DOI:
10.1039/D0RA07701A
(Paper)
RSC Adv., 2020,
10, 39708-39711
Rhodium(III)-catalyzed C–H annulation of 2-acetyl-1-arylhydrazines with sulfoxonium ylides: synthesis of 2-arylindoles†
Received
8th September 2020
, Accepted 23rd October 2020
First published on 30th October 2020
Abstract
An efficient Rh(III)-catalyzed synthesis of 2-arylindole derivatives via intermolecular C–H annulation of arylhydrazines with sulfoxonium ylides was accomplished. A variety of 2-acetyl-1-arylhydrazines with sulfoxonium ylides were converted into 2-arylindoles in satisfactory yields. Excellent selectivity and good functional group tolerance of this transformation were also observed.
Introduction
Indoles represent one of the most abundant heterocycles in natural products, biologically active molecules, pharmacological compounds, and materials.1 Particularly, 2-arylindole and its derivatives are core structural frameworks in numerous drug molecules (Scheme 1).2 Traditional strategies to access 2-arylindoles include Fisher,3 Larock,4 Buchwald,5 and Hegadus indole synthesis.6 However, the above methods usually suffer from harsh reaction conditions, multistep synthesis, limited substrate scope, and undesirable toxic waste was inevitable in some transformations. Therefore, developing more convenient, efficient and sustainable methods to access 2-arylindole derivatives is highly desirable.
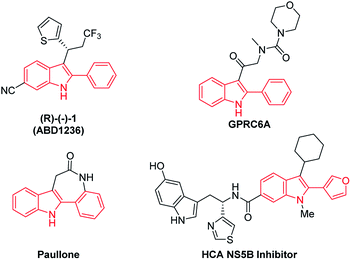 |
| Scheme 1 Important representative 2-arylindole derivatives. | |
Over the past decades, transition-metal-catalyzed directed C–H activation has been developed as a powerful and straightforward synthetic approach to heterocycles.7 Moreover, efficient synthesis of indole derivatives using this strategy has also been greatly employed.8 In recent years, sulfoxonium ylides, featuring operational safety and synthetic convenience as popular carbene surrogates,9 were used as important building blocks in transition-metal-catalyzed C–H annulation reactions with nucleophilic directing groups for synthesis of indole derivatives. In 2019, Huang and co-workers realized efficient synthesis of 2-arylindole via Ru(II)-catalyzed tandem annulation of N-aryl-2-aminopyridines and sulfoxonium ylides (Scheme 2a).10 In the same year, Liu's group reported [Ru(p-cymene)Cl2]2 catalyzed imidamides C–H activation and coupling with sulfoxonium ylides to give 2-arylindole (Scheme 2b).11 In 2020, Wu's and Cui's group developed a novel synthesis of 2-arylindole from N-nitrosoanilines and sulfoxonium ylides via Rh(III)-catalyzed acylmethylation (Scheme 2c).12 Encouraged by these eminent studies, herein a simple Rh(III)-catalyzed synthesis of 2-arylindole was disclosed by easily available arylhydrazines with sulfoxonium ylides as substrates (Scheme 2d).
 |
| Scheme 2 Ru/Rh-catalyzed C–H annulation of sulfur ylides to 2-arylindole. | |
Results and discussion
The C–H annulation reaction of 2-acetyl-1-phenylhydrazine (1a) with α-benzoyl sulfoxonium ylide (2a) was used as a model to optimize the reaction conditions (Table 1). Catalyst systems were first screened in the presence of NaOAc, which was used as the additive, in 1,2-dichloroethane (DCE) at 100 °C under a nitrogen atmosphere. The desired product 3aa was obtained with 35% yield by using [Cp*RhCl2]2/AgSbF6 as the catalyst system (entry 1). The transformation does not occur in the presence of other catalysts such as [Cp*Co(CO)I2]2 and [RuCl2(p-cymene)]2 (entry 2 and 3). The effect of silver salts was investigated (entry 1 vs. entry 4). AgNTf2 gave the desired product 3aa in 62% yield. The solvents were subsequently screened using [Cp*RhCl2]2/AgNTf2 as the catalyst system. Among the solvents examined [1,2-dichloroethane (DCE), 1,4-dioxane (dioxane), toluene, and methanol (MeOH)], DCE was the best solvent (entry 4 vs. entries 5–7). Among the various additives tested, NaOAc/HOAc showed the highest efficiency for the reaction (entries 8–11). Further enhancement of the yield (93%) of 3aa was achieved by increasing the loading of HOAc (entry 12). No reaction was observed when the model reaction was conducted in the absence of AgNTf2 (entry 13). Therefore, the optimal reaction conditions were identified as follows: 2.5 mol% of [Cp*RhCl2]2 with AgNTf2 (10 mol%) as the catalyst system, NaOAc (50 mol%) with HOAc (1.0 equiv.) as the additives in DCE at 100 °C under a nitrogen atmosphere for 12 h.
Table 1 Screening of the reaction conditionsa

|
Entry |
Catalyst system |
Additive |
Solvent |
Yieldb (%) |
Reaction conditions: 2-acetyl-1-phenylhydrazine (1a, 0.2 mmol, 30.0 mg), α-benzoyl sulfoxonium ylide (2a, 0.24 mmol, 47.1 mg), catalyst (2.5 mol%), AgSbF6 or AgNTf2 (10 mol%), and additive (50 mol%) in solvent (1.0 mL) at 100 °C under a nitrogen atmosphere for 12 h. Isolated yield. 1.0 equiv. of PivOH was used. 1.0 equiv. of HOAc was used. |
1 |
[Cp*RhCl2]2/AgSbF6 |
NaOAc |
DCE |
35 |
2 |
[Cp*Co(CO)I2]2/AgSbF6 |
NaOAc |
DCE |
0 |
3 |
[RuCl2(p-cymene)]2/AgSbF6 |
NaOAc |
DCE |
0 |
4 |
[Cp*RhCl2]2/AgNTf2 |
NaOAc |
DCE |
62 |
5 |
[Cp*RhCl2]2/AgNTf2 |
NaOAc |
Dioxane |
0 |
6 |
[Cp*RhCl2]2/AgNTf2 |
NaOAc |
Toluene |
0 |
7 |
[Cp*RhCl2]2/AgNTf2 |
NaOAc |
MeOH |
0 |
8 |
[Cp*RhCl2]2/AgNTf2 |
NaOAc |
DCE |
43 |
9 |
[Cp*RhCl2]2/AgNTf2 |
KOAc |
DCE |
35 |
10 |
[Cp*RhCl2]2/AgNTf2 |
CsOAc |
DCE |
0 |
11c |
[Cp*RhCl2]2/AgNTf2 |
NaOAc |
DCE |
79 |
12d |
[Cp*RhCl2]2/AgNTf2 |
NaOAc |
DCE |
93 |
13d |
[Cp*RhCl2]2 |
NaOAc |
DCE |
0 |
With the optimized reaction conditions in hand, the scope and limitation of various 2-acetyl-1-arylhydrazines 1a–1q with α-benzoyl sulfoxonium ylide (2a) were further investigated, as shown in Scheme 3. As expected, some functional groups, such as methyl (3ba–3da, 79–89%), methoxy (3ea 87%), butyl (3fa, 81%; 3ga, 86%), and phenyl (3ha, 83%), were well compatible under the reaction conditions with good yields. Remarkably, halogen atoms (F, Cl, Br, and I) linked to the benzene rings of substrates 1i–1n were maintained in the structures of products 3ia–3na (78–87%), suggesting that further manipulation may produce additional useful compounds. Relatively low yields were observed in the reactions of substrates 1o, 1p, and 1q bearing the strong electron-withdrawing groups CF3, COOMe, and CN on their benzene rings (3oa–3qa, 60–76%).
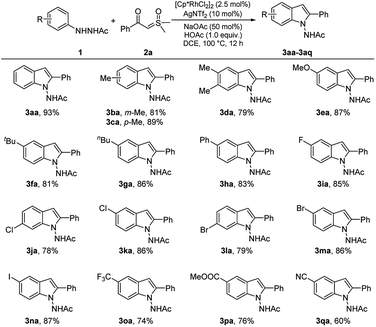 |
| Scheme 3 Substrate scope of 2-acetyl-1-arylhydrazines. Reaction conditions: arylhydrazines (1a–1q, 0.2 mmol), α-benzoyl sulfoxonium ylide (2a, 0.24 mmol, 47.1 mg), [Cp*RhCl2]2 (2.5 mol%, 3.1 mg), AgNTf2 (10 mol%, 7.8 mg), NaOAc (50 mol%, 8.2 mg) and HOAc (1.0 equiv., 12.0 mg) in DCE (1.0 mL) at 100 °C under a nitrogen atmosphere for 12 h. Isolated yield. | |
Then, the scope of (hetero)aroyl sulfoxonium ylides were also examined (Scheme 4). Benzoyl-substituted sulfoxonium ylides bearing a variety of important functional groups, such as electron-donating groups (Me, OMe, phenyl), the halogens (F, Cl), and electron-withdrawing groups (CF3, COOMe) at the ortho, meta, and para positions of the phenyl ring reacted smoothly with 1a to afford the corresponding products (3ab–3aj) in moderate to high yields (54%–90%). The reactants could contain a naphthalene or furan/thiophene ring and the corresponding products (3ak–3am) were obtained in 71%, 95%, and 93% yields, respectively. Moreover, the sulfoxonium ylides were not limited to (hetero)aryl-substituted substrates, an alkenyl (3an, 81%) and several alkyl substrates (3ao–3aq, 67–82%) were also compatible.
 |
| Scheme 4 Substrate scope of (hetero)aroyl sulfoxonium ylides. Reaction conditions: 2-acetyl-1-phenylhydrazine (1a, 0.2 mmol, 30.0 mg), (hetero)aroyl sulfoxonium ylides (2a–2p, 0.24 mmol), [Cp*RhCl2]2 (2.5 mol%, 3.1 mg), AgNTf2 (10 mol%, 7.8 mg), NaOAc (50 mol%, 8.2 mg) and HOAc (1.0 equiv., 12.0 mg) in DCE (1.0 mL) at 100 °C under a nitrogen atmosphere for 12 h. Isolated yield. | |
To further explore the practicability of our methodology, the Rh(III)-catalyzed annulation was scaled up to the gram scale (Scheme 5). The product 3aa was also isolated in excellent yield (91%) even with a reduced catalyst loading.
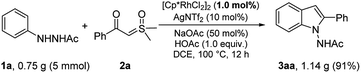 |
| Scheme 5 Gram-scale synthesis. | |
For insight into the mechanism of this reaction, control experiments were performed (Scheme 6). The deuterium kinetic isotope effect was investigated by conducting an intermolecular competition reaction between 1a and 1a-d5. The 3.3
:
1 ratio of 3aa to 3aa-d4 demonstrated that the cleavage of the aromatic C–H bond is probably involved in the turnover-limiting step (eqn (1)). A 2.4
:
1 ratio of 3ca to 3pa was observed in the intermolecular competition reaction between 1c and 1p (eqn (2)), indicating that C–H activation probably occurs through an electrophilic aromatic substitution (SEAr) process.
 |
| Scheme 6 Mechanistic studies. | |
Based on previous reports11–13 and our experimental outcomes, a plausible mechanism is shown in Scheme 7. First, the active cationic rhodium catalyst species [Cp*RhX2] is formed through the reaction of the precatalyst [Cp*RhCl2]2 with AgNTf2 or NaOAc. The coordination of 1a to rhodium catalyst species and subsequent ortho C–H bond activation generates cationic five-membered rhodacyclic intermediate A with the release of HX (X = NTf2 or OAc). Next, sulfoxonium ylide 2a reacts with intermediate A to form Rh(III) intermediate B, which in turn gives the reactive carbene species C by α-elimination of DMSO. Subsequently, a migratory insertion of carbene group into the Rh–C bond to afford a six-membered rhodacycle intermediate D. Then, protonolysis of the intermediate D releases acylmethylated intermediate E with regenerating active [Cp*RhX2]. Finally, the ketone carbonyl E could undergo keto–enol tautomerism and cyclization in the presence of HOAc to give the desired product 3aa.
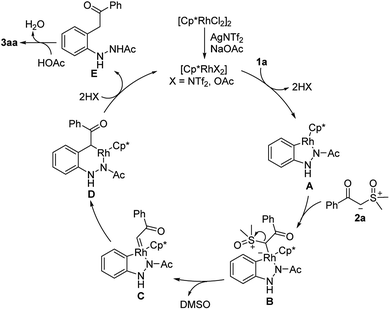 |
| Scheme 7 Proposed mechanism. | |
Conclusions
In summary, we have developed a Rh(III)-catalyzed synthesis of 2-arylindoles from easily available arylhydrazines and sulfoxonium ylides under mild conditions. The protocol is useful to prepare various 2-arylindoles because of its high atom economy, broad substrate scope, and simple procedure. The synthesis could be easily scaled up to gram scale even with a reduced catalyst loading.
Conflicts of interest
There are no conflicts to declare.
Acknowledgements
This work was financially supported by the National Natural Science Foundation of China (21961024 and 21961025), Inner Mongolia Natural Science Foundation (2018JQ05, 2020BS02009, and 2020BS02014), Inner Mongolia Autonomous Region Science & Technology Planning Project for Applied Technology Research and Development (2019GG261), Inner Mongolia Autonomous Region Funding Project for Science & Technology Achievement Transformation (CGZH2018156) and IMUN Doctoral Research Startup Fund Project (BS481 and BS560).
Notes and references
-
(a) D. A. Horton, G. T. Bourne and M. L. Smythe, Chem. Rev., 2003, 103, 893–930 CrossRef CAS;
(b) M. Somei and F. Yamada, Nat. Prod. Rep., 2005, 22, 73–103 RSC;
(c) T. Kawasaki and K. Higuchi, Nat. Prod. Rep., 2005, 22, 761–793 RSC;
(d) A. J. Kochanowska-Karamyan and M. T. Hamann, Chem. Rev., 2010, 110, 4489–4497 CrossRef CAS;
(e) S. A. Patil, R. Patil and D. D. Miller, Future Med. Chem., 2012, 4, 2085–2115 CrossRef CAS.
-
(a) D. R. Stuart, P. Alsabeh, M. Kuhn and K. Fagnou, J. Am. Chem. Soc., 2010, 132, 18326–18339 CrossRef CAS;
(b) H. Johansson, M. W. Boesgaard, L. Nørskov-Lauritsen, I. Larsen, S. Kuhne, D. E. Gloriam, H. Brauner-Osborne and D. S. Pedersen, J. Med. Chem., 2015, 58, 8938–8951 CrossRef CAS;
(c) P. Prasad, P. G. Shobhashana and M. P. Patel, R. Soc. Open Sci., 2017, 4, 170764 CrossRef;
(d) C.-C. Tseng, G. Baillie, G. Donvito, M. A. Mustafa, S. E. Juola, C. Zanato, C. Massarenti, S. D. Angelo, W. T. A. Harrison, A. H. Lichtman, R. A. Ross, M. Zanda and I. R. Greig, J. Med. Chem., 2019, 62, 5049–5062 CrossRef CAS.
-
(a) R. B. V. Order and H. G. Lindwall, Chem. Rev., 1942, 30, 69–96 CrossRef;
(b) B. Robinson, Chem. Rev., 1963, 63, 373–401 CrossRef.
-
(a) R. C. Larock and E. K. Yum, J. Am. Chem. Soc., 1991, 113, 6689–6690 CrossRef CAS;
(b) R. C. Larock, E. K. Yum and M. D. Refvik, J. Org. Chem., 1998, 63, 7652–7662 CrossRef CAS.
-
(a) S. Wagaw, B. H. Yang and S. L. Buchwald, J. Am. Chem. Soc., 1998, 120, 6621–6622 CrossRef CAS;
(b) J. L. Rutherford, M. P. Rainka and S. L. Buchwald, J. Am. Chem. Soc., 2002, 124, 15168–15169 CrossRef CAS.
- L. S. Hegedus, Angew. Chem., Int. Ed., 1988, 27, 1113–1126 CrossRef.
-
(a) T. Satoh and M. Miura, Chem. – Eur. J., 2010, 16, 11212–11222 CrossRef CAS;
(b) D. A. Colby, A. S. Tsai, R. G. Bergman and J. A. Ellman, Acc. Chem. Res., 2012, 45, 814–825 CrossRef CAS;
(c) G. Song and X. Li, Acc. Chem. Res., 2015, 48, 1007–1020 CrossRef CAS;
(d) Y. Minami and T. Hiyama, Acc. Chem. Res., 2016, 49, 67–77 CrossRef CAS;
(e) T. Gensch, M. N. Hopkinson, F. Glorius and J. Wencel-Delord, Chem. Soc. Rev., 2016, 45, 2900–2936 RSC;
(f) J. R. Hummel, J. A. Boerth and J. A. Ellman, Chem. Rev., 2017, 117, 9163–9227 CrossRef CAS;
(g) Z. Nairoukh, M. Cormier and I. Marek, Nat. Rev. Chem., 2017, 1, 0035 CrossRef;
(h) J. Chen, S. Lv and S. Tian, ChemSusChem, 2019, 12, 115–132 CrossRef CAS;
(i) Z. Shen, C. Pi, X. Cui and Y. Wu, Chin. Chem. Lett., 2019, 30, 1374–1378 CrossRef CAS;
(j) L.-Y. Xie, T.-G. Fang, J.-X. Tan, B. Zhang, Z. Cao, L.-H. Yang and W.-M. He, Green Chem., 2019, 21, 3858–3863 RSC;
(k) Q.-W. Gui, X. He, W. Wang, H. Zhou, Y. Dong, N. Wang, J.-X. Tang, Z. Cao and W.-M. He, Green Chem., 2020, 22, 118–122 RSC;
(l) W.-B. He, L.-Q. Gao, X.-J. Chen, Z.-L. Wu, Y. Huang, Z. Cao, X.-H. Xu and W.-M. He, Chin. Chem. Lett., 2020, 31, 1895–1898 CrossRef CAS.
-
(a) N. Yoshikai and Y. Wei, Asian J. Org. Chem., 2013, 2, 466–478 CrossRef CAS;
(b) L. Ackermann, Acc. Chem. Res., 2014, 47, 281–295 CrossRef CAS;
(c) S. Wang, S.-Y. Chen and X.-Q. Yu, Chem. Commun., 2017, 53, 3165–3180 RSC;
(d) G. Duarah, P. P. Kaishap, T. Begum and S. Gogoi, Adv. Synth. Catal., 2019, 361, 654–672 CrossRef CAS;
(e) Y. Xiang, C. Wang, Q. Ding and Y. Peng, Adv. Synth. Catal., 2019, 361, 919–944 CrossRef CAS.
-
(a) M. Gandelman, B. Rybtchinski, N. Ashkenazi, R. M. Gauvin and D. Milstein, J. Am. Chem. Soc., 2001, 123, 5372–5373 CrossRef CAS;
(b) I. K. Mangion, I. K. Nwamba, M. Shevlin and M. A. Huffman, Org. Lett., 2009, 11, 3566–3569 CrossRef CAS;
(c) R. M. P. Dias and A. C. B. Burtoloso, Org. Lett., 2016, 18, 3034–3037 CrossRef CAS;
(d) R. Oost, J. D. Neuhaus, J. Merad and N. Maulide, Sulfur Ylides in Organic Synthesis and Transition Metal Catalysis, ed. V. H. Gessner and R.-U. Bochum, Springer, Berlin, 2017 Search PubMed;
(e) J. Vaitla, A. Bayer and K. H. Hopmann, Angew. Chem., Int. Ed., 2017, 56, 4277–4281 CrossRef CAS;
(f) M. Barday, C. Janot, N. R. Halcovitch, J. Muir and C. Aïssa, Angew. Chem., Int. Ed., 2017, 56, 13117–13221 CrossRef CAS;
(g) X. Wu, S. Sun, J.-T. Yu and J. Cheng, Synlett, 2019, 30, 21–29 CrossRef CAS.
- X.-F. Cui, Z.-H. Ban, W.-F. Tian, F.-P. Hu, X.-Q. Zhou, H.-J. Ma, Z.-Z. Zhan and G.-S. Huang, Org. Biomol. Chem., 2019, 17, 240–243 RSC.
- C. Wu, J. Zhou, G. He, H. Li, Q. Yang, R. Wang, Y. Zhou and H. Liu, Org. Chem. Front., 2019, 6, 1183–1188 RSC.
- Y. Wu, C. Pi, X. Cui and Y. Wu, Org. Lett., 2020, 22, 361–364 CrossRef CAS.
-
(a) W. Xie, X. Chen, J. Shi, J. Li and R. Liu, Org. Chem. Front., 2019, 6, 2662–2666 RSC;
(b) N. Lv, Z. Chen, Z. Liu and Y. Zhang, J. Org. Chem., 2019, 84, 13013–13021 CrossRef CAS.
Footnotes |
† Electronic supplementary information (ESI) available. See DOI: 10.1039/d0ra07701a |
‡ These authors contributed equally to this work. |
|
This journal is © The Royal Society of Chemistry 2020 |