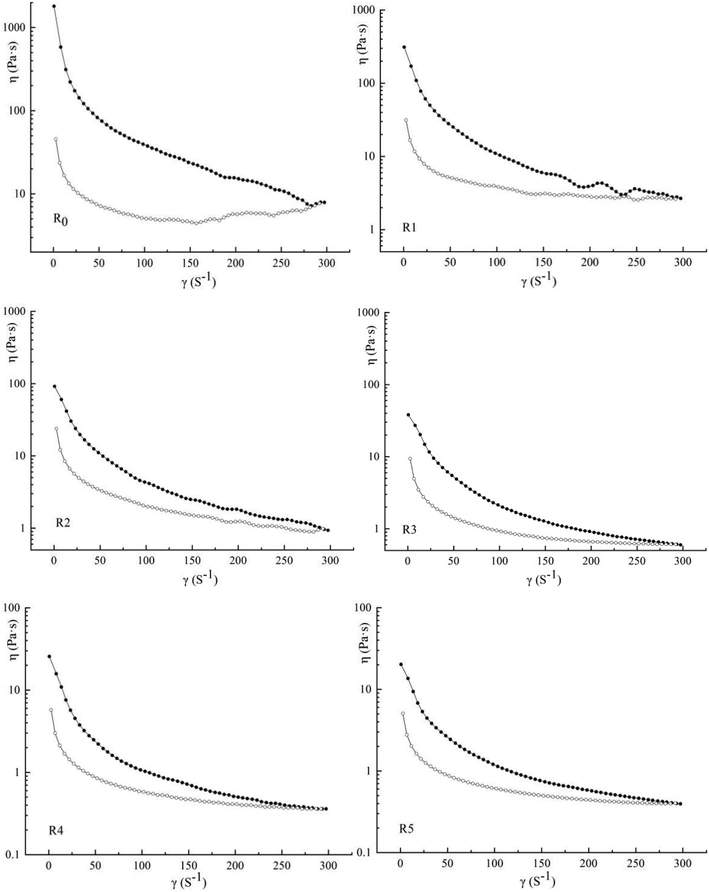 |
| Fig. 2 Hysteresis loops of blended doughs (note: τ: shear stress. η: viscosity. R1–R5: dough blends prepared from mung bean starch and actomyosin with mixing ratios ranging from 9 : 1 to 5 : 5. R0: dough prepared from mung bean starch without the addition of actomyosin. Upward curves: solid points; downward curves: hollow points). | |
The mechanism of thixotropic behavior in starch doughs may be related to the irreversible fracture of the starch gel formed by the binder paste.25 Moreover, this was also the main reason why the upward and downward curves did not coincide. It can be seen from the results that the Dt values decreased gradually, indicating that the addition of actomyosin considerably weakened the extent of the damage. During the actual production process, Dt sometimes does not play a guiding role because the “dropping” method involves a more complex rheological behavior, which is similar to extensional flow.
3.3 Viscoelasticity of blended raw doughs
Dynamic strain sweep was used to determine the linear viscoelasticity (LVR) of the starch doughs. As seen in Fig. 3, the LVRs of the starch dough with different protein content were limited up to a strain of 1.0%. The viscoelasticity started to decrease obviously when the strain continued to increase, indicating the breakdown of dough structure beyond a certain level of deformation. Previous studies show that the LVR of doughs made using raw wheat range from 0.1% to 0.25% (40some fermented wheat doughs show a very high value), which is far below that of model doughs prepared using mung bean starch and fish actomyosin investigated in this study.41–43 This may be attributed to the interaction between the mung bean starch and actomyosin, their respective properties, and water-binding ability.
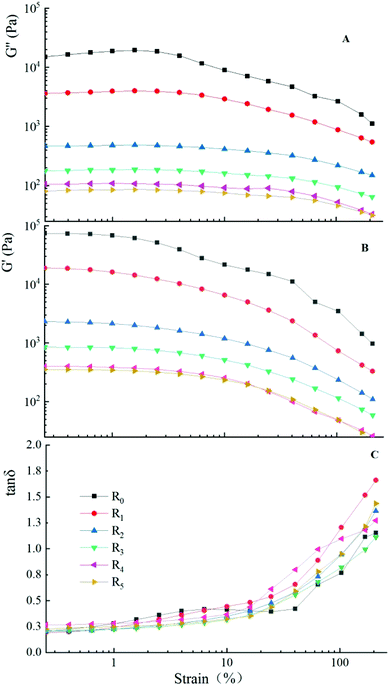 |
| Fig. 3 Dynamic strain sweep curves for starch–actomyosin model doughs. Note: G′: storage modulus. G′′: loss modulus. tan δ: loss factor. R1–R5: dough blends prepared from mung bean starch and actomyosin with mixing ratios ranging from 9 : 1 to 5 : 5, R0: dough prepared from mung bean starch without the addition of actomyosin. (A) Storage modulus (G′) versus strain (%). (B) Loss modulus (G′′) versus strain (%). (C) Loss factor (tan δ) versus strain (%). | |
Generally, starch doughs form a weak and fragile polysaccharide network. Their structures are unstable under a high shear strain and can undergo shear thinning easily.25,44 This can be verified from the R0 in Fig. 3. With an increase in the amount of added actomyosin, the trend of weakening of both G′ and G′′ and the subsequent decrease significantly implied that protein addition made the dough less prone to shear-thinning and enabled it to hold its shape. It was obvious that actomyosin changed the flow characteristics and enhanced the stability of the dough. Feng et al. determined the effect of the addition of egg white on the LVR of dough prepared using sweet potato starch.45 They found that the viscoelasticity of common starch decreased significantly when the strain was more than 0.1%, but the starch–protein dough samples appeared more stable. The trend observed in their study is similar to the results obtained in our study. Furthermore, Zhang et al. reported similar findings when they studied the LVR of starch–hydroxypropylmethylcellulose (HPMC) dough.46
Furthermore, for all doughs, the elastic component (G′) was found to remain well above the viscous component (G′′), which indicated that the raw doughs had characteristics of solids but not liquids.47 Moreover, the addition of actomyosin resulted in a sharp decrease of G′ and G′′, ranging from 10
000 to around 100. These findings implied that the addition of actomyosin did not change the solid characteristics of doughs, rather, made it softer compared to the control.
3.4 Analysis of the interaction strength of molecules in model doughs
Dynamic frequency sweep simulates the stability of dough at different vibrations. Moreover, when this method is used, the frequency varies with time while the stress remains constant (within the LVRs), suggesting that the dough structure is not damaged. Therefore, this test provides an approach to understanding the interaction between actomyosin and mung bean starch molecules in model doughs.48 The parameters including the type of molecular interactions, Z′, and strength of the doughs, K′, are shown in Table 2. In general, the Z′ values represent the dependent degree of frequency on the modulus (G′ or G′′). A Z′ value of 0 indicates that there are covalent bonds between the molecules, the G′ or G′′ are frequency independent, and that the dough has a very stable network. Z′ > 0 indicates that there are physical links between molecules, whereas a higher Z′ is suggestive of weaker interactions.49 It can be seen that the Z′ values of all doughs are >0 and show a tendency of an initial rise followed by a fall in both G′ and G′′ (Table 2). The model dough R3 shows the maximum Z′ value of 0.40 for G′ and 0.31 for G′′. We speculate that these values may also be related to the phase separation of the blended system, in which the proteins and binder likely form a bicontinuous phase, although the network structures are very weak. In fact, this conclusion was confirmed by subsequent observations of the microstructures of doughs using optical methods of analysis. K represents the physical strength of the dough, and it can be seen that the values of K gradually decreased with the addition of actomyosin.50 This may be attributed to the gel nature of actomyosin as well as the mung bean starch. The gel strength of the protein is much lower than that of starch.
Table 2 The characteristic values of actomyosin–mung bean starch doughs in dynamic frequency sweepa
Treatment |
G′ |
G′′ |
Z′ |
K |
R2 |
Z′ |
K |
R2 |
Note: G′: storage modulus. G′′: loss modulus. Z′: molecular interaction type. K: molecular interaction strength. R1–R5: doughs prepared from mung bean starch and actomyosin with a mixing ratios ranging from 9 : 1 to 5 : 5, R0: dough prepared from mung bean starch and set as control. Results in the table represent the mean of triplicate measurements. Mean ± standard deviation. Different letters in each column indicate significant differences (p < 0.05). |
R0 |
0.16 ± 0.04c |
283 576 ± 1164a |
0.997 |
0.07 ± 0.01c |
67 852 ± 911a |
0.997 |
R1 |
0.27 ± 0.03b |
80 299 ± 892b |
0.999 |
0.17 ± 0.03b |
26 955 ± 538b |
0.998 |
R2 |
0.30 ± 0.02b |
9063 ± 399c |
0.998 |
0.23 ± 0.03b |
3181.4 ± 264c |
0.999 |
R3 |
0.40 ± 0.04a |
2831 ± 107d |
0.998 |
0.31 ± 0.03a |
1011.7 ± 77d |
0.999 |
R4 |
0.29 ± 0.01b |
2113 ± 68e |
0.999 |
0.22 ± 0.01b |
906.54 ± 53e |
0.999 |
R5 |
0.21 ± 0.04c |
291 ± 23f |
0.995 |
0.16 ± 0.02c |
114.54l ± 15f |
0.996 |
3.5 Creep and recovery characters of doughs
The system deformation per unit stress is defined as compliance and is measured over time.51 The results showed that all the model doughs exhibited a typical viscoelastic behavior combining both viscous fluid and elastic components (Creep–recovery plots are shown in ESI Fig. S2†). The creep deformation consists of three stages, namely, instantaneous deformation, retarded deformation, and plastic deformation. Each stage is individually induced by the deformation of bond length and bond angle, segmental motion, and flow of linear amorphous polymers.52 When the stress is removed suddenly, the dough experiences a recovery deformation consisting of two stages, namely, instantaneous recovery and steady deformation.53 In reality, there is no clear boundary between these five stages and it is difficult to separate them quantitatively.
The effect of the actomyosin on the creep–recovery parameters of model doughs are summarized in Table 3. J0, Jm, and Jmax represent the instantaneous compliance, viscoelastic compliance, and compliance of the creep end, respectively. Jmax is used to describe the rigidity of the doughs and it reflects the maximum resistance to deformation as an indicator of the bond strength between the structural units. In other words, the stronger dough had a smaller Jmax value. During the creep stage, it can be seen that with an increase in the proportion of the added protein, all three compliance parameters increased significantly. These findings suggest that the doughs became soft after protein addition and the interaction between the protein and polymer molecules was considerably weakened. This conclusion can also be drawn based on the increasing value of γ, as seen in Table 3. Moreover, zero shear viscosity (η0) is an important parameter that was used to represent the flow character of doughs when the stress was removed. A higher η0 indicated more resistance for the dough to retain its original shape. R5 exhibited the highest compliance parameters and lowest η0, indicating the fine fluidity of the dough. Fan et al. obtained similar results during the study of fish myofibrillar protein-cassava starch composites.54 The reason for this occurrence could be that the addition of proteins broke down the starch-gel network that was formed by the binder paste. Furthermore, the addition of actomyosin significantly improved the softness of the starch doughs. During the production of starch noodles using the extrusion method, these aspects of the dough may facilitate the extrusion of the noodles from the molds.
Table 3 Rheological parameters of actomyosin–mung bean starch blended doughs in creep–recovery testa
Treatment γ |
Creep phase |
Recovery phase |
J0 × 10−4 |
Jm × 10−4 |
λ |
η0 × 102 |
Jmax × 10−3 |
J0 × 10−3 |
Jm × 10−3 |
λ |
Note: R1–R5: dough blends prepared from mung bean starch and actomyosin with mixing ratios ranging from 9 : 1 to 5 : 5, R0: dough prepared from mung bean starch without the addition of actomyosin. γ, strain at the end of creep phase (%): J0, instantaneous compliance (Pa−1). Jm, viscoelastic compliance (Pa−1). λ, mean retardation time (s). η0, zero shear viscosity (Pa s). Jmax, maximum creep compliance (Pa−1). Je, steady-state compliance (Pa−1). Different letters in each column indicated significant differences (p < 0.05). |
R0 |
0.14 ± 0.06f |
12 ± 1.6f |
17.9 ± 1.9f |
1.62 ± 0.06b |
510 ± 11.6a |
2.9 ± 0.6f |
2.4 ± 0.6f |
2.7 ± 0.6f |
128.0 ± 9.9f |
R1 |
0.56 ± 0.09e |
52.6 ± 5.7e |
79.7 ± 5.8e |
1.38 ± 0.02c |
203 ± 18.9b |
11.2 ± 1.6e |
7.9 ± 1.1e |
10.8 ± 0.9e |
60.5 ± 3.6e |
R2 |
0.74 ± 0.05d |
63.4 ± 5.9d |
95.1 ± 4.8d |
1.23 ± 0.04d |
100 ± 6.3c |
17.8 ± 1.4d |
11.3 ± 2.1d |
11.5 ± 1.3d |
58.1 ± 5.7d |
R3 |
2.66 ± 0.52c |
210 ± 13.3c |
378.9 ± 15.3c |
1.28 ± 0.03d |
50 ± 8.6d |
59.2 ± 11.4c |
36.4 ± 1.4c |
135.1 ± 14.3c |
4.2 ± 0.9c |
R4 |
9.19 ± 1.43b |
462 ± 24.9b |
1106.6 ± 33.5b |
1.69 ± 0.02b |
7.6 ± 2.7e |
183.8 ± 25.8b |
133.5 ± 14.7b |
352.6 ± 19.5b |
54.3 ± 4.4b |
R5 |
24.02 ± 2.04a |
638 ± 25.1a |
2190.1 ± 71.4a |
2.19 ± 0.02a |
1.8 ± 0.5f |
465.5 ± 58.8a |
428 ± 27.3a |
493.9 ± 25.2a |
204.4 ± 19.2a |
In the recovery phase, the compliance of doughs gradually decreased from the maximum value (Jmax) to a stable state. The recovered compliance is referred to as elastic compliance (Je). The compliance phase wherein the dough recovery reaches equilibrium at the terminal region is referred to as Jv.55 Creep–recovery experiments can be used to simulate the rheological behavior of the dough under high stress, such as the recovery of dough deformation after suddenly pressing the dough with a finger. Results of the proportion analyses of Je and Jv in Jmax are shown in Fig. 4. A higher Je/Jmax value indicates a better recovery ability after deformation. The addition of actomyosin changed the Je/Jmax values of model starch doughs significantly. When the amount of the added protein was 30% (R3), the Je/Jmax value reached a maximum of 84.15%. The high recovery ability of R3 may be related to the rheological properties of the actomyosin paste and mung bean starch doughs themselves.
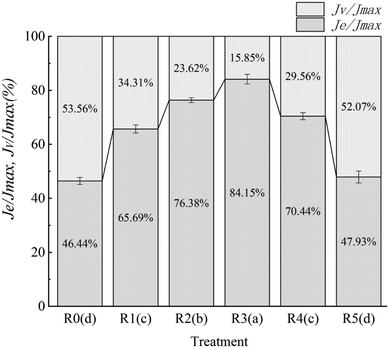 |
| Fig. 4 Effect of actomyosin on the relative elastic (Je, dots) and viscous (Jv, lines) portion of maximum creep compliance (Jmax) in the recovery phase of creep tests. Treatments followed by the same letter are not significantly different at P < 0.05 (note: R1–R5: dough blends prepared from mung bean starch and actomyosin with mixing ratios ranging from 9 : 1 to 5 : 5, R0: dough prepared from mung bean starch without the addition of actomyosin). | |
3.6 Variation in viscoelasticity characteristics of doughs during thermal processing
A temperature sweep was performed to understand the viscoelastic change of doughs during heating. tan
δ is a parameter that describes the relationship between G′ and G′′. A high tan
δ indicates that there are relatively more viscous components and less elastic components, suggesting the shear resistance of starch doughs.26 Fig. 5 shows the plot of loss factor tan
δ as a function of temperature. The doughs could be classified into two groups based on the effect of temperature on tan
δ. When the added protein was less than 20%, a peak was observed (Fig. 5A), which could likely be attributed to the gelatinization of the mung bean starch. During this phase, amylose gradually leaked from the starch granules and the weak network formed by the binder was destroyed. When the level of added proteins exceeded 20%, three peaks were observed (Fig. 5B). The peaks on the left appeared around 40 °C and the middle peaks at around 56 °C. We speculated that this change might have stemmed from the unwinding of the head and tail of myosin. Liu et al. studied the effect of temperature on the gelation of the myosin from silver carp and found that the α-helix of the myosin head and tail unwound at temperatures higher than 40 °C and 55 °C, respectively. These findings were similar to the results of our study.56
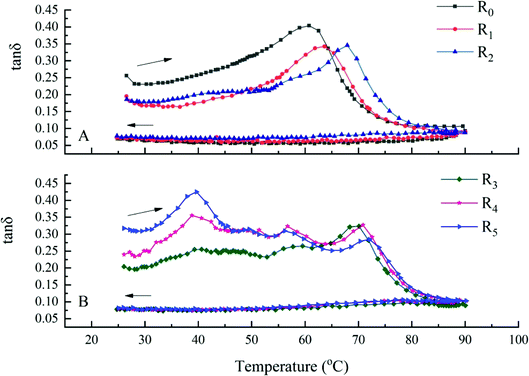 |
| Fig. 5 Effect of heating on loss factor (tan δ) in actomyosin–mung bean starch doughs (note: G′: storage modulus. G′′: loss modulus. tan δ: loss factor. R1–R5: dough blends prepared from mung bean starch and actomyosin with mixing ratios ranging from 9 : 1 to 5 : 5. R0: dough prepared from mung bean starch without the addition of actomyosin. (A) Curves of R0–R2. (B) Curves of R3–R5). | |
Furthermore, the addition of actomyosin increased the gelation temperature of mung bean starch. With an increase in the amount of added protein, the effect was more obvious. When the level of the added protein was 50%, the gelatinization temperature of starch increased from 60.77 °C to 72.08 °C and was ascribed to the inhibition of swelling of the starch granules by the protein. The addition of actomyosin resulted in an increasing amount of starch granules being wrapped by the protein, which inhibited the leaking of amylose to form the network. Collectively, these effects resulted in the need for more heat to enable the water molecules to enter the starch granules to complete the process of gelatinization. Our findings are in accordance also with similar studies that explored protein–starch and polysaccharide–starch blends.57–59
Based on the distribution range of the upward curves of the model doughs, it can be seen all the values of tan
δ were <1, indicating the more elastic and solid-like nature of the doughs.60 During the cooking period (downward curves), all the curves gradually appeared to exhibit a similar trend and the corresponding values were also relatively close (P < 0.05). These findings suggested that all model doughs, with the addition of actomyosin, formed the same elastic gel as the pure starch dough when cooled.
3.7 Phase structure of the cooked doughs
Based on the conclusions stated in the previous sections, we postulated that the doughs made using different blending ratios would have different phase structures owing to phase separation. To further prove the reliability of our theory, the model doughs were extruded into noodles and the phase structure was fixed by steaming. Schematic diagram of the phase structures and the corresponding optical photos of model doughs are shown in Fig. 6. Actomyosin was dyed green using fast green and starch was dyed light purple (amylose) or dark purple (amylopectin) using iodine, based on its binding ability and affinity toward the two components of starch. The red and blue curves represent starch and protein respectively.
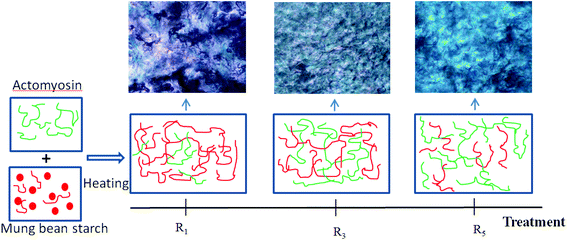 |
| Fig. 6 Schematic diagram of phase structure and the corresponding optical photos of blended doughs after heating (note: R1, R3, R5: doughs prepared from mung bean starch and actomyosin using a mixing ratio of 9 : 1, 7 : 3 and 5 : 5. Magnification: 100×. In the optical photos, starch is dyed purple, and protein is dyed green. In the schematic diagram, the red curves represent chain segments of starch, the red points represent starch granules, and the green curves represent chain segments of protein). | |
When protein was added to the starch noodles, a phase-separation process between actomyosin and mung bean starch was observed. In R1, it was obvious that starch formed a continuous phase while the protein constituted the dispersed phase. The protein network gradually formed after the addition of actomyosin. In the sample prepared using R3, a bicontinuous phase of starch and protein was formed and an interpenetrating network of actomyosin and mung bean starch was observed. In R5, the protein network appeared dominant with the starch dispersed within it.
Starch and proteins are polymers present in food that can easily undergo phase separation owing to thermodynamic incompatibility.61 Many previous studies have reported findings similar to ours.62 Schorsch et al. reported a phase separation of micellar casein and locust bean gum blends, wherein they found that different mixing ratios lead to different continuous appearances.54 Fan et al. also reported the formation of an interpenetrating network using various mixing ratios of fish myofibrillar protein and cassava starch. The benefit of phase separation is that it can result in changes in the food microstructure, leading to the food have different quality and taste.
4. Conclusion
In this study, we evaluated the phase separation of mung bean starch dough that was fortified with fish actomyosin. It was found that the different mixing ratios of starch and protein could lead to a different phase structure of the polymer blends, thereby affecting dough quality. The results showed that the addition of actomyosin enabled the dough to flow more easily. Moreover, it was found that the interaction between starch and protein was very weak, and their gel networks were interpenetrating. Thixotropic analysis indicated that the shear sensitivity of the dough blends decreased with an increase in actomyosin. Results of the dynamic rheological test indicated that the LVRs (linear viscoelasticity) of the starch doughs of all samples were limited up to a strain of 1.0%, and were higher than the values reported for traditional cereal doughs. On other hand, owing to phase separation, wall slip occurred in the triple-phase system comprising binder-protein–starch granules. Results of the interaction strength indicated very weak physical links between them. R3 showed maximum Z′ values 0.40 for G′ and 0.31 for G′′, indicating that the interaction between protein and starch was weakest in this dough compared to other preparations. Additionally, creep–recovery results indicated that R3 (protein addition: 30%) had the best recovery ability compared to the other raw doughs. Furthermore, the phase behavior and phase structure of blended doughs were studied during heating. It was found that all the samples could form a solid gel during the heating process. When the added protein was 10% (R1), the starch network played a dominant role. When the amount of added protein exceeded 10%, the protein network was found form gradually. Moreover, the addition of actomyosin increased the gelling temperature of mung bean starch. A bicontinuous phase structure with an interpenetrating network was observed in R3. An ideal starch based dough should have both flow resistance and an ability to recover from deformation. R3 suitably met these desirable properties and was thought to be the ideal blended dough. However, there are still many aspects worth studying further in this project. For example,63,64 large-amplitude oscillatory shear (LAOS) can be used to assess the nonlinear characteristics of viscoelastic responses. Furthermore, the interaction of protein and starch can be further studied at the molecular level using Fourier-transform infrared spectroscopy and thermodynamic analyses.
Funding
This work was supported by the National Natural Science Foundation of China (31972107, U20A2067), and Liaoning Revitalization Talents Program (XLYC 1907040).
Conflicts of interest
There are no conflicts to declare.
Acknowledgements
We would like to thank all the reviewers who participated in the review and MJEditor (www.mjeditor.com) for its linguistic assistance during the preparation of this manuscript.
References
- C. Y. Lii and S. M. Chang, Characterization of red bean (Phaseolus radiatus var. Aurea) starch and its noodle quality, J. Food Sci., 1981, 46(1), 78–81 CrossRef.
- A. Kaur, K. Shevkani and N. Singh, et al., Effect of guar gum and xanthan gum on pasting and noodle-making properties of potato, corn and mung bean starches, J. Food Sci. Technol., 2015, 52(12), 8113–8121 CrossRef CAS.
- E. Silva, M. Birkenhake and E. Scholten, et al., Controlling rheology and structure of sweet potato starch noodles with high broccoli powder content by hydrocolloids, Food Hydrocolloids, 2013, 30(1), 42–52 CrossRef CAS.
- T. Funami, Y. Kataoka and T. Omoto, et al., Effects of non-ionic polysaccharides on the gelatinization and retrogradation behavior of wheat starch, Food Hydrocolloids, 2005, 19(1), 1–13 CrossRef CAS.
- M. H. Lee, M. H. Baek and D. S. Cha, et al., Freeze–thaw stabilization of sweet potato starch gel by polysaccharide gums, Food Hydrocolloids, 2002, 16(4), 345–352 CrossRef CAS.
- H. Saito, M. Tamura and Y. Ogawa, Starch digestibility of various Japanese commercial noodles made from different starch sources, Food Chem., 2019, 283, 390–396 CrossRef CAS.
- A. Marti and M. A. Pagani, What can play the role of gluten in gluten free pasta?, Trends Food Sci. Technol., 2013, 31(1), 63–71 CrossRef CAS.
- K. S. Sandhu and S. T. Lim, Digestibility of legume starches as influenced by their physical and structural properties, Carbohydr. Polym., 2008, 71(2), 245–252 CrossRef CAS.
- R. M. Faulks and A. L. Bailey, Digestion of cooked starches from different food sources by porcine α-amylase, Food Chem., 1990, 36(3), 191–203 CrossRef CAS.
- A. S. Desai, M. A. Brennan and C. S. Brennan, Influence of semolina replacement with salmon (Oncorhynchus tschawytscha) powder on the physicochemical attributes of fresh pasta, Int. J. Food Sci. Technol., 2019, 54(5), 1497–1505 CrossRef CAS.
- F. Ronda, B. Oliete and M. Gómez, et al., Rheological study of layer cake batters made with soybean protein isolate and different starch sources, J. Food Eng., 2011, 102(3), 272–277 CrossRef.
- L. Kumar, M. A. Brennan and H. Zheng, et al., The effects of dairy ingredients on the pasting, textural, rheological, freeze-thaw properties and swelling behaviour of oat starch, Food Chem., 2018, 518–524 CrossRef CAS.
- L. Patrascu, I. Banu and I. Vasilean, et al., Rheological and Thermo-mechanical Characterization of Starch–Protein Mixtures, Agric. Agric. Sci. Procedia, 2016, 280–288 Search PubMed.
- P. D. Ribotta, A. Colombo and C. M. Rosell, et al., Enzymatic modifications of pea protein and its application in protein–cassava and corn starch gels, Food Hydrocolloids, 2012, 27(1), 185–190 CrossRef CAS.
- A. A. Nowsad, S. Kanoh and E. Niwa, Setting of transglutaminase-free actomyosin paste prepared from Alaska pollack surimi, Fish. Sci., 1994, 60(3), 295–297 CrossRef CAS.
- Y. Funatsu, N. Katoh and K. I. Arai, Aggregate formation of salt-soluble proteins in salt-ground meat from Walleye Pollack surimi setting, Nippon Suisan Gakkaishi, 1996, 62(1), 112–122 CrossRef CAS.
- S. L. Turgeon, M. Beaulieu and C. Schmitt, et al., Protein–polysaccharide interactions: phase-ordering kinetics, thermodynamic and structural aspects, Curr. Opin. Colloid Interface Sci., 2003, 8(4–5), 401–414 CrossRef CAS.
- L. Van den Berg, Y. Rosenberg and M. A. J. S. Van Boekel, et al., Microstructural features of composite whey protein/polysaccharide gels characterized at different length scales, Food Hydrocolloids, 2009, 23(5), 1288–1298 CrossRef CAS.
- F. van de Velde, E. H. A. de Hoog and A. Oosterveld, et al., Protein-polysaccharide interactions to alter texture, Annu. Rev. Food Sci. Technol., 2015, 6, 371–388 CrossRef CAS.
- Y. Xu, N. Yang and J. Yang, et al., Protein/polysaccharide intramolecular electrostatic complex as superior food-grade foaming agent, Food Hydrocolloids, 2020, 101, 105474 CrossRef CAS.
- E. J. Bealer, S. Onissema-Karimu and A. Rivera-Galletti, et al., Protein–Polysaccharide Composite Materials: Fabrication and Applications, Polymers, 2020, 12(2), 464 CrossRef CAS.
- C. L. Wu, Q. H. Chen and X. Y. Li, et al., Formation and characterisation of food protein–polysaccharide thermal complex particles: effects of pH, temperature and polysaccharide type, Int. J. Food Sci. Technol., 2020, 55(3), 1368–1374 CrossRef CAS.
- G. A. M. Donald and T. C. Lanier, Actomyosin Stabilization to Freeze-Thaw and Heat Denaturation by Lactate Salts, J. Food Sci., 1994, 59(1), 101–105 CrossRef.
- E. Layne, Spectrophotometric and turbidimetric methods for measuring proteins, Methods Enzymol., 1957, 10(73), 447–455 Search PubMed.
- Y. Wang, F. Ye and J. Liu, et al., Rheological nature and dropping performance of sweet potato starch dough as influenced by the binder pastes, Food Hydrocolloids, 2018, 39–50 CrossRef.
- L. Chen, Y. Tian and Y. Bai, et al., Effect of frying on the pasting and rheological properties of normal maize starch, Food Hydrocolloids, 2017, 77(4), 85–95 Search PubMed.
- H. Z. Tan, B. Tan and H. Gao, et al., Rheological Behavior of Mung Bean Starch Dough, Food Sci. Technol. Res., 2007, 13(2), 103–110 CrossRef.
- C. Onyango, C. Mutungi and G. Unbehend, et al., Creep-recovery parameters of gluten-free batter and crumb properties of bread prepared from pregelatinised cassava starch, sorghum and selected proteins, Int. J. Food Sci. Technol., 2010, 44(12), 2493–2499 CrossRef.
- H. Z. Tan, W. Y. Gu and J. P. Zhou, et al., Comparative Study on the Starch Noodle Structure of Sweet Potato and Mung Bean, J. Food Sci., 2006, 71(8), 447–455 CrossRef.
- V. Vamadevan and E. Bertoft, Structure-function relationships of starch components, Starch/Staerke, 2015, 67(1–2), 55–68 CrossRef CAS.
- M. C. Wu, T. C. Lanier and D. D. Hamann, et al., Thermal Transitions of Admixed Starch/Fish Protein Systems During Heating, J. Food Sci., 2006, 50(1), 20–25 CrossRef.
- C. S. Kong, H. Ogawa and N. Iso, et al., Compression properties of fish-meat gel as affected by gelatinization of added starch, J. Food Sci., 1999, 64(2), 283–286 CrossRef CAS.
- H. Tan, Z. Li and B. Tan, et al., Starch noodles: History, classification, materials, processing, structure, nutrition, quality evaluating and improving, Food Res. Int., 2009, 42(42), 551–576 CrossRef CAS.
- G. Tucker, Applications of rheological data into the food industry[M], Advances in Food Rheology and Its Applications, Woodhead Publishing, 2017, pp. 159–175 Search PubMed.
- N. C. Crawford, L. B. Popp and K. E. Johns, et al., Shear thickening of corn starch suspensions: Does concentration matter?, J. Colloid Interface Sci., 2013, 396(Complete), 83–89 CrossRef CAS.
- M. Gonzalez, I. Reyes and Y. Carrera-Tarela, et al., Charcoal bread: Physicochemical and textural properties, in vitro digestibility, and dough rheology, Int. J. Gastron. Food Sci., 2020, 21, 100227 CrossRef.
- V. Glicerina and S. Romani, Advances in Yield Stress Measurements for Chocolate[M], Cesena(FC), Italy, 2017 Search PubMed.
- A. Tecante and J. L. Doublier, Steady flow and viscoelastic behavior of crosslinked waxy corn starch-κ-carrageenan pastes and gels, Carbohydr. Polym., 1999, 40(3), 221–231 CrossRef CAS.
- E. K. Chamberlain, M. A. Rao and C. Cohen, Shear thinning and antithixotropic behavior of a heated cross-linked waxy maize starch dispersion, Int. J. Food Prop., 1999, 2(1), 63–77 CrossRef CAS.
- E. J. Vernon-Carter, S. Garcia-Diaz and I. Reyes, et al., Rheological and thermal properties of dough and textural and microstructural characteristics of bread with pulque as leavening agent, Int. J. Gastron. Food Sci., 2017, 9, 39–48 CrossRef.
- N. Phan-Thien and M. Safari-Ardi, Linear viscoelastic properties of flour–water doughs at different water concentrations, J. Non-Newtonian Fluid Mech., 1998, 74(1–3), 137–150 CrossRef CAS.
- N. Phan-Thien, M. Safari-Ardi and A. Morales-Patñ, Oscillatory and simple shear flows of a flour-water dough: a constitutive model, Rheol. Acta, 1997, 36(1), 38–48 CrossRef CAS.
- A. Y. Guadarrama-Lezama, H. Carrillo-Navas and E. J. Vernon-Carter, et al., Rheological and thermal properties of dough and textural and microstructural features of bread obtained from nixtamalized corn/wheat flour blends, J. Cereal Sci., 2016, 69, 158–165 CrossRef.
- S. Kasapis and A. Bannikova, Rheology and Food Microstructure[M], Melbourne, Vic, Australia, 2017 Search PubMed.
- Y. Y. Feng, T. H. Mu and M. Zhang, et al., Effects of different polysaccharides and proteins on dough rheological properties, texture, structure and in vitro starch digestibility of wet sweet potato vermicelli, Int. J. Biol. Macromol., 2020, 148, 1–10 CrossRef CAS.
- D. Zhang, T. Mu and H. Sun, Comparative study of the effect of starches from five different sources on the rheological properties of gluten-free model doughs, Carbohydr. Polym., 2017, 176, 345–355 CrossRef CAS.
- R. Moreira, F. Chenlo and M. D. Torres, Rheology of commercial chestnut flour doughs incorporated with gelling agents, Food Hydrocolloids, 2011, 25(5), 1361–1371 CrossRef CAS.
- D. Weipert, The benefits of basic rheometry in studying dough rheology, Cereal Chem., 1900, 67, 311–327 Search PubMed.
- D. Renard, F. van de Velde and R. W. Visschers, The gap between food gel structure, texture and perception, Food Hydrocolloids, 2006, 20(4), 423–431 CrossRef CAS.
- W. W. Kim and B. Yoo, Rheological behaviour of acorn starch dispersions: effects of concentration and temperature, Int. J. Food Sci. Technol., 2009, 44(3), 503–509 CrossRef CAS.
- M. Rubinstein and R. H. Colby, Polymer physics[M], Oxford university press, New York, 2003 Search PubMed.
- A. Abd Karim, M. H. Norziah and C. C. Seow, Methods for the study of starch retrogradation, Food Chem., 2000, 71(1), 9–36 CrossRef.
- F. C. Wang and X. S. Sun, Creep-recovery of wheat flour doughs and relationship to other physical dough tests and breadmaking performance, Cereal Chem., 2002, 79(4), 567–571 CrossRef CAS.
- M. Fan, T. Hu and S. Zhao, et al., Gel characteristics and microstructure of fish myofibrillar protein/cassava starch composites, Food Chem., 2017, 218, 221–230 CrossRef CAS.
- A. Lazaridou, D. Duta and M. Papageorgiou, et al., Effects of hydrocolloids on dough rheology and bread quality parameters in gluten-free formulations, J. Food Eng., 2007, 79(3), 1033–1047 CrossRef CAS.
- H. M. Liu, S. B. Xiong and L. Zhang, et al., Studies on heat-induced setting temperature of myosin from silver carp, J. Fish. China, 2010, 34(4), 643–647 CrossRef CAS.
- C. Yang, F. Zhong and H. D. Goff, et al., Study on starch-protein interactions and their effects on physicochemical and digestible properties of the blends, Food Chem., 2019, 280, 51–58 CrossRef CAS.
- D. Liu, Z. Li and Z. Fan, et al., Effect of soybean soluble polysaccharide on the pasting, gels, and rheological properties of kudzu and lotus starches, Food Hydrocolloids, 2019, 89, 443–452 CrossRef CAS.
- I. O. Mohamed and J. Babucurr, Effect of date syrup on pasting, rheological, and retrogradation properties of corn starch gels, Starch/Staerke, 2015, 67(7–8), 709–715 CrossRef CAS.
- S. Gunasekaran and M. M. Ak, Dynamic oscillatory shear testing of foods—selected applications, Trends Food Sci. Technol., 2000, 11(3), 115–127 CrossRef CAS.
- X. T. Le, L. E. Rioux and S. L. Turgeon, Formation and functional properties of protein-polysaccharide electrostatic hydrogels in comparison to protein or polysaccharide hydrogels, Adv. Colloid Interface Sci., 2016, 239, 127–135 CrossRef.
- C. Schorsch, A. H. Clark and M. G. Jones, et al., Behaviour of milk protein/polysaccharide systems in high sucrose, Colloids Surf., B, 1999, 12(3–6), 317–329 CrossRef CAS.
- J. Alvarez-Ramirez, R. Escarela-Perez and E. J. Vernon-Carter, et al., Large amplitude oscillatory shear (LAOS) rheology of nixtamalized corn masa, J. Cereal Sci., 2019, 88, 31–37 CrossRef.
- J. Alvarez-Ramirez, Y. Carrera-Tarela and H. Carrillo-Navas, et al., Effect of leavening time on LAOS properties of yeasted wheat dough, Food Hydrocolloids, 2019, 90, 421–432 CrossRef CAS.
Footnote |
† Electronic supplementary information (ESI) available. See DOI: 10.1039/d0ra10236a |
|
This journal is © The Royal Society of Chemistry 2021 |