DOI:
10.1039/D0RA07986C
(Paper)
RSC Adv., 2020,
10, 40055-40067
Facile route to synthesize Fe3O4@acacia–SO3H nanocomposite as a heterogeneous magnetic system for catalytic applications†
Received
18th September 2020
, Accepted 19th October 2020
First published on 4th November 2020
Abstract
In this work, a novel catalytic system for facilitating the organic multicomponent synthesis of 9-phenyl hexahydroacridine pharmaceutical derivatives is reported. Concisely, this catalyst was constructed from acacia gum (gum arabic) as a natural polymeric base, iron oxide magnetic nanoparticles (Fe3O4 NPs), and sulfone functional groups on the surface as the main active catalytic sites. Herein, a convenient preparation method for this nanoscale composite is introduced. Then, essential characterization methods such as various spectroscopic analyses and electron microscopy (EM) were performed on the fabricated nano-powder. The thermal stability and magnetic properties were also precisely monitored via thermogravimetric analysis (TGA) and vibrating-sample magnetometry (VSM) methods. Then, the performance of the presented catalytic system (Fe3O4@acacia–SO3H) was further investigated in the referred organic reaction by using various derivatives of the components involved in the reaction. Optimization, mechanistic studies, and reusability screening were carried out for this efficient catalyst as well. Overall, remarkable reaction yields (94%) were obtained for the various produced derivatives of 9-phenyl hexahydroacridine in the indicated optimal conditions.
1. Introduction
Recently, powder technology has received much attention in heterogeneous catalytic systems; powders have great potential to be applied in complex organic synthesis reactions and are conveniently separated during the purification processes through their heterogeneity.1,2 One of the best-known members of this family of materials is magnetic nanoscale powder, which has been widely used for various scientific purposes such as drug delivery,3 disease diagnosis,4 water desalination,5 environment cleaning,6 and chemical catalysis.7 In our previous work, we have reported several different catalytic systems constructed with the individual iron oxide (Fe3O4) powder (in nanoscale) and applied them in various catalytic processes.8–15 These nanoparticles could also be composed of other fibrous materials and could be immobilized into polymeric matrices. Through this method, the general properties of the catalytic systems such as the physicomechanical features of the individual Fe3O4 powder are improved. In this regard, numerous studies have been performed, and it has been revealed that the efficiency of the Fe3O4 powder can be significantly modified through its composition with other materials.16–20 For instance, a composition of graphene oxide, Fe3O4 and silver nanoparticles was prepared and applied for enhanced photocatalytic degradation of phenols in the past year.21 Moreover, Javanbakht et al. composited magnetic nanoparticles with a chitosan matrix for the efficient removal of lead(II) from water resources.22 Here, we attempted to prepare a suitable composite of Fe3O4 nanoparticles and “acacia gum” powder, and we applied this composition to facilitate the organic synthesis of 9-phenyl hexahydroacridine (HHA) pharmaceuticals.
Acacia gum, also called “gum arabic”, is obtained from wild trees, and its main origin is Somalia. From the organic chemical aspect, there are several hydroxyl groups in the structure of this polymer that can be used as appropriate sites for covalent binding and catalytic applications.23 From the physicomechanical aspect, the suitable stability of acacia gum led us to apply it as an appropriate substrate for immobilization of magnetic nanoparticles. Previously, acacia gum was used as a matrix for catalytic systems. For instance, Banerjee and Chen used this polymer to design a nanoscale absorbent system for the removal of copper ions from water resources. They also magnetized acacia gum through the composition of Fe3O4 NPs for an easy separation from the mixture.24 In this work, we attempted to perform a chemical modification of acacia gum by sulfonation of the hydroxyl functional groups and use the product as an acidic catalytic system for the facilitated synthesis of HHA derivatives. The chemical structure of the acacia gum polymer is presented in Fig. 1(a).
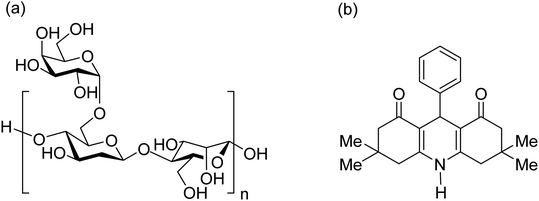 |
| Fig. 1 (a) Chemical structure of the acacia gum polymer and (b) general structure of tetramethyl-9-phenyl-hexahydroacridine-1,8(2H,5H)-dione. | |
To date, various types of hydroacridine derivatives have been developed and investigated for their therapeutic properties. For example, it has been revealed that 5,6-dihydroacridine derivatives possess antidiabetic and antioxidant properties.25,26 Therefore, it is highly important to prepare appropriate conditions for fast and direct synthesis of hydroacridine derivatives. Generally, HHAs and a wide spectrum of active pharmaceutical ingredients (APIs) are synthesized via multicomponent coupling reactions. Today, to obtain purer products with high reaction yields and to shorten the reaction time, many strategies are being introduced and applied. One of the most effective strategies is to use heterogeneous metallic catalytic systems.27–29 Briefly, through the existence of heteroatoms in the structure of the preliminary reactants of multicomponent reactions, constructive electronic interactions provide suitable conditions for chemical bonding. In this regard, sulfonated polymeric networks appear to be efficient for catalysis of organic synthesis reactions. For instance, a polymer-impregnated sulfonated carbon composite was recently reported as an acidic catalytic system for assisting the alkylation of phenol.30 In this study, our aim was to sulfonate acacia gum and apply it to facilitate the multicomponent synthesis reactions of tetramethyl-9-phenyl-hexahydroacridine-1,8(2H,5H)-dione. The general structure of these pharmaceuticals is presented in Fig. 1(b).
Concisely, we introduce a convenient method to synthesize an Fe3O4@acacia–SO3H heterogeneous magnetic catalytic system. Then, it is clearly shown that the synthesis of HHA derivatives is highly facilitated through applying this efficient catalyst. 87–94% reaction yields were obtained for different derivatives of HHA in reaction times of less than two hours. Moreover, convenient separation and excellent reusability were observed for this system through its magnetic properties.
2. Results and discussion
2.1. Preparation method of the Fe3O4@acacia–SO3H nano-powder
As presented in Fig. 2, iron(II) and iron(III) chloride salts were dissolved in deionized water at room temperature. Then, acacia gum powder was added and was also dissolved. In the next stage, iron ions were precipitated via co-deposition and produced Fe3O4 nanoparticles, which were well composed with the polymeric texture of acacia.31 Via this in situ method, a better composition was obtained, and the dark particles of Fe3O4 were well immobilized. For this purpose, ammonia solution was used to raise the pH value. Moreover, after separation and drying of the precipitate, the particles of Fe3O4@acacia were dispersed in chloroform and the temperature was reduced by an ice bath. Due to the exothermic reaction of sulfonic acid, gentle addition of this material at cool temperatures is required. During the preparation process, the Fe3O4 nanoparticles appear to electrostatically combine with the acacia textures because both species contain several hydroxyl groups in their chemical structures. In the case of sulfone groups, they are most likely covalently attached to the acacia and Fe3O4 nanoparticles.32 In the next stage, after completion of the addition of sulfonic acid and stirring for 120 min, the particles of Fe3O4@acacia–SO3H composite were magnetically separated, washed, and dried in an oven.
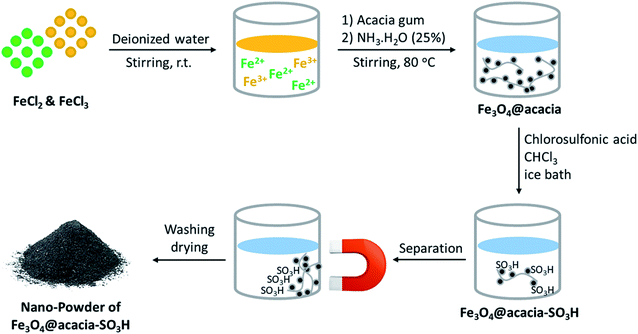 |
| Fig. 2 Schematic of the preparation route of the Fe3O4@acacia–SO3H nano-powder. | |
2.2. Characterization of the Fe3O4@acacia–SO3H nano-powder
2.2.1. FT-IR and EDX studies. To investigate the presence of essential functional groups in the structure of the Fe3O4@acacia–SO3H nano-powder, Fourier-transform infrared (FT-IR) spectra of the neat acacia gum (spectrum i), Fe3O4@acacia binary composite (spectrum ii), and Fe3O4@acacia–SO3H nano-powder (spectrum iii) were acquired and are presented in Fig. 3(a). As can be seen in the spectra, the presence of the O–H, C–H (hybridation sp3), and C–O bands was confirmed by the peaks appearing at 3400, 2929, 1050 and 1250 cm−1, respectively. Also, it appears that some of the hydroxyl groups in the structure of the acacia gum were converted to C
O. This claim is proven by the peak that appeared at ∼1660 cm−1 in the spectrum (i). The composition of the Fe3O4 NPs was confirmed by the peak appearing at ∼590 cm−1 in the spectrum (ii), which is related to the Fe–O bond. As observed in the spectrum (ii), the sharp broad peak of hydroxyl groups in the structure of acacia gum became deformed; this result may be due to the physicochemical composition of the Fe3O4 NPs. According to literature, the peak related to the S
O bond is appeared in the range of 1000–1200 cm−1. Accordingly, as shown in the spectrum (iii), this peak appeared and confirmed the successful sulfonation of the Fe3O4@acacia binary composite. To obtain more confirmation of the successful execution of the sulfonation process, energy-dispersive X-ray (EDX) analysis was also performed. As Fig. 3(b) shows, 5.3% of the total weight of the Fe3O4@acacia–SO3H nanocomposite was formed of sulfur after carrying out the sulfonation process. The existence of the other essential elements, such as carbon, oxygen, and iron, related to the desired structures of the Fe3O4@acacia binary composite and Fe3O4@acacia–SO3H nano-powder are also proven by EDX analysis.
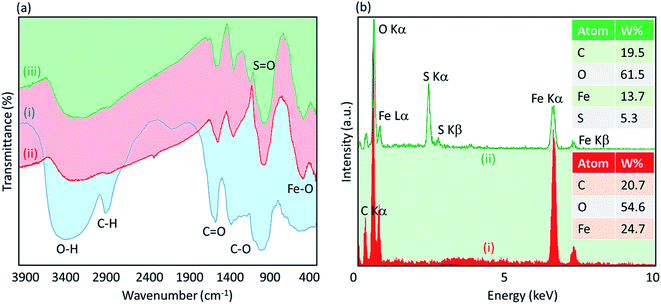 |
| Fig. 3 (a) Fourier-transform infrared spectra of (i) the neat acacia gum, (ii) Fe3O4@acacia binary composite, and (iii) Fe3O4@acacia–SO3H nano-powder; (b) energy-dispersive X-ray spectra of (i) the Fe3O4@acacia binary composite and (ii) the fabricated Fe3O4@acacia–SO3H nano-powder. | |
2.2.2. TGA and VSM studies. To check thermal stability of our prepared Fe3O4@acacia–SO3H nano-powder, thermogravimetric analysis (TGA) was performed in a thermal range of 0–3500 °C (Fig. 4(a)). This method also gives some information about the combination of the Fe3O4 NPs and the sulfonated acacia via monitoring of the decomposition process. For the Fe3O4@acacia binary composite (curve i), it can be clearly observed that proportional to the temperature rise, two distinct shoulders in the thermal ranges of 0–700 °C and 800–1700 °C appeared; then, the weight percentage gradually decreased from ∼1700 °C onwards. The first shoulder can be related to the dehydroxylation process of the acacia gum. Reportedly, the organic layers and the hydroxyl groups are separated from the structure as hydrate molecules up to 700 °C.33 In the next stage, in which ∼55% of the total weight was lost, the acacia gum likely decomposed and the individual Fe3O4 NPs started to collapse from ∼1700 °C. In curve (ii), which belongs to the fabricated Fe3O4@acacia–SO3H nano-powder, it can be clearly observed that the stability of the organic functional groups was significantly enhanced and the dehydroxylation process was prolonged to ∼1500 °C instead of 700 °C. Then, the decomposition process started from ∼1600 °C, and the weight was gradually reduced. It can also be seen that only 40% of the total weight was lost up to 3500 °C; this indicates that the general stability of the fabricated nano-powder was enhanced via the composition process. The magnetic property of the desired product was also studied by vibrating-sample magnetometry (VSM), and a comparison was made with the Fe3O4@acacia binary composite through their magnetic-hysteresis (M–H) curves (Fig. 4(b)). As shown, the magnetic property of the Fe3O4@acacia binary composite (curve i) decreased slightly (∼4.0 emu g−1) after performing the sulfonation process. The most probable reason is removal of some of the Fe3O4 magnetic NPs that were not strongly attached to the polymeric fibers during the sulfonation process. However, magnetic saturation for the fabricated Fe3O4@acacia–SO3H nano-powder occurred at ∼23.5 emu g−1 by applying a magnetic field with 10
000 (Oe) power, and this value is enough to perform a convenient magnetic separation process.
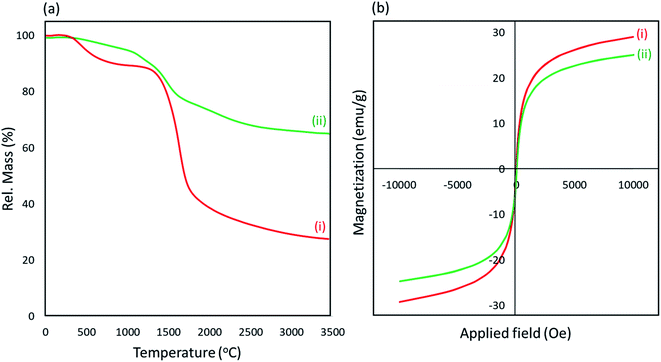 |
| Fig. 4 (a) Thermogravimetric analysis curves and (b) room-temperature M–H curves of the (i) Fe3O4@acacia binary composite and (ii) fabricated Fe3O4@acacia–SO3H nano-powder. | |
2.2.3. XRD study. The X-ray diffraction (XRD) pattern of the prepared Fe3O4@acacia–SO3H nano-powder was also investigated to check the effects of the composited ingredients on the general crystal structure (Fig. 5). With a quick look at the spectrum, the presence of a broad peak starting from 2θ = 20° and continuing to 40° is confirmed. According to the literature, this broad peak is related to the crystal structure of neat acacia gum.34 This result indicates that the acacia polymeric network does not include a well-defined crystal structure in comparison with the inorganic components. Also, there are some other peaks in the XRD spectrum that are relatively sharp and can be considered as indicative signals of the Fe3O4 inorganic crystal structure. Via a comparison with the reference pattern of the Fe3O4 NPs (JCPDS #99-0073), it was revealed that the peaks appearing at 2θ = 30.7, 36.2, 43.4, 57.7, and 63.4° belong to the crystal structure of the composited Fe3O4 NPs. These peaks are also associated with the Miller indices (2 2 0), (3 1 1), (4 0 0), (5 1 1), and (4 4 0), respectively.
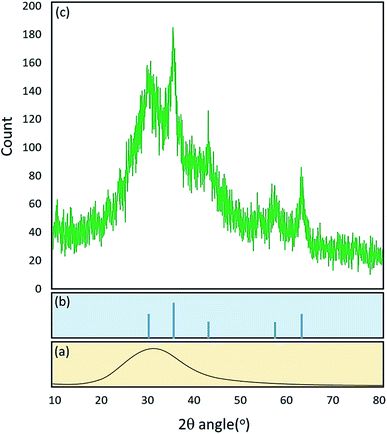 |
| Fig. 5 X-ray diffraction patterns of (a) neat acacia gum, (b) the Fe3O4 NPs, and (c) the fabricated Fe3O4@acacia–SO3H nano-powder. | |
2.2.4. EM study. One of the most preferred methods for investigating the sizes, morphologies, and compositions of microscale and nanoscale materials is electron microscopy (EM). This is because EM gives direct information from the samples without any need for further interpretation or inaccurate estimations. Fig. 6 illustrates the field-emission scanning electron microscopy (FESEM) (images a and b) and transmission electron microscopy (TEM) images (images c and d) of the fabricated Fe3O4@acacia–SO3H nano-powder at different magnifications. As can be observed in all the images, the mean size of the captured Fe3O4 NPs between the acacia textures is around 86 nm. Also, high uniformity in the sizes and shapes of the particles as well as a monotonous distribution onto the acacia gum fibers are nicely illustrated in image (a). Obviously, this good dispersion of the particles provides an extremely active surface area for catalytic applications. The TEM images also clearly disclosed that the spherical-shaped NPs were entrapped in the polymeric matrix. This composition may lead to higher mechanical stability in catalytic systems. This stability will be better highlighted in the recycling process investigation.
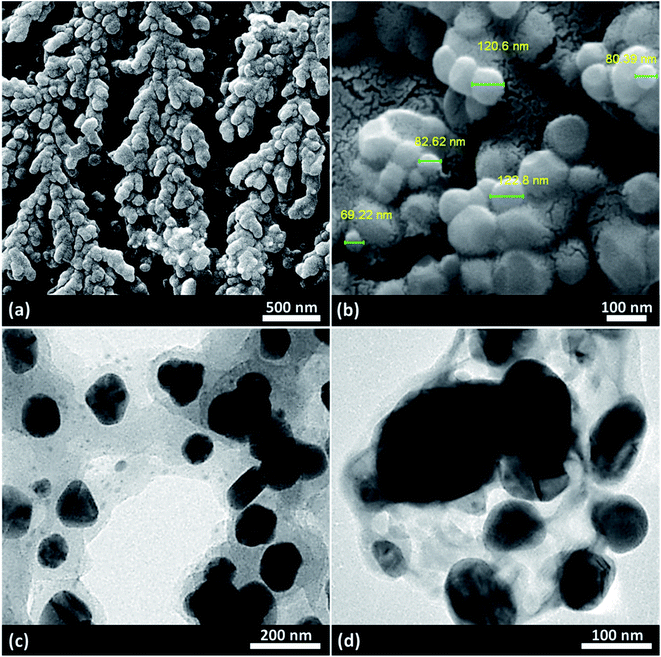 |
| Fig. 6 (a and b) Field-emission scanning electron microscopy and (c and d) transmission electron microscopy images of the fabricated Fe3O4@acacia–SO3H nano-powder. | |
2.3. Catalytic application of the Fe3O4@acacia–SO3H nano-powder in the organic synthesis of 9-phenyl hexahydroacridine pharmaceutical derivatives
As discussed in the Introduction section, the main goal of the design and fabrication of the Fe3O4@acacia–SO3H nano-powder was to provide a suitably active substrate with high heterogeneity to increase the convenience of the organic synthesis of 9-phenyl hexahydroacridine pharmaceutical derivatives. Here, it is clearly demonstrated that high reaction yields were obtained through applying the present catalytic system. Moreover, the reaction time significantly decreased in comparison with the catalyst-free conditions. A brief comparison was made between our novel designed catalytic system and other recently reported systems that highlights the high efficiency of the present nanocomposite in organic catalysis (Table 1). Scheme 1 presents a general view of the targeted organic reaction that was intended to be catalyzed by the Fe3O4@acacia–SO3H nano-powder.
Table 1 Optimization information for the catalyzed synthesis reaction of 9-(4-methoxyphenyl)-3,3,6,6-tetramethyl-3,4,6,7,9,10-hexahydroacridine-1,8(2H,5H)-dionea
Entry |
Cat. system |
Cat. weight (g) |
Medium |
Temp. (°C) |
Time (min) |
Yieldb (%) |
Abbreviations: Cat.: catalyst; Temp.: temperature, DMF: dimethylformamide; DCM: dichloromethane; N.R.: no reaction. The reaction progress was controlled by thin-layer chromatography, and the desired hexahydroacridine product was purified via flash-column chromatography. Isolated yield. Optimum conditions. |
1 |
— |
— |
EtOH |
25 |
110 |
N.R. |
2 |
— |
— |
EtOH |
75 |
110 |
N.R. |
3 |
Fe3O4 NPs |
0.02 |
EtOH |
75 |
110 |
Trace |
4 |
Acacia gum |
0.02 |
EtOH |
75 |
110 |
Trace |
5 |
Fe3O4@acacia–SO3H |
0.01 |
EtOH |
75 |
110 |
88 |
6 |
Fe3O4@acacia–SO3H |
0.02 |
EtOH |
75 |
110 |
94c |
7 |
Fe3O4@acacia–SO3H |
0.02 |
EtOH |
75 |
300 |
94 |
8 |
Fe3O4@acacia–SO3H |
0.03 |
EtOH |
75 |
110 |
94 |
9 |
Fe3O4@acacia–SO3H |
0.03 |
EtOH |
50 |
110 |
91 |
10 |
Fe3O4@acacia–SO3H |
0.02 |
H2O |
80 |
110 |
62 |
11 |
Fe3O4@acacia–SO3H |
0.02 |
DMF |
130 |
110 |
75 |
12 |
Fe3O4@acacia–SO3H |
0.02 |
DCM |
35 |
110 |
79 |
13 |
Fe3O4@acacia–SO3H |
0.02 |
Toluene |
130 |
110 |
76 |
14 |
Fe3O4@acacia–SO3H |
0.02 |
CH3CN |
75 |
110 |
79 |
15 |
Nano-Fe3O4–TiO2–SO3H |
0.01 |
Solvent free |
110 |
55 |
86 (ref. 35) |
16 |
Fe3O4@SiO2–MoO3H |
0.02 |
Solvent free |
90 |
40 |
90 (ref. 36) |
17 |
Cell-Pr-NHSO3H |
0.05 |
Ethanol |
Reflux |
48 |
88 (ref. 37) |
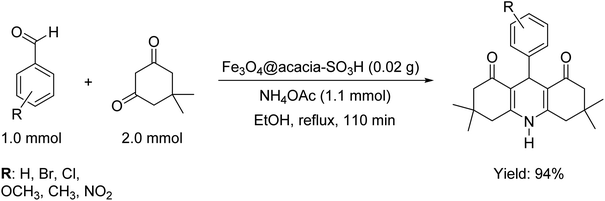 |
| Scheme 1 General schematic of the organic synthesis reaction of the 9-phenyl hexahydroacridine derivatives catalyzed by the Fe3O4@acacia–SO3H nanocatalyst. | |
2.3.1. Optimization. Concisely, various conditions, including catalyst-free reactions, reactions catalyzed by the neat Fe3O4 NPs and acacia gum powder individually, and catalytic systems with different amounts of Fe3O4@acacia–SO3H, various reaction media and different reaction times were precisely monitored in the synthesis reaction of 9-(4-methoxyphenyl)-3,3,6,6-tetramethyl-3,4,6,7,9,10-hexahydroacridine-1,8(2H,5H)-dione, which was considered as a model reaction. Table 1 briefly reports the obtained results from each case and also shows that 94% yield was obtained through using 0.02 g of Fe3O4@acacia–SO3H nanocomposite under reflux conditions.
2.3.3. Suggested mechanism of the catalytic activity of the Fe3O4@acacia–SO3H nano-powder. The Fe3O4@acacia–SO3H nano-powder is an acidic catalytic system in which the catalysis proceeds via H-bonding interactions with the involved ingredients in the synthesis reactions. As a plausible mechanism, Fe3O4@acacia–SO3H starts with activation of the aldehyde component in the first stage. Dimedone enters the cycle by performing a nucleophilic attack on the activated aldehyde (stage 2). Next, a π-conjugated system is formed during a dehydration process (stage 3). Afterward, in stage four, another dimedone performs a nucleophilic attack on the structure of the conjugated compound; then, NH4 enters the cycle and forms the structure of the target 9-phenyl hexahydroacridine. Scheme 2 schematically presents the explained catalytic cycle.46–51
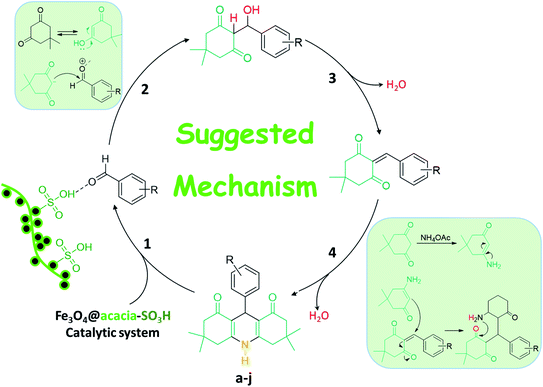 |
| Scheme 2 Plausible mechanism of the catalytic activity of the fabricated Fe3O4@acacia–SO3H nanocatalyst in the synthesis reactions of 9-phenyl hexahydroacridine derivatives. | |
2.3.4. Recyclability of the Fe3O4@acacia–SO3H catalytic system. The stability of the fabricated catalytic system was precisely investigated by successive running of the catalytic process in the synthesis reaction of product i. As can be seen in Fig. 7(a), acceptable reaction yields were obtained in a total of ten runs of the reaction. After recycling and reusing the nanoparticles ten times, FT-IR and EDX spectra of the recovered nanocomposite were prepared and investigated. From these analyses, it was clearly revealed that no significant changes occurred in the structure of the Fe3O4@acacia–SO3H catalytic system. As can be observed in Fig. 7(b and c), all of the distinct indicative peaks appeared in both spectra. Moreover, inductively coupled plasma (ICP) analysis was performed to investigate the metal leaching from the system. After completion of the catalytic process (after run 1), the particles were separated and the supernatant was filtered and analyzed. Briefly, it was observed that only 0.15 mg of the iron element leached from 0.05 g of the catalytic system.
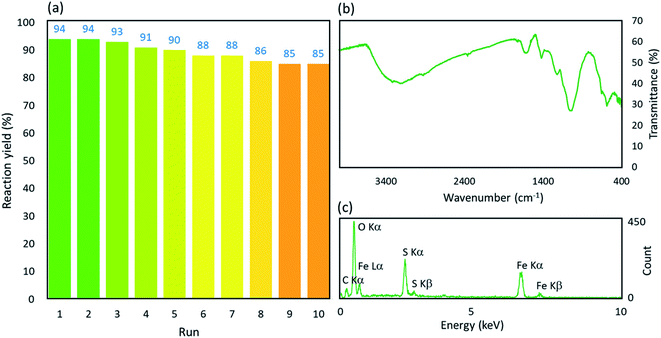 |
| Fig. 7 (a) Recycling diagram, (b) Fourier-transform infrared spectrum, and (c) energy-dispersive X-ray spectrum of the recovered Fe3O4@acacia–SO3H catalytic system. | |
3. Experimental
3.1. Materials and equipment
All commercially available chemicals, solvents, reagents and were purchased from Sigma-Aldrich and Merck Company. All the applied materials and equipment are summarized in Table S1.†
3.2. Practical methods
3.2.1. Preparation of Fe3O4@acacia binary composite. In a round bottom flask (50 mL), FeCl2·4H2O and FeCl3·6H2O salts (1.0 mmol and 2.0 mmol, respectively) were dissolved in deionized water (10 mL) via vigorous stirring at room temperature. Then, acacia gum powder (0.6 g) was added in several portions and also dissolved. In the next stage, the reaction mixture was gradually heated to around 80 °C under a neutral atmosphere of N2. Then, ammonia solution (13 mL) was added dropwise until the pH value reached ∼12. The dark mixture was then stirred under the same conditions for an additional 1 h. Finally, the magnetic particles were collected via holding an external magnet at the bottom of the flask after cooling to room temperature. The particles were washed with ethanol and water several times and dried in an oven at 60 °C.
3.2.2. Preparation of Fe3O4@acacia–SO3H nano-powder. In a round bottom flask (50 mL), the particles of Fe3O4@acacia (0.6 g) were dispersed in chloroform (10 mL), and the temperature was reduced by an ice bath. In a separate flask, chlorosulfonic acid (99%) (2.0 mL) was mixed with chloroform (2.0 mL), and the resulting solution was added dropwise to the main reaction flask with stirring. After completion of the addition, the ice bath was removed, and vigorous stirring was continued for an additional 2 h at room temperature. Ultimately, the product was magnetically separated, washed, and dried as described above.
3.2.3. General procedure for the catalyzed synthesis of 9-phenyl hexahydroacridine pharmaceutical derivatives. In a round bottom flask (25 mL), aldehyde (1.0 mmol), dimedone (2.0 mmol), ammonium acetate salt (1.1 mmol), and Fe3O4@acacia–SO3H nano-powder (0.02 g) were mixed in ethanol (2.0 mL), and the mixture was refluxed. After the appropriate time had passed (110 min), the particles of the catalytic system were magnetically removed and the desired product was purified via flash-column chromatography. The original 1H and 13C-NMR spectra of the selected products are shown in Fig. S1–S20 in the ESI† section.
3.2.4. Recycling of the catalyst. After completion of the first round, the Fe3O4@acacia–SO3H particles were magnetically separated and the rest were separated via decanting. Then, the particles were washed well with deionized water and ethanol (20 mL) four successive times. Afterward, the particles were died in a vacuum oven for 24 h. To reuse the particles, redispersion was initially performed by an ultrasound cleaner bath (50 kHz, 200 W L−1); then, the reactants were added to the flask.
3.3. Spectral data for selected products
3,3,6,6-Tetramethyl-9-phenyl-3,4,6,7,9,10-hexahydroacridine-1,8(2H,5H)-dione (product a): M. P (°C): 279–281. 1H NMR (300 MHz, DMSO), δ (ppm): 9.30 (s, 1H), 7.00–7.13 (m, 5H), 4.60 (s, 1H), 2.40–2.48 (m, 2H), 2.27 (d, 2H), 2.12 (d, 2H), 2.00 (d, 2H), 0.98 (s, 6H), 0.83 (s, 6H). 13C NMR (75 MHz, DMSO), δ (ppm): 194.3, 149.3, 147.1, 127.6, 127.5, 125.4, 111.4, 50.2, 32.9, 32.2, 29.1, 26.4.
9-(2-Chlorophenyl)-3,3,6,6-tetramethyl-3,4,6,7,9,10-hexahydroacridine-1,8(2H,5H)-dione (product b): M. P (°C): 264–266. 1H NMR (300 MHz, DMSO), δ (ppm): 9.59 (s, 1H), 7.25–7.27 (d, 1H), 7.10–7.20 (m, 1H), 7.04–7.08 (m, 1H), 6.99–7.01 (m, 1H), 5.05 (s, 1H), 2.71–2.10 (m, 8H), 0.98 (s, 6H), 0.92 (s, 6H). 13C NMR (75 MHz, DMSO), δ (ppm): 196.4, 152.4, 142.6, 132.0, 129.7, 128.2, 115.2, 50.6, 40.8, 32.2, 31.4, 29.3, 27.2.
9-(4-Chlorophenyl)-3,3,6,6-tetramethyl-3,4,6,7,9,10-hexahydroacridine-1,8(2H,5H)-dione (product c): M. P (°C): 290–292. 1H NMR (300 MHz, DMSO), δ (ppm): 9.92 (s, 1H), 7.27–7.30 (d, 2H), 7.18–7.19 (d, 2H), 4.50 (s, 1H), 2.50–2.59 (dd, 4H), 2.25–2.28 (d, 2H), 2.07–2.10 (d, 2H), 1.04 (s, 6H), 0.90 (s, 6H). 13C NMR (75 MHz, DMSO), δ (ppm): 196.1, 149.3, 147.1, 131.7, 129.6, 129.3, 128.2, 113.1, 50.2, 33.6, 32.8, 29.1, 27.3.
9-(2,4-Dichlorophenyl)-3,3,6,6-tetramethyl-3,4,6,7,9,10-hexahydroacridine-1,8(2H,5H)-dione (product d): M. P (°C): 318–320. 1H NMR (300 MHz, DMSO), δ (ppm): 9.95 (s, 1H), 7.34 (s, 1H), 7.28–7.33 (d, 1H), 7.19–7.22 (d, 1H), 5.58 (s, 1H), 2.38–2.43 (m, 2H), 2.34 (d, 2H), 2.32 (d, 2H), 2.31 (d, 2H), 1.14 (s, 6H), 1.07 (s, 6H). 13C NMR (75 MHz, DMSO), δ (ppm): 189.8, 135.3, 134.1, 130.0, 129.5, 126.7, 115.4, 47.0, 46.9, 46.3, 31.7, 31.2, 28.9, 27.8.
9-(3-Methylphenyl)-3,3,6,6-tetramethyl-3,4,6,7,9,10-hexahydroacridine-1,8(2H,5H)-dione (product e): M. P (°C): 211–213. 1H NMR (300 MHz, DMSO), δ (ppm): 8.07 (s, 1H), 7.26 (s, 1H), 7.09–7.11 (d, 1H), 7.03–7.08 (m, 1H), 6.86–6.88 (d, 1H), 5.05 (s, 1H), 2.32–2.40 (m, 2H), 2.26 (d, 2H), 2.22 (d, 2H), 2.16 (d, 2H), 1.05 (s, 6H), 0.95 (s, 6H). 13C NMR (75 MHz, DMSO), δ (ppm): 196.1, 149.5, 146.7, 137.3, 129.1, 128.0, 126.9, 125.2, 113.3, 51.1, 40.8, 33.6, 32.7, 29.8, 27.2, 21.8.
9-(3-Methoxyphenyl)-3,3,6,6-tetramethyl-3,4,6,7,9,10-hexahydroacridine-1,8(2H,5H)-dione (product f): M. P (°C): 301–303. 1H NMR (300 MHz, DMSO), δ (ppm): 8.94 (s, 1H), 7.25 (s, 1H), 7.22 (d, 1H), 7.01 (m, 1H), 6.98 (d, 1H), 5.07 (s, 1H), 3.91 (s, 3H), 2.22–2.27 (m, 2H), 2.17 (d, 2H), 2.11 (d, 2H), 2.04 (d, 2H), 1.12 (s, 6H), 0.95 (s, 6H). 13C NMR (75 MHz, DMSO), δ (ppm): 196.1, 158.72, 149.9, 143.8, 134.9, 128.5, 127.7, 125.2, 112.8, 50.8, 40.2, 33.0, 32.3, 29.4, 27.9, 20.9.
9-(4-Nitrophenyl)-3,3,6,6-tetramethyl-3,4,6,7,9,10-hexahydroacridine-1,8(2H,5H)-dione (product g): M. P (°C): 286–288. 1H NMR (300 MHz, DMSO), δ (ppm): 9.90 (s, 1H), 7.19–7.21 (d, 2H), 7.12–7.14 (d, 2H), 4.76 (s, 1H), 2.42–2.49 (d, 4H), 2.14–2.32 (d, 2H), 1.95–1.98 (d, 2H), 0.99 (s, 6H), 0.84 (s, 6H). 13C NMR (75 MHz, DMSO), δ (ppm): 194.4, 149.5, 146.1, 129.9, 129.5, 127.5, 115.5, 111.1, 50.1, 40.0, 32.6, 32.2, 29.0, 26.4.
3,3,6,6-Tetramethyl-9-(3-nitrophenyl)-3,4,6,7,9,10-hexahydroacridine-1,8(2H,5H)-dione (product h): M. P (°C): 281–283. 1H NMR (300 MHz, DMSO), δ (ppm): 9.31 (s, 1H), 8.00–8.01 (d, 2H), 7.65–7.66 (d, 1H), 7.54–7.57 (t, 1H), 4.65 (s, 1H), 2.50–2.59 (dd, 4H), 2.27–2.30 (d, 2H), 2.08–2.12 (d, 2H), 1.04 (s, 6H), 0.91 (s, 6H). 13C NMR (75 MHz, DMSO), δ (ppm): 196.0, 149.4, 149.0, 131.1, 129.7, 122.3, 112.9, 51.0, 40.9, 33.9, 32.8, 29.7, 27.3, 21.1.
9-(4-Methoxyphenyl)-3,3,6,6-tetramethyl-3,4,6,7,9,10-hexahydroacridine-1,8(2H,5H)-dione (product i): M. P (°C): 288–290. 1H NMR (300 MHz, DMSO), δ (ppm): 9.20 (s, 1H), 7.05–7.07 (t, 2H), 6.76–6.80 (t, 2H), 4.46 (s, 1H), 3.70 (s, 3H), 2.56 (d, 2H), 2.49–2.51 (m, 2H), 2.27 (d, 2H), 2.09 (d, 2H), 1.03 (s, 6H), 0.91 (s, 6H). 13C NMR (75 MHz, DMSO), δ (ppm): 196.2, 157.5, 149.6, 139.2, 128.8, 125.2, 113.0, 50.8, 40.36, 32.6, 32.4, 29.5, 26.9.
9-(4-Bromophenyl)-3,3,6,6-tetramethyl-3,4,6,7,9,10-hexahydroacridine-1,8(2H,5H)-dione (product j): M. P (°C): 321–324. 1H NMR (300 MHz, DMSO), δ (ppm): 9.33 (s, 1H), 7.23–7.26 (dd, 2H), 6.87–6.92 (m, 2H), 4.73 (s, 1H), 2.26–2.46 (m, 4H), 2.19–2.22 (m, 2H), 2.15 (m, 4H), 1.10 (s, 6H), 0.99 (s, 6H). 13C NMR (75 MHz, DMSO), δ (ppm): 194.4, 149.6, 149.3, 147.1, 127.9, 127.8, 126.0, 111.9, 50.7, 32.2, 31.2, 29.2, 27.3.
4. Conclusions
In this work, we designed and fabricated a novel catalytic system with high heterogeneity and magnetic features to facilitate the MCR synthetic reactions of 9-phenyl hexahydroacridine pharmaceutical derivatives. A combination of acacia gum (gum arabic) with iron oxide magnetic particles on the nanoscale was used as a magnetized natural matrix. From the physicochemical aspect, through effective H-binding interactions, the organic and inorganic ingredients were firmly fixed and combined well with each other. EM imaging approaches indeed disclosed the composition of the composite. Then, the prepared Fe3O4@acacia binary composite was equipped with sulfone groups, which are considered to be the main active catalytic sites. Afterward, the high catalytic performance of the formed cluster-shaped composite was investigated in the organic synthesis reactions of 9-phenyl hexahydroacridine derivatives. The mechanical and thermal stability of the fabricated Fe3O4@acacia–SO3H nano-powder was also studied, and this substantial stability was highlighted in the recycling process. Overall, herein, we have made an effort to comprehensively study the structural features of the Fe3O4@acacia–SO3H nano-powder and demonstrate the catalytic performance of this product. Due to the high convenience of the synthesis process and the low prices of the used raw materials, this product is recommended for industrial applications.
Conflicts of interest
The authors declare no conflict of interest.
Acknowledgements
The authors gratefully acknowledge the partial support from the Research Council of the Iran University of Science and Technology (IUST). Furthermore, AES is grateful for the National Research grants from MINECO “Juan de la Cierva” [FJCI-2018-037717].
References
- A. Gulati, J. Malik, Mandeep and R. Kakkar, Peanut shell biotemplate to fabricate porous magnetic Co3O4 coral reef and its catalytic properties for p-nitrophenol reduction and oxidative dye degradation, Colloids Surf., A, 2020, 604, 125328 CAS.
- R. Taheri-Ledari, J. Rahimi and M. Ali, Mater. Res. Express, 2020, 7, 015067 CrossRef CAS.
- W. Zhang, R. Taheri-Ledari, Z. Hajizadeh, E. Zolfaghari, M. R. Ahghari, A. Maleki, M. R. Hamblin and Y. Tian, Enhanced activity of vancomycin by encapsulation in hybrid magnetic nanoparticles conjugated to a cell-penetrating peptide, Nanoscale, 2020, 12, 3855–3870 RSC.
- N. Kang, D. Xu, Y. Han, X. Lv, Z. Chen, T. Zhou, L. Ren and X. Zhou, Magnetic targeting core/shell Fe3O4/Au nanoparticles for magnetic resonance/photoacoustic dual-modal imaging, Mater. Sci. Eng., C, 2019, 98, 545–549 CrossRef CAS.
- R. T. Ledari, W. Zhang, M. Radmanesh, S. S. Mirmohammadi, A. Maleki, N. Cathcart and V. Kitaev, Small, 2020, 2002733 CrossRef.
- Z. Hajizadeh, K. Valadi, R. Taheri-Ledari and A. Maleki, Convenient Cr(VI) Removal from Aqueous Samples: Executed by a Promising Clay-Based Catalytic System, Magnetized by Fe3O4 Nanoparticles and Functionalized with Humic Acid, ChemistrySelect, 2020, 5, 2441–2448 CrossRef CAS.
- A. Maleki, F. Hassanzadeh-Afruzi, Z. Varzi and M. S. Esmaeili, Magnetic dextrin nanobiomaterial: an organic–inorganic hybrid catalyst for the synthesis of biologically active polyhydroquinoline derivatives by asymmetric Hantzsch reaction, Mater. Sci. Eng., C, 2020, 109, 110502 CrossRef CAS.
- A. Maleki, R. Taheri-Ledari and M. Soroushnejad, Surface functionalization of magnetic nanoparticles via palladium-catalyzed Diels-Alder approach, ChemistrySelect, 2018, 3, 13057–13062 CrossRef CAS.
- A. E. Shalan, M. Rasly and M. M. Rashad, Organic acid precursor synthesis and environmental photocatalysis applications of mesoporous anatase TiO2 doped with different transition metal ions, J. Mater. Sci.: Mater. Electron., 2014, 25(7), 3141–3146 CrossRef CAS.
- S. Parvaz, R. Taheri-Ledari, M. S. Esmaeili, M. Rabbani and A. Maleki, A brief survey on the advanced brain drug administration by nanoscale carriers: with a particular focus on AChE reactivators, Life Sci., 2020, 240, 117099 CrossRef CAS.
- A. Maleki, R. Taheri-Ledari, J. Rahimi, M. Soroushnejad and Z. Hajizadeh, Facile peptide bond formation: effective interplay between isothiazolone rings and silanol groups at silver/iron oxide nanocomposite surfaces, ACS Omega, 2019, 4, 10629–10639 CrossRef CAS.
- A. Maleki, R. Taheri-Ledari, R. Ghalavand and R. Firouzi-Haji, Palladium-decorated o-phenylenediamine-functionalized Fe3O4/SiO2 magnetic nanoparticles: a promising solid-state catalytic system used for Suzuki–Miyaura coupling reactions, J. Phys. Chem. Solids, 2020, 136, 109200 CrossRef CAS.
- R. Taheri-Ledari, A. Maleki, E. Zolfaghari, M. Radmanesh, H. Rabbani, A. Salimi and R. Fazel, High-performance sono/nano-catalytic system: Fe3O4@Pd/CaCO3-DTT core/shell nanostructures, a suitable alternative for traditional reducing agents for antibodies, Ultrason. Sonochem., 2020, 61, 104824 CrossRef CAS.
- R. Taheri-Ledari, J. Rahimi and A. Maleki, Synergistic catalytic effect between ultrasound waves and pyrimidine-2,4-diamine-functionalized magnetic nanoparticles: applied for synthesis of 1,4-dihydropyridine pharmaceutical derivatives, Ultrason. Sonochem., 2019, 59, 104737 CrossRef.
- K. Valadi, S. Gharibi, R. Taheri-Ledari and A. Maleki, Ultrasound-assisted synthesis of 1,4-dihydropyridine derivatives by an efficient volcanic-based hybrid nanocomposite, Solid State Sci., 2020, 101, 106141 CrossRef CAS.
- J. Rahimi, R. Taheri-Ledari, M. Niksefat and A. Maleki, Enhanced reduction of nitrobenzene derivatives: effective strategy executed by Fe3O4/PVA-10% Ag as a versatile hybrid nanocatalyst, Catal. Commun., 2020, 134, 105850 CrossRef CAS.
- A. Maleki, M. Niksefat, J. Rahimi and R. Taheri-Ledari, Multicomponent synthesis of pyrano[2,3-d]pyrimidine derivatives via a direct one-pot strategy executed by novel designed cooperated Fe3O4@polyvinyl alcohol magnetic nanoparticles, Mater. Today Chem., 2019, 13, 110–120 CrossRef CAS.
- R. Taheri-Ledari, S. M. Hashemi and A. Maleki, High-performance sono/nano-catalytic system: CTSN/Fe3O4–Cu nanocomposite, a promising heterogeneous catalyst for the synthesis of N-arylimidazoles, RSC Adv., 2019, 9, 40348–40356 RSC.
- S. S. Soltani, R. Taheri-Ledari, S. M. F. Farnia, A. Maleki and A. Foroumadi, Synthesis and characterization of a supported Pd complex on volcanic pumice laminates textured by cellulose for facilitating Suzuki–Miyaura cross-coupling reactions, RSC Adv., 2020, 10, 23359–23371 RSC.
- R. Taheri-Ledari and A. Maleki, Antimicrobial therapeutic enhancement of levofloxacin via conjugation to a cell-penetrating peptide: an efficient sonochemical catalytic process, J. Pept. Sci., 2020, 26, e3277 CrossRef CAS.
- G. U. Rehman, M. Tahir, P. S. Goh, A. F. Ismail and I. U. Khan, Controlled synthesis of reduced graphene oxide supported magnetically separable Fe3O4@rGO@AgI ternary nanocomposite for enhanced photocatalytic degradation of phenol, Powder Technol., 2019, 356, 547–558 CrossRef CAS.
- V. Javanbakht, S. M. Ghoreishi, N. Habibi and M. Javanbakht, A novel magnetic chitosan/clinoptilolite/magnetite nanocomposite for highly efficient removal of Pb(II) ions from aqueous solution, Powder Technol., 2016, 302, 372–383 CrossRef CAS.
- A. M. lslam, G. O. Phillipsl, A. Sljivo, M. J. Snowden and P. A. Williams, A review of recent developments on the regulatory, structural and functional aspects of gum Arabic, Food Hydrocolloids, 1997, 11, 493–505 CrossRef.
- S. S. Banerjee and D. H. Chen, Fast removal of copper ions by gum Arabic modified magnetic nano-adsorbent, J. Hazard. Mater., 2007, 147, 792–799 CrossRef CAS.
- Á. Magyar and Z. Hell, Molecular Sieve Supported Lanthanum Catalyst for the Efficient Synthesis of Polyhydroquinolines via Hantzsch Synthesis, Period. Polytech., Chem. Eng., 2017, 61, 278–282 CrossRef.
- K. T. Naveen, K. B. Sai and K. Chandana, Synthesis, Characterization and screening of novel 5,6-dihydroacridine derivatives as potent antidiabetic and antioxidant agent, J. Basic Appl. Res. Int., 2016, 2, 176–184 Search PubMed.
- A. Maleki, Z. Varzi and F. Hassanzadeh-Afruzi, Preparation and characterization of an eco-friendly ZnFe2O4@alginic acid nanocomposite catalyst and its application in the synthesis of 2-amino-3-cyano-4H-pyran derivatives, Polyhedron, 2019, 171, 193–202 CrossRef CAS.
- Z. Varzi and A. Maleki, Design and preparation of ZnS-ZnFe2O4: a green and efficient hybrid nanocatalyst for the multicomponent synthesis of 2,4,5-triaryl-1H-imidazoles, Appl. Organomet. Chem., 2019, 33, e5008 CrossRef.
- H. Veisi, N. Dadres, P. Mohammadi and S. Hemmati, Green synthesis of silver nanoparticles based on oil-water interface method with essential oil of orange peel and its application as nanocatalyst for A3 coupling, Mater. Sci. Eng., C, 2019, 105, 110031 CrossRef CAS.
- P. K. Khatri, M. Manchanda, I. K. Ghosh and S. L. Jain, Polymer impregnated sulfonated carboncomposite solid acid catalyst for alkylation of phenol with methyl-tert-butyl ether, RSC Adv., 2015, 5, 3286–3290 RSC.
- M. S. Esmaeili, Z. Varzi, R. Eivazzadeh-Keihan, A. Maleki and H. Ghafuri, Design and development of natural and biocompatible raffinose-Cu2O magnetic nanoparticles as a heterogeneous nanocatalyst for the selective oxidation of alcohols, Mol. Catal., 2020, 492, 111037 CrossRef CAS.
- A. Bagheri, P. Salarizadeh, M. S. Asre Hazer, P. Hosseinabadi, S. Kashefi and H. Beydaghi, The effect of adding sulfonated SiO2 nanoparticles and polymer blending on properties and performance of sulfonated poly ether sulfone membrane: fabrication and optimization, Electrochim. Acta, 2019, 295, 875–890 CrossRef CAS.
- C. Işik, G. Arabaci, Y. I. Doğaç, İ. Deveci and M. Teke, Synthesis and characterization of electrospun PVA/Zn2+ metal composite nanofibers for lipase immobilization with effective thermal, pH stabilities and reusability, Mater. Sci. Eng., C, 2019, 99, 1226–1235 CrossRef.
- T. Şişmanoğlu, S. Karakuş, Ö. Birer, G. S. P. Soylu, A. Kolan, E. Tan, Ö. Ürk, G. Akdut and A. Kilislioglu, Preparation and characterization of antibacterial Senegalia (Acacia) senegal/iron–silica bio-nanocomposites, Appl. Surf. Sci., 2015, 354, 250–255 CrossRef.
- A. Amoozadeh, S. Golian and S. Rahmani, TiO2-coated magnetite nanoparticle-supported sulfonic acid as a new, efficient, magnetically separable and reusable heterogeneous solid acid catalyst for multicomponent reactions, RSC Adv., 2015, 5, 45974–45982 RSC.
- M. Kiani and M. Mohammadipour, Fe3O4@SiO2–MoO3H nanoparticles: a magnetically recyclable nanocatalyst system for the synthesis of 1,8-dioxo-decahydroacridine derivatives, RSC Adv., 2017, 7, 997–1007 RSC.
- S. Karhale, C. Bhenki, G. Rashinkar and V. Helavi, Covalently anchored sulfamic acid on cellulose as heterogeneous solid acid catalyst for the synthesis of structurally symmetrical and unsymmetrical 1,4-dihydropyridine derivatives, New J. Chem., 2017, 41, 5133–5141 RSC.
- G. M. Ziarani, A. Badiei, M. Hassanzadeh and S. Mousavi, Synthesis of 1,8-dioxo-decahydroacridine derivatives using sulfonic acid functionalized silica (SiO2–Pr–SO3H) under solvent free conditions, Arabian J. Chem., 2014, 7, 335–339 CrossRef CAS.
- D. Patil, D. Chandam, A. Mulik, P. Patil, S. Jagadale, R. Kant, V. Gupta and M. Deshmukh, Novel Brønsted Acidic Ionic Liquid ([CMIM][CF3COO]) Prompted Multicomponent Hantzsch Reaction for the Eco-Friendly Synthesis of Acridinediones: An Efficient and Recyclable Catalyst, Catal. Lett., 2014, 144, 949–958 CrossRef CAS.
- A. Magyar and Z. Hell, An Efficient One-Pot Four-Component Synthesis of 9-Aryl-Hexahydroacridine-1,8-Dione Derivatives in the Presence of a Molecular Sieves Supported Iron Catalyst, Catal. Lett., 2019, 149, 2528–2534 CrossRef CAS.
- S. Asgharnasl, R. Eivazzadeh-Keihan, F. Radinekiyan and A. Maleki, Preparation of a novel magnetic bionanocomposite based on factionalized chitosan by creatine and its application in the synthesis of polyhydroquinoline, 1,4-dyhdropyridine and 1,8-dioxo-decahydroacridine derivatives, Int. J. Biol. Macromol., 2020, 144, 29–46 CrossRef CAS.
- E. Eidi, M. Z. Kassaee and Z. Nasresfahani, Nanocrystalline TiO2, via green combustion synthesis, as an efficient and reusable catalyst for the preparation of 1,8-dioxooctahydroxanthenes and 1,8-dioxodecahydroacridines, Appl. Organomet. Chem., 2015, 29, 793–797 CrossRef CAS.
- M. S. Mirhosseyni, F. Nemati and A. Elhampour, Hollow Fe3O4@DA–SO3H: an efficient and reusable heterogeneous nano-magnetic acid catalyst for synthesis of dihydropyridine and dioxodecahydroacridine derivatives, J. Iran. Chem. Soc., 2017, 14, 791–801 CrossRef CAS.
- A. Amoozadeh, S. Rahmani, M. Bitaraf, F. B. Abadi and E. Tabrizian, Nano-zirconia as an excellent nano support for immobilization of sulfonic acid: a new, efficient and highly recyclable heterogeneous solid acid nanocatalyst for multicomponent reactions, New J. Chem., 2016, 40, 770–780 RSC.
- M. A. Zolfigol, F. Karimi, M. Yarie and M. Torabi, Catalytic application of sulfonic acid-functionalized titana-coated magnetic nanoparticles for the preparation of 1,8-dioxodecahydroacridines and 2,4,6-triarylpyridines via anomeric-based oxidation, Appl. Organomet. Chem., 2018, 32, e4063 CrossRef.
- S. Karhale, C. Bhenki, G. Rashinkar and V. Helavi, Covalently anchored sulfamic acid on cellulose as heterogeneous solid acid catalyst for the synthesis of structurally symmetrical and unsymmetrical 1,4-dihydropyridine derivatives, New J. Chem., 2017, 41, 5133–5141 RSC.
- A. Bamoniri and S. Fouladgar, SnCl4-functionalized nano-Fe3O4 encapsulated-silica particles as a novel heterogeneous solid acid for the synthesis of 1,4-dihydropyridine derivatives, RSC Adv., 2015, 5, 78483–78490 RSC.
- A. Bamoniri, B. B. F. Mirjalili and S. Fouladgar, Sonochemically synthesis of 1,4-dihydropyridine derivatives using nano-silica supported tin tetrachloride as a reusable solid acid catalyst, J. Taiwan Inst. Chem. Eng., 2016, 63, 396–403 CrossRef CAS.
- Z. Zarei and B. Akhlaghinia, ZnII doped and immobilized on functionalized magnetic hydrotalcite (Fe3O4/HT-SMTU-ZnII): a novel, green and magnetically recyclable bifunctional
nanocatalyst for the one-pot multi-component synthesis of acridinediones under solvent-free conditions, New J. Chem., 2017, 41, 15485–15500 RSC.
- A. Zhu, R. Liu, C. Du and L. Li, Betainium-based ionic liquids catalysed multicomponent Hantzsch reactions for the efficient synthesis of acridinediones, RSC Adv., 2017, 7, 6679–6684 RSC.
- L. M. Ramos, M. O. Rodrigues and B. A. D. Neto, Org. Biomol. Chem., 2019, 17, 7260–7269 RSC.
Footnote |
† Electronic supplementary information (ESI) available: The table of the materials and equipment applied in this project and the original spectra of the selected synthesized 9-phenyl hexahydroacridine compounds. This section can be found in the online version. See DOI: 10.1039/d0ra07986c |
|
This journal is © The Royal Society of Chemistry 2020 |
Click here to see how this site uses Cookies. View our privacy policy here.