DOI:
10.1039/D0SC02060E
(Edge Article)
Chem. Sci., 2020,
11, 5238-5245
Differentiating Aβ40 and Aβ42 in amyloid plaques with a small molecule fluorescence probe†
Received
13th April 2020
, Accepted 29th April 2020
First published on 11th May 2020
Abstract
Differentiating amyloid beta (Aβ) subspecies Aβ40 and Aβ42 has long been considered an impossible mission with small-molecule probes. In this report, based on recently published structures of Aβ fibrils, we designed iminocoumarin–thiazole (ICT) fluorescence probes to differentiate Aβ40 and Aβ42, among which Aβ42 has much higher neurotoxicity. We demonstrated that ICTAD-1 robustly responds to Aβ fibrils, evidenced by turn-on fluorescence intensity and red-shifting of emission peaks. Remarkably, ICTAD-1 showed different spectra towards Aβ40 and Aβ42 fibrils. In vitro results demonstrated that ICTAD-1 could be used to differentiate Aβ40/42 in solutions. Moreover, our data revealed that ICTAD-1 could be used to separate Aβ40/42 components in plaques of AD mouse brain slides. In addition, two-photon imaging suggested that ICTAD-1 was able to cross the BBB and label plaques in vivo. Interestingly, we observed that ICTAD-1 was specific toward plaques, but not cerebral amyloid angiopathy (CAA) on brain blood vessels. Given Aβ40 and Aβ42 species have significant differences of neurotoxicity, we believe that ICTAD-1 can be used as an important tool for basic studies and has the potential to provide a better diagnosis in the future.
Introduction
Amyloid beta (Aβ) plaques, one of the characteristic biomarkers for Alzheimer's disease (AD), have been discovered for more than 100 years in the postmortem brains of AD patients.1,2 However, the role of Aβ plaques in the pathology of AD has still been heavily debated, because the correlation between plaque burdens (numbers and areas) and the severity of AD is poor.3–5 In the plaques, Aβ40 and Aβ42 peptides are major constituents. Nonetheless, unlike the role of plaque, there is nearly no argument that Aβ42 has much higher neurotoxicity than Aβ40 does.1,2,4,6,7 Conceivably, differentiating Aβ40 and Aβ42 can considerably clarify the role of plaque in AD pathology. Unfortunately, small-molecule probes with such capacity are scarce.7
Due to the small difference in the amino acid sequence of the peptides, discovering small-molecule probes capable of differentiating Aβ40 and Aβ42 has been considered as an impossible mission. In our previous studies, inspired by the binding principles of antibodies for soluble and insoluble Aβs, we designed a series of small fluorescent molecules to selectively detect soluble Aβs,8 the likely biomarker for the early stage of AD pathology. Encouraged by the antibody's capability to specifically recognize Aβ40 or Aβ42 peptides, we hypothesized that it could be possible to differentiate Aβ40 and Aβ42 with a small molecule probe. Our design strategy is different from most previous studies, which are focused on adjusting optical properties to turn on (off) signals or make larger stokes shifts.9–13 Unfortunately, few probes have been designed based on the insights from the Aβ structures. In this report, we demonstrated that our designed small-molecule fluorescence probe, ICTAD-1, has the capacity to spectrally differentiate Aβ40 and Aβ42 in vitro and in the biologically relevant environment. We believe that our strategy could provide a new path for designing Aβ probes.
Results
Design of fluorescent imaging probes
It is obvious that the C-terminal of Aβ peptide is the key for designing small-molecule probes to distinguish Aβ40 and Aβ42. However, this is extremely challenging, due to the difference in only two amino acids (isoleucine–alanine). Nonetheless, it has been routinely performed in numerous laboratories with anti-Aβ42 antibodies to determine the contents of Aβ42 in cell media and brain tissues.14–17 These anti-Aβ42 antibodies were designed based on the epitope of the C-terminal of the peptide. This fact has bolstered us to believe that the properties of the C-terminal can be relied on to design our small molecule probes. In the past, X-ray structures of full-length Aβs were rare.18–20 However, in recent years, several detailed structures of Aβ fibrils have been published. Particularly, the advanced cryoEM technology has impressively facilitated Aβ structure studies.21–23
After having carefully surveyed the published structures of Aβ fibrils, we found several potential binding sites for designing probes for Aβ fibrils. The sites are A site, which locates in the hydrophilic N-terminal portion, B site, the hydrophobic mid-region, and C site, which is formed by a hydrophobic C-terminal from one Aβ peptide and a hydrophilic N-terminal from another Aβ peptide (Fig. 1a). Numerous small molecules have been reported as ligands of Aβ40 and Aβ42 species, including several sub-species such as soluble oligomers and insoluble fibrils. However, nearly all of the compounds have been designed to interact with the B site, which is the middle region of Aβ peptides.8,10,24–35 Obviously, this is not the suitable region for designing probes to differentiate Aβ40 and Aβ42. Surprisingly, to the best of our knowledge, no compound has been intentionally designed or validated to target the C-terminal and the C site. Interestingly, we noticed that, for the site C in most cases, multiple pieces of the Aβ peptide forms a gulf, in which one side consists of hydrophilic amino acids (K28, D1), and another side is hydrophobic of V39, V40, I41, and A42 (isoleucine–alanine), the last two amino acids in Aβ42 (Fig. 1b and S1†).
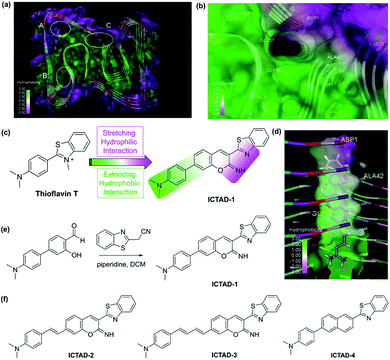 |
| Fig. 1 (a) The possible binding sites of Aβ42 protein (PDB: 5OQV); (b) binding site C of Aβ42 (PDB: 5OQV); (c) the design strategy of probe ICTAD-1; (d) the binding model of probe ICTAD-1 with Aβ42 protein (PDB: 5OQV), the red dashed lines note hydrogen bonds; (e) the synthetic route for probe ICTAD-1; (f) the structures of compounds ICTAD-2, ICTAD-3, and ICTAD-4. | |
This interesting feature of the C site requires its binding ligands to meet the following criteria: (1) although Aβ40 and Aβ42 only have two amino acids difference, their hydrophobicity is very distinct. It is well known that, compared to Aβ40, Aβ42 is much stickier and considerably easier to aggregate. This is the key feature for designing probes to distinguish them, and this key feature requires the designed probes contain a hydrophobic moiety to interact differently with the hydrophobic side of the C-site; (2) in addition to the hydrophobic moiety, the ligand needs to contain a hydrophilic moiety to interact with the polar side; (3) the designed probe has a different binding affinity to Aβ40 and Aβ42 fibrils; and (4) the probes should have distinct fluorescence spectra and/or binding strength in the presence of Aβ40 and Aβ42 fibrils.
In terms of the fluorescent properties of small-molecule fluorescent probes, numerous compounds showed blue-shifting (hypsochromic shifting) after interacting with Aβs, suggesting they bind to a hydrophobic environment. However, benzothiazole analogues are distinct because they showed fluorescence red-shifting (bathochromic shifting),36 indicating this moiety can bind to a polar environment. The most typical compound is thioflavin T, which has been used as a gold standard for Aβ plaque staining, and its derivative PIB has been widely used in the clinic as a PET tracer for imaging Aβ deposits.37–43 Thioflavin T provides the classic emission of spectral red-shifting. However, thioflavin T cannot spectrally differentiate the Aβ fibrils, likely due to its incapacity to differently interact with the hydrophobic moiety of the C-site. Nonetheless, we consider thioflavin T is a good starting point for probe designing.
Based on the above consideration, the designed probe should be tilted between the hydrophilic and hydrophobic moieties with a vertical arrangement (parallel to the fibril axis) (Fig. 1d), while thioflavin T is too short to touch the hydrophobic moiety and this is why it does not have the differentiating capacity. Conceivably, extending the length of the hydrophobic patch of thioflavin T has the potential to achieve this goal (Fig. 1c). In this regard, we designed ICTAD-1 (imino-coumarin–thiazole for AD), in which N,N-dimethyl-phenyl is for touching the hydrophobic moiety and for adjusting the binding affinity. In addition, iminocoumarin was introduced to stretch the hydrophilic moiety, which can enhance the binding through increasing hydrogen bonds (Fig. 1c). Moreover, the NH group in iminocoumarin could be served as a hydrogen donor to form an intramolecular hydrogen bond with benzothiazole moiety, leading to the enhanced planarity of the two heterocycles, which was distinctly different from the previously reported coumarin derivatives.
To validate this design strategy, molecular docking between ICTAD-1 and Aβs was performed. The molecular docking results ICTAD-1 with Aβ42 (PDB: 5OQV) showed that, indeed, the hydrophilic patch of C-site interacted with the stretched hydrophilic moiety of ICTAD-1, and the extended aromatic ring moiety interacted with the hydrophobic patch (Fig. 1d). The hydrophobic interaction between ICTAD-1 with residues ALA2 and ILE41 of Aβ42 was observed. The oxygen atom in iminocoumarin formed an intermolecular hydrogen bonding with the NH of GLY38. Moreover, the intramolecular hydrogen bond was also observed. The docking score for ICTAD-1 with Aβ42 at site C was −8.8476, which was lower than thioflavin T (−6.8242), indicating ICTAD-1 might have better binding affinity to Aβ42 than thioflavin T. Similar docking results of ICTAD-1 with other Aβ42 fibrillar structures (PDB: 5KK3 and 2NAO) were achieved (Fig. S2a and b†). We also carried out the docking studies of ICTAD-1 with Aβ40 fibrillar structures (PDB: 2MVX). The docking results showed ICTAD-1 could bind to the C site of Aβ40 through three intramolecular hydrogen bonds with residues HIS6 and GLU11 (Fig. S2c†). And it was observed that there were hydrophobic interactions between ICTAD-1 with residues PHE4 and VAL40. Moreover, the docking score of ICTAD-1 with Aβ40 was −8.2130, which was slightly lower than Aβ42. Similarly, ICTAD-1 could also dock into the C sites of other Aβ40 fibrils (PDB: 2MPZ and 6SHS) (Fig. S2d and e†). Considering the difference in the hydrophobic environment at sites C in Aβ40 and Aβ42, these results indicated that ICTAD-1 had the potential to bind and discriminate Aβ40 and Aβ42 fibrils.
To investigate whether the iminocoumarin moiety is necessary for binding, we designed ICTAD-2 by replacing it with a naphthalene ring. In addition, we designed ICTAD-3 and -4 to further extend the hydrophobic moiety to investigate whether the compounds can better match with the hydrophobic patch in the C-site. The synthesis of ICTAD-1 is straightforward, and its route is shown in Fig. 1e. The structures and synthetic routes for ICTAD-2, -3, and -4 are shown in Fig. 1f and ESI.†
Properties of ICTAD-1
With the probe in hand, we firstly performed the photostability experiments in DMF to investigate whether ICTAD-1 is stable under light (1500 joule per minute), and found that there were nearly no changes of fluorescence intensity after irradiating 120 minutes (Fig. 2a and S3a–d†), suggesting that ICTAD-1 has excellent stability to resist photobleaching. To explore ICTAD-1's responses towards different pH media, we titrated it within the range of pH 2–11, and found its absorbance intensity was consistent from pH 6–11. While we found that its fluorescence intensity decreased dramatically from pH 2–5, and its fluorescence is minimal under pH 7 (Fig. 2b and S4†), suggesting this probe has minimal fluorescence background signal and is suitable for in vivo imaging. With ICTAD-1 in hand, we also investigate its absorbance and emission spectra in different solvents. We found that the absorbance is much less dependent (<30 nm changes) on solvent polarity, while its emission could be drastically changed in different solvents, and about 150 nm difference could be found from non-polar hexane to glycol. As we expected, ICTAD-1 displayed much longer emission in polar solvents such as DMSO and glycol, indicating this probe can interact with a polar environment (Fig. 2c, d and S4†). Moreover, ICTAD-1 exhibited higher quantum yield in the nonpolar solvents, such as hexane and toluene (Table S1†).
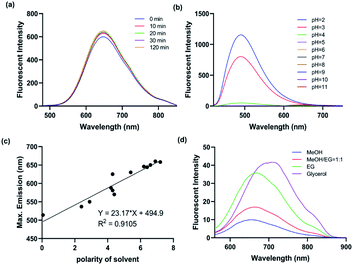 |
| Fig. 2 (a) The fluorescent spectra changes of ICTAD-1 (10 μM) in DMF under the irradiation of daylight lamp for 120 min; (b) the fluorescent spectra of ICTAD-1 (10 μM) in different pH aqueous solutions (1% DMF); (c) the linear relationship between the polarity of solvent and the maximum emission wavelength; (d) the fluorescence spectra of ICTAD-1 (10 μM) in solvents of different viscosity, EG: ethylene glycol. | |
Responses of ICTAD-1 to Aβ fibrils in solutions
To examine ICTAD-1's emission response to Aβ fibrils, we incubated 250 nM of the probe with different concentrations of Aβ40 and observed a consistent red-shifting of emission from 1.25 μM to 12.5 μM, suggesting the binding of ICTAD-1 with Aβs is not 1
:
1 stoichiometry (Fig. 3a). Meanwhile, apparent fluorescence intensity increases could be observed with different concentrations of Aβ40. The largest shift was 47 nm and the largest intensity increase was over 6-fold. We also observed that the fluorescence intensity increased with time and it reached a plateau within 20 minutes (Fig. S5c and d†). The turn-on effect of ICTAD-1 is likely due to twisted intramolecular charge transfer (TICT) upon binding to the fibrils, in which the planar configuration is preferred, and the rotation of the aromatic rings is restricted.33,44 From this study, we were also able to calculate a binding constant Kd = 6.27 μM for Aβ40, while Kd of Aβ42 was 3.78 μM, suggesting that the binding to Aβ40 fibrils was weaker than that for Aβ42 fibrils (Fig. 3a–d). In addition, we found that ICTAD-1 provided excellent linear correlations with the concentrations of Aβ40 and Aβ42 fibrils in the range of 0–4 μM (Fig. S5†).
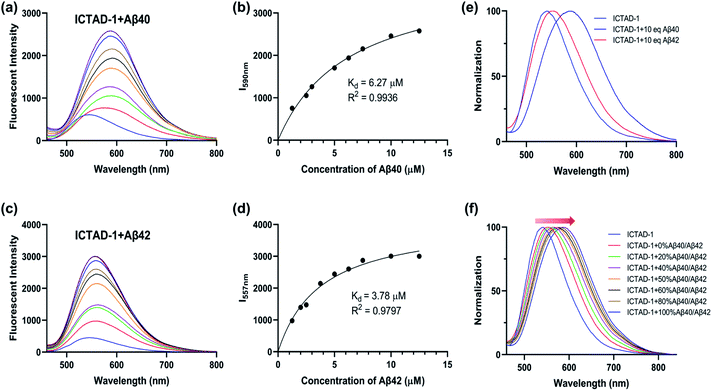 |
| Fig. 3 The fluorescent spectra of ICTAD-1 (250 nM) in the absence and presence of Aβ40 (a) or Aβ42 (c); the binding curve of ICTAD-1 (250 nM) with Aβ40 (b) or Aβ42 (d); (e) normalization fluorescent spectra of ICTAD-1 (250 nM) with Aβ40 or Aβ42 (10 eq., 2.5 μM); (f) normalization fluorescent spectra of ICTAD-1 (250 nM) with different percent of Aβ40 in Aβ42 (10 eq., 2.5 μM). | |
We then performed similar experiments to study the emission changes of ICTAD-1 with Aβ42 fibrils and found consistent red-shifts. Remarkably, we observed that the degrees of emission peak shifting were different, and Aβ40 provided much larger red-shifting (Fig. 3e and f). This is expected because Aβ42 provided tight binding due to its higher hydrophobicity and crowed space, while Aβ40 is less crowded and provides loose binding. This is consistent with ICTAD-1's binding constants for Aβ40/42. Interestingly, in the solutions of Aβ40/42 mixture, we found that the normalized spectral peaks shifted from shorter wavelengths (blue) to longer wavelengths (red) with the increasing ratio of Aβ40 component, and the shifted wavelength number was linear to the ratio of Aβ40/42 (Fig. S6†). In addition, we found that ICTAD-1 had excellent selectivity over metal ions and other proteins, such as bovine serum albumin (BSA) and human serum albumin (HSA) (Fig. S7†).
To examine whether a naphthalene ring can be used to replace the iminocoumarin moiety in ICTAD-1, we performed similar solution tests with ICTAD-2, and found that ICTAD-2 provided a slight red-shifting and a slight intensity decrease (Fig. S8a†), suggesting the hydrophilic iminocoumarin moiety is necessary for binding to the hydrophilic patch in Aβ fibrils. Different from ICTAD-1, ICTAD-3 showed no apparent red-shifting, while it showed a slight intensity increase (Fig. S8b†). ICTAD-4 showed an observable blue-shifting and a moderate intensity increase (Fig. S8c†). Since ICTAD-2, -3, and -4 didn't have favorable properties for differentiating Aβ40/42, we didn't perform further investigation for these compounds.
Differentiating Aβ40 and Aβ42 with ICTAD-1via spectral unmixing imaging
To explore whether ICTAD-1 can be used to quantify the amount of Aβ40 and Aβ42 fibrils, spectral unmixing with mixtures of these fibrils was performed (Fig. S9†). First, we used pure Aβ40 and Aβ42 fibrils solutions to establish the spectra on a 96-well plate. The linear relationships could be observed for both fibrils (Fig. S10†), suggesting that spectral unmixing is feasible to quantify the contribution of each component in the mixture. The detection limitations are 3.3 nM and 28.3 nM for Aβ40 fibrils and Aβ42 fibrils respectively. Then we conducted library-based spectral unmixing to separate the ratios of these two fibrils. Indeed, we found that it was feasible to deconvolute the signals with the characteristic spectra of free ICTAD-1 (green), ICTAD-1 with Aβ40 fibrils (red), and Aβ42 fibrils (blue) (Fig. 4a and b).
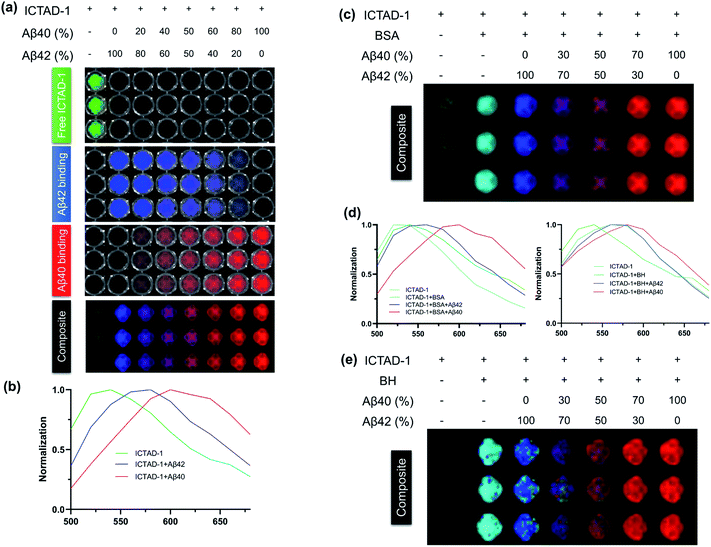 |
| Fig. 4 (a) Plate imaging of ICTAD-1 (250 nM) in the presence of different ratios of Aβ40/Aβ42 (2.5 μM, 10 eq.) in 96-wells plate after unmixing. Ex = 430 nm, Em = 500–680 nm. (b) Normalized unmixing spectra of ICTAD-1 (250 nM) with or without Aβ40 and Aβ42; (c) plate imaging of ICTAD-1 (250 nM) in the presence of different ratios of Aβ40/Aβ42 (2.5 μM, 10 eq.) in 96-wells plate after unmixing in the presence of BSA; (d) normalized unmixing spectra of ICTAD-1 (250 nM) with or without Aβ40 and Aβ42 in the presence of BSA or mouse brain homogenate (BH); (e) plate imaging of ICTAD-1 (250 nM) in the presence of different ratios of Aβ40/Aβ42 (2.5 μM, 10 eq.) in 96-wells plate after unmixing in the presence of mouse brain homogenate. | |
Next, to investigate whether spectral unmixing is feasible in a biologically relevant environment, we performed the above experiments in the presence of bovine serum albumin (BSA) and mouse brain homogenate (BH). As we expected, spectral unmixing could be used to deconvolute the signals from the free probe (green), binding with BSA or BH (cyan), binding with Aβ40 (red) and Aβ42 (blue) (Fig. 4c–e), suggesting it is possible to spectrally differentiate the Aβ fibrils in biologically relevant environments.
Tissue staining and spectral unmixing with ICTAD-1
We first explored whether ICTAD-1 could provide a high quality of plaque staining, and found that plaques on brain slides of 5xFAD mice can be sharply visualized after the slide was incubated with the probe for 15 minutes (Fig. 5a), and the labeled plaque showed excellent colocalization with 6E10 antibody staining (Fig. 5a), which is specific to Aβs. This result suggested that ICTAD-1 was able to specifically label Aβ plaques.
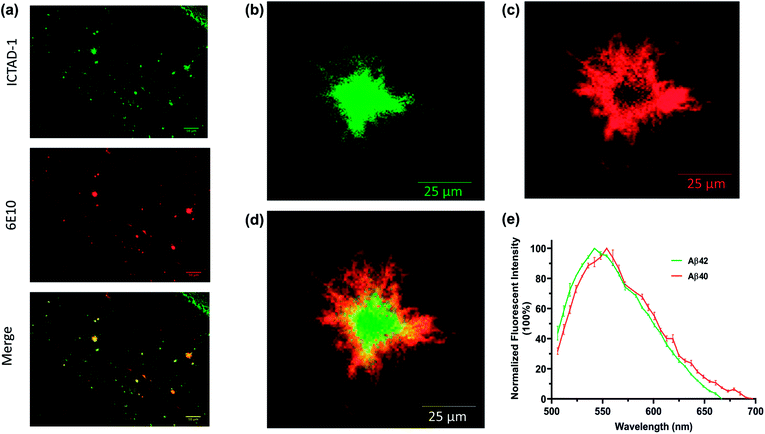 |
| Fig. 5 (a) The brain tissues from 5xFAD mice were co-stained with ICTAD-1 and antibody 6E10; (b) the core of Aβ plaque stained with ICTAD-1; (c) the periphery of Aβ plaque stained with ICTAD-1; (d) merged image of (b) and (c); (e) the normalized unmixing fluorescent spectra of Aβ plaques stained with ICTAD-1 (n = 3). | |
The previous results inspired us to explore the capability of ICTAD-1 in differentiating Aβ40 and Aβ42 fibrils in the plaques. Then we relied on confocal imaging that has been equipped with a spectral unmixing function. Interestingly, the spectra from the core most closely resembled the one for Aβ42 in vitro studies, whose peak wavelength was shorter. Meanwhile, the spectra from the periphery were similar to the one from Aβ40 fibrils in vitro, whose peak has a longer wavelength (Fig. 5b–e and S11†). The peak wavelength difference from the two spectra was 12 nm (Fig. 5e). Reportedly, Aβ42 is the dominant species in the core of plaques,45–48 which is consistent with our spectral unmixing results. Taken together, these results suggested that ICTAD-1 could be used to quantify the contents of Aβ42/Aβ40 from brain slides. This also indicates that it is possible to quantify the subspecies in human brain tissue, which is very important to clarify the contributions of Aβ42 fibrils to the AD pathology.
In vivo two-photon imaging with ICTAD-1
To validate whether ICTAD-1 can be used for in vivo imaging, we performed two-photon microscopic imaging with 15 month old 5xFAD mice. We first intravenously (iv) injected the mouse with FITC–dextran as the contrast agent for blood vessels. After 5 minutes, we iv injected ICTAD-1 (1 mg kg−1), and imaged the mouse at 5 minutes post-injection of ICTAD-1. As expected, the plaques can be easily identified, due to the excellent contrast (Fig. 6a–c), suggesting that the probe can cross the brain–blood barrier (BBB) and can label plaques in vivo. Interestingly, we experienced some difficulties to identify cerebral amyloid angiopathy (CAA) on the vessels, which can be found from its autofluorescence (blue in Fig. 6d and e). These results indicated that ICTAD-1 has weak capacities to label CAAs, in which Aβ40 is the dominant species.49,50 This result could be possibly explained with our in vitro Kd data, which indicated that ICTAD-1 had much stronger binding to Aβ42, while it showed weaker binding to Aβ40. This in vivo data also suggested that differentiating Aβ40 and Aβ42 in vivo via different binding strength (Kd) was feasible.
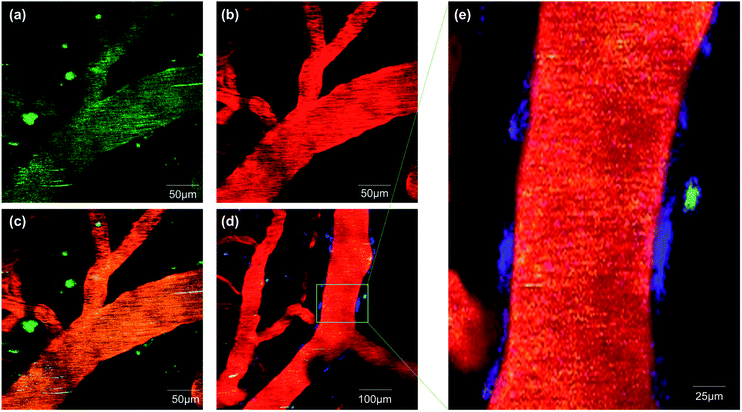 |
| Fig. 6 Two-photon fluorescent images in 5xFAD mice after tail intravenous injected with ICTAD-1 and FITC–dextran. (a) The fluorescence from ICTAD-1 in emission channel of 570–620 nm; (b) the fluorescence from FITC–dextran in emission channel of 500–550 nm; (c) the merged image of (a) and (b); (d) fluorescence imaging of Aβ plaques, CAA and blood vessel; (e) partial enlarged detail of (d). | |
Discussion
Aβ plaques have been discovered for more than 100 years, however, their roles in AD pathology are still poorly defined. The complicated dynamics and contributions of each component are the likely factors for the perplex. It is well documented that Aβ40 and Aβ42 are the major components of the plaques. Nonetheless, it is not clear what is the contribution weight of each Aβ subspecies to the pathology, and whether the content of Aβ42 in plaques has better correlations with the severity of the disease. With our probe, it is possible to partially solve the mysteries, and we may have the capacity to quantify how much Aβ42 is in each plaque, each brain slide, and specific brain areas. Conceivably, it is also possible to quantify Aβ42 contents for a whole-brain through serial slide imaging. With such a tool, we will be well equipped to answer those basic mechanism questions and will clarify the questions around postmortem plaques, which will provide more diagnostic information.
Thioflavin T reportedly showed slight difference of fluorescence lifetimes in pure solutions for Aβ40 and Aβ42 fibrils,51 however, it remains unknown whether the differences can be applied under physiological conditions. Kung et al.52 reported that radioligand [125I]DMTZ had certain preference for Aβ42-positive cerebral amyloid angiopathy (CAA). However, it is not clear whether it has the capacity to differentiate Aβ40/42 in the plaques.
In this report, we also demonstrated that ICTAD-1 could be used to separate Aβ40/42 using spectral properties in vitro and binding strength in vivo. The spectral unmixing is easy to perform on a regular confocal microscope, suggesting our method can be easily adapted by other biological-oriented laboratories. From the slide spectral unmixing, the separation of emission peaks of Aβ40/42 was 12 nm, which may indicate that the separation of Aβ40/42 is imperfect. However, it is possible to achieve a cleaner separation (a larger difference in the emission peak) if a better algorithm is developed.
The difference of Kd for Aβ40/42 could be utilized for designing PET tracers that have much high specificity for Aβ42, the most toxic species. Our in vivo two-photon imaging indicated that ICTAD-1 had certain binding preference towards Aβ42, and this could be considered as clues for designing for Aβ42 specific PET tracers. In addition, it is also tremendously important if we can differentiate CAA and plaques with a PET tracer, which is not currently available. Reportedly, Aβ40 is the major component of CAAs.49,50 Our data from this report suggests that our probe and its analogues may have the potential to differentiate plaques and CAAs. With such a probe, we will be able to dissect the vascular CAA contribution and plaque contribution to AD pathology.
Our studies also pointed to several possible directions for future AD research. First, we may have the capacity to monitor therapy that is prone to reduce Aβ42. Second, it is possible to design drugs that specifically target Aβ42. Lastly, we will be able to investigate whether the specific reduction of Aβ42 is a validated approach for future drug development.
Conclusions
In summary, we demonstrated that ICTAD-1 was able to spectrally differentiate Aβ40/42 in solutions, in plasma and in brain tissues. ICTAD-1 has the potential to dissect the toxicity contributions of Aβ40 and Aβ42, and could be considered as a lead compound for developing Aβ42 specific PET tracers.
Ethical statement
All animal experiments were performed in strict accordance with the NIH guidelines for the care and use of laboratory animals (NIH Publication No. 85-23 Rev. 1985) and was approved by the Institutional Animal Care and Use Committee at Massachusetts General Hospital.
Conflicts of interest
There are no conflicts to declare.
Acknowledgements
This work was supported by NIH R01AG055413, R21AG065826 and R21AG059134 awards (C. R.). The authors would like to thank Pamela Pantazopoulos, B. S. for proofreading this manuscript.
Notes and references
- J. Hardy and D. J. Selkoe, Science, 2002, 297, 353–356 CrossRef CAS PubMed.
- M. Goedert and M. G. Spillantini, Science, 2006, 314, 777–781 CrossRef CAS PubMed.
- C. A. McLean, R. A. Cherny, F. W. Fraser, S. J. Fuller, M. J. Smith, K. Beyreuther, A. I. Bush and C. L. Masters, Ann. Neurol., 1999, 46, 860–866 CrossRef CAS PubMed.
- L. F. Lue, Y. M. Kuo, A. E. Roher, L. Brachova, Y. Shen, L. Sue, T. Beach, J. H. Kurth, R. E. Rydel and J. Rogers, Am. J. Pathol., 1999, 155, 853–862 CrossRef CAS PubMed.
- R. Terry, E. Masliah, D. P. Salmon, N. Butters, R. DeTeresa, R. Hill, L. A. Hansen and R. Katzman, Ann. Neurol., 1991, 30, 572–580 CrossRef CAS PubMed.
- S. L. Bernstein, N. F. Dupuis, N. D. Lazo, T. Wyttenbach, M. M. Condron, G. Bitan, D. B. Teplow, J. E. Shea, B. T. Ruotolo, C. V. Robinson and M. T. Bowers, Nat. Chem., 2009, 1, 326–331 CrossRef CAS PubMed.
- T. Qiu, Q. Liu, Y. X. Chen, Y. F. Zhao and Y. M. Li, J. Pept. Sci., 2015, 21, 522–529 CrossRef CAS PubMed.
- Y. Li, J. Yang, H. Liu, J. Yang, L. Du, H. Feng, Y. Tian, J. Cao and C. Ran, Chem. Sci., 2017, 8, 7710–7717 RSC.
- Y. W. Jun, S. W. Cho, J. Jung, Y. Huh, Y. Kim, D. Kim and K. H. Ahn, ACS Cent. Sci., 2019, 5, 209–217 CrossRef CAS PubMed.
- W. Fu, C. Yan, Z. Guo, J. Zhang, H. Zhang, H. Tian and W. H. Zhu, J. Am. Chem. Soc., 2019, 141, 3171–3177 CrossRef CAS PubMed.
- M. Staderini, M. A. Martin, M. L. Bolognesi and J. C. Menendez, Chem. Soc. Rev., 2015, 44, 1807–1819 RSC.
- A. Aliyan, N. P. Cook and A. A. Marti, Chem. Rev., 2019, 119, 11819–11856 CrossRef CAS PubMed.
- X. Zhang and C. Ran, Curr. Org. Chem., 2013, 17, 580–593 CrossRef CAS PubMed.
- Y. Levites, P. Das, R. W. Price, M. J. Rochette, L. A. Kostura, E. M. McGowan, M. P. Murphy and T. E. Golde, J. Clin. Invest., 2006, 116, 193–201 CrossRef CAS PubMed.
- A. Hatami, R. Albay III, S. Monjazeb, S. Milton and C. Glabe, J. Biol. Chem., 2014, 289, 32131–32143 CrossRef CAS PubMed.
- L. Richter, L. M. Munter, J. Ness, P. W. Hildebrand, M. Dasari, S. Unterreitmeier, B. Bulic, M. Beyermann, R. Gust, B. Reif, S. Weggen, D. Langosch and G. Multhaup, Proc. Natl. Acad. Sci. U. S. A., 2010, 107, 14597–14602 CrossRef CAS PubMed.
- J. Dunys, A. Valverde and F. Checler, J. Biol. Chem., 2018, 293, 15419–15428 CrossRef CAS PubMed.
- J. X. Lu, W. Qiang, W. M. Yau, C. D. Schwieters, S. C. Meredith and R. Tycko, Cell, 2013, 154, 1257–1268 CrossRef CAS PubMed.
- A. K. Schutz, T. Vagt, M. Huber, O. Y. Ovchinnikova, R. Cadalbert, J. Wall, P. Guntert, A. Bockmann, R. Glockshuber and B. H. Meier, Angew. Chem., Int. Ed., 2015, 54, 331–335 CrossRef PubMed.
- M. A. Walti, F. Ravotti, H. Arai, C. G. Glabe, J. S. Wall, A. Bockmann, P. Guntert, B. H. Meier and R. Riek, Proc. Natl. Acad. Sci. U. S. A., 2016, 113, E4976–E4984 CrossRef CAS PubMed.
- L. Gremer, D. Scholzel, C. Schenk, E. Reinartz, J. Labahn, R. B. G. Ravelli, M. Tusche, C. Lopez-Iglesias, W. Hoyer, H. Heise, D. Willbold and G. F. Schroder, Science, 2017, 358, 116–119 CrossRef CAS PubMed.
- M. Schmidt, A. Rohou, K. Lasker, J. K. Yadav, C. Schiene-Fischer, M. Fandrich and N. Grigorieff, Proc. Natl. Acad. Sci. U. S. A., 2015, 112, 11858–11863 CrossRef CAS PubMed.
- M. Kollmer, W. Close, L. Funk, J. Rasmussen, A. Bsoul, A. Schierhorn, M. Schmidt, C. J. Sigurdson, M. Jucker and M. Fandrich, Nat. Commun., 2019, 10, 4760 CrossRef PubMed.
- C. Ran, X. Xu, S. B. Raymond, B. J. Ferrara, K. Neal, B. J. Bacskai, Z. Medarova and A. Moore, J. Am. Chem. Soc., 2009, 131, 15257–15261 CrossRef CAS PubMed.
- X. Zhang, Y. Tian, Z. Li, X. Tian, H. Sun, H. Liu, A. Moore and C. Ran, J. Am. Chem. Soc., 2013, 135, 16397–16409 CrossRef CAS PubMed.
- X. Zhang, Y. Tian, C. Zhang, X. Tian, A. W. Ross, R. D. Moir, H. Sun, R. E. Tanzi, A. Moore and C. Ran, Proc. Natl. Acad. Sci. U. S. A., 2015, 112, 9734–9739 CrossRef CAS PubMed.
- M. L. Bolognesi, A. Gandini, F. Prati and E. Uliassi, J. Med. Chem., 2016, 59, 7759–7770 CrossRef CAS PubMed.
- R. Jakob-Roetne and H. Jacobsen, Angew. Chem., Int. Ed., 2009, 48, 3030–3059 CrossRef CAS PubMed.
- W. M. Chang, M. Dakanali, C. C. Capule, C. J. Sigurdson, J. Yang and E. A. Theodorakis, ACS Chem. Neurosci., 2011, 2, 249–255 CrossRef CAS PubMed.
- S. R. Choi, G. Golding, Z. Zhuang, W. Zhang, N. Lim, F. Hefti, T. E. Benedum, M. R. Kilbourn, D. Skovronsky and H. F. Kung, J. Nucl. Med., 2009, 50, 1887–1894 CrossRef CAS PubMed.
- M. Cui, M. Ono, H. Watanabe, H. Kimura, B. Liu and H. Saji, J. Am. Chem. Soc., 2014, 136, 3388–3394 CrossRef CAS PubMed.
- C. L. Teoh, D. Su, S. Sahu, S. W. Yun, E. Drummond, F. Prelli, S. Lim, S. Cho, S. Ham, T. Wisniewski and Y. T. Chang, J. Am. Chem. Soc., 2015, 137, 13503–13509 CrossRef CAS PubMed.
- J. Shin, P. Verwilst, H. Choi, S. Kang, J. Han, N. H. Kim, J. G. Choi, M. S. Oh, J. S. Hwang, D. Kim, I. Mook-Jung and J. S. Kim, Angew. Chem., Int. Ed., 2019, 58, 5648–5652 CrossRef CAS PubMed.
- A. C. Sedgwick, W. T. Dou, J. B. Jiao, L. Wu, G. T. Williams, A. T. A. Jenkins, S. D. Bull, J. L. Sessler, X. P. He and T. D. James, J. Am. Chem. Soc., 2018, 140, 14267–14271 CrossRef CAS PubMed.
- K. J. Cao and J. Yang, Chem. Commun., 2018, 54, 9107–9118 RSC.
- A. A. Maskevich, V. I. Stsiapura, V. A. Kuzmitsky, I. M. Kuznetsova, O. I. Povarova, V. N. Uversky and K. K. Turoverov, J. Proteome Res., 2007, 6, 1392–1401 CrossRef CAS PubMed.
- H. LeVine III, Methods Enzymol., 1999, 309, 274–284 Search PubMed.
- H. Naiki, K. Higuchi, M. Hosokawa and T. Takeda, Anal. Biochem., 1989, 177, 244–249 CrossRef CAS PubMed.
- C. A. Mathis, Y. Wang, D. P. Holt, G. F. Huang, M. L. Debnath and W. E. Klunk, J. Med. Chem., 2003, 46, 2740–2754 CrossRef CAS PubMed.
- W. E. Klunk, H. Engler, A. Nordberg, Y. Wang, G. Blomqvist, D. P. Holt, M. Bergstrom, I. Savitcheva, G. F. Huang, S. Estrada, B. Ausen, M. L. Debnath, J. Barletta, J. C. Price, J. Sandell, B. J. Lopresti, A. Wall, P. Koivisto, G. Antoni, C. A. Mathis and B. Langstrom, Ann. Neurol., 2004, 55, 306–319 CrossRef CAS PubMed.
- M. Biancalana and S. Koide, Biochim. Biophys. Acta, 2010, 1804, 1405–1412 CrossRef CAS PubMed.
- K. Rajasekhar, N. Narayanaswamy, N. A. Murugan, G. Kuang, H. Agren and T. Govindaraju, Sci. Rep., 2016, 6, 23668 CrossRef CAS PubMed.
- T. Bussiere, F. Bard, R. Barbour, H. Grajeda, T. Guido, K. Khan, D. Schenk, D. Games, P. Seubert and M. Buttini, Am. J. Pathol., 2004, 165, 987–995 CrossRef CAS PubMed.
- Z. R. Grabowski, K. Rotkiewicz and W. Rettig, Chem. Rev., 2003, 103, 3899–4032 CrossRef PubMed.
- H. Welander, J. Franberg, C. Graff, E. Sundstrom, B. Winblad and L. O. Tjernberg, J. Neurochem., 2009, 110, 697–706 CrossRef CAS PubMed.
- A. Tamaoka, T. Kondo, A. Odaka, N. Sahara, N. Sawamura, K. Ozawa, N. Suzuki, S. Shoji and H. Mori, Biochem. Biophys. Res. Commun., 1994, 205, 834–842 CrossRef CAS PubMed.
- D. M. Mann, T. Iwatsubo, Y. Ihara, N. J. Cairns, P. L. Lantos, N. Bogdanovic, L. Lannfelt, B. Winblad, M. L. Maat-Schieman and M. N. Rossor, Am. J. Pathol., 1996, 148, 1257–1266 CAS.
- S. Kumar-Singh, P. Cras, R. Wang, J. M. Kros, J. van Swieten, U. Lubke, C. Ceuterick, S. Serneels, K. Vennekens, J. P. Timmermans, E. Van Marck, J. J. Martin, C. M. van Duijn and C. Van Broeckhoven, Am. J. Pathol., 2002, 161, 507–520 CrossRef CAS PubMed.
- D. L. Miller, I. A. Papayannopoulos, J. Styles, S. A. Bobin, Y. Y. Lin, K. Biemann and K. Iqbal, Arch. Biochem. Biophys., 1993, 301, 41–52 CrossRef CAS PubMed.
- S. A. Gravina, L. Ho, C. B. Eckman, K. E. Long, L. Otvos Jr, L. H. Younkin, N. Suzuki and S. G. Younkin, J. Biol. Chem., 1995, 270, 7013–7016 CrossRef CAS PubMed.
- D. J. Lindberg, M. S. Wranne, M. Gilbert Gatty, F. Westerlund and E. K. Esbjörner, Biochem. Biophys. Res. Commun., 2015, 458, 418–423 CrossRef CAS PubMed.
- M.-P. Kung, D. M. Skovronsky, C. Hou, Z.-P. Zhuang, T. L. Gur, B. Zhang, J. Q. Trojanowski, V. M. Lee and H. F. Kung, J. Mol. Neurosci., 2003, 20, 15–23 CrossRef CAS PubMed.
Footnotes |
† Electronic supplementary information (ESI) available. See DOI: 10.1039/d0sc02060e |
‡ J. Yang and B. Zhu contributed equally. |
|
This journal is © The Royal Society of Chemistry 2020 |