DOI:
10.1039/D0RA04746E
(Paper)
RSC Adv., 2020,
10, 28567-28575
Dye wastewater treatment enabled by piezo-enhanced photocatalysis of single-component ZnO nanoparticles†
Received
29th May 2020
, Accepted 24th July 2020
First published on 3rd August 2020
Abstract
Conventionally, composite materials are usually employed as a catalyst in piezo-photocatalytic dye wastewater treatment. Here, we report the synthesis of ZnO nanoparticles, as a single-component catalyst, by surfactant-assisted precipitation in which the size of ZnO nanoparticles (20–100 nm) can be simply controlled by the use of Tween80 as a surfactant. Although, ZnO nanoparticles exhibited appreciable photocatalytic activities for the degradation of methylene blue (MB) dye, upon the addition of a mechanical force, the photocatalytic dye degradation efficiency was substantially improved. Furthermore, we postulated that the surface properties of ZnO play an important role in charge transfer phenomena based on photoluminescence results together with functional groups on the surface of ZnO. In addition, application of single-component ZnO in piezo-promoted photocatalytic degradation of cationic and anionic dyes was accomplished. Our results regarding the behaviour of single-component ZnO nanoparticles under vibrational energy in addition to their conventional solar harvesting can provide a promising strategy for developing photocatalysts for practical wastewater treatment.
Introduction
Zinc oxide (ZnO) is a multifunctional material which is extensively used in various applications due to its environmental friendliness and diverse properties.1–3 The excellent properties and availabilities of morphological control on the nanoscale make ZnO an excellent material for optoelectronics,4–6 piezoelectric,7,8 nanosensors9 as well as antibacterial applications.10–12 Since ZnO provides a direct wide band gap, photocatalytic activity for application in the elimination of toxic chemicals is also feasible.13,14 Morphology, surface properties and crystal defects are important factors for achieving excellent photocatalytic activity.14–16 However, using a single-component photocatalyst is practically limited because the UV light can be screened by catalyst particles themselves,17 and it has a competitive charge recombination process at a high rate due to inefficient charge separation and transport.18
How to enhance photocatalytic efficiency is the key for practical use of ZnO for dye degradation in wastewater treatment especially in large scale process. Dual energy harvesting in ZnO is one of effective strategies to increase the dye degradation performance. Beside the long-run development of ZnO as photocatalyst, the piezoelectric effect of ZnO has recently applied in wastewater treatment applications.8,19,20 Due to its high effective piezoelectric coefficient d33 (especially for ZnO nanorods at 130 pm V−1 (ref. 21)), ZnO is one of dominated piezoelectric materials. By introducing mechanical energy, induced dipoles with positively and negatively charged surfaces are created. The electric polarization of dielectric materials significantly creates driving force for transport of free charge carriers to the surface and also facilitates interfacial energetic states.20,22 Therefore, these phenomena support the reduction–oxidation of O2 and H2O molecules to form superoxide and hydroxyl radicals for dye degradation as also occurred in the photocatalysis.23,24 Consequently, synergic piezo-promoted photocatalysis is a promising strategy for application in wastewater treatment.
Conventionally, composite catalysts are employed as multi-energy harvesting as piezo-enhanced photocatalysts e.g., ZnO/TiO2 core–shell nanofiber, FeS/ZnO nanowire, carbon/ZnO and CuS/ZnO nanowire.25–29 The photocatalytic efficiency immensely depends on the electron and hole generations, the charge separation, the competitive recombination process, and charge transport along distance between free carriers and active surfaces.30,31 These composite catalysts utilize the benefits of narrow-band-gap semiconductors for expanding light absorption into the visible region and for separating the photogenerated electron–hole pairs by both built-in electric field at the interface32 and driving force induced by piezoelectric effects. This strategy could efficiently enhance the dye degradation efficiency.
Beside the composite catalysts, which may be prepared by a complicated synthetic method, single-component ZnO nanoparticle catalyst can be a simpler alternative. This is because nanoparticle-based catalysts possessed shorter transit time to the active surface area within their lifetime, therefore, the charge recombination and energy loss are suppressed resulting in excellent photocatalytic activities.33 The charge separation of photogenerated electron–hole pairs in nanoparticles can be enhanced by the addition of mechanical energy as mentioned above. Therefore, we speculate that the dye degradation efficiency can be improved by piezo-enhanced photocatalysis with single-component ZnO nanoparticle-based catalysts.
In this work, size-controllable ZnO nanoparticles can be simply synthesized by precipitation method with the assistance of Tween80 (TW) as a surfactant, yielding particle size of 20–100 nm. The photocatalysis and piezo-promoted photocatalysis of as-synthesized and calcined ZnO in methylene blue (MB) degradations were studied which reveal that surface defects related to photocatalysis and piezo-promoted photocatalysis and dye degradation mechanism. In addition, calcined ZnO catalyzed piezo-promoted photocatalysis was also effective for the degradation of rhodamine B (RhB) and thymol blue (TB). Our single-component ZnO nanoparticle-based catalyst is a simpler alternative for potential dual utilization of vibrational and solar energy to deal with wastewater problems.
Experimental methods
Chemicals
Zinc nitrate hexahydrate (Zn(NO3)2·6H2O) was purchased from Sigma-Aldrich. Potassium hydroxide (KOH, 85%) was purchased from RCI-Labscan. Polyoxyethylene sorbitan monooleate or TW was obtained from Sigma-Aldrich. All chemicals were used as-purchased without further purification. De-ionized (DI) water was used as the solvent in all reactions.
Synthesis of ZnO powder
ZnO powder was synthesized by a precipitation method with TW as surfactant. TW was added to a solution of zinc nitrate hexahydrate (0.1125 mM, 80 mL) in DI-water at different TW/Zn2+ molar ratios (0, 0.05, 0.10, 0.20, 0.40, and 0.60). The solution was hold at 60 °C before addition of an aqueous KOH solution (0.45 M, 40 mL) with dosing time of 10 min. The mixture was kept stirring vigorously at 60 °C for 120 min to produce white precipitate. After centrifugation, the supernatant was discarded, and the precipitates were collected. Residue in the obtained particles were removed by using DI-water and ethanol. Finally, the resultant products were dried in an oven at 60 °C overnight to obtain the ZnO powder. Note that ZnOTW-x refers to the ZnO powder synthesized by using TW/Zn2+ molar ratio of x, where x is 0, 0.05, 0.10, 0.20, 0.40, or 0.60.
Characterization techniques
Field emission scanning electron microscope (FE-SEM, JEOL JSM-6335F) was used to investigate the morphology of the resultant products. X-ray diffractometer (XRD, Rigaku MiniflexII) was used to determine the crystal structure of the obtained powder. Transmission Electron Microscope (TEM, JEM 2010) along with selected area electron diffraction (SAED) technique was employed to investigate nanoparticles and confirm phase formation. Thermogravimetric analysis (TGA), undertaken using Thermo plus EV02 (Rigaku), was used to determine residual contents of the samples. Bruker Tensor 27 FT-IR instrument was used to record Fourier-transform infrared (FT-IR) spectra of the samples with KBr pellets (BDH, 98.5%) in the range of 400–4000 cm−1. Particle surface area was evaluated by Brunauer–Emmett–Teller (BET) technique on Autosorb 1 MP, Quantachrome. Photoluminescence (PL) spectrometer (AvaSpec-ULS2048LTEC) integrated with Ocean optic LED light sources was used to collect PL spectra of the ZnO powder by using excitation wavelength of 345 nm and power of 20 μW.
Photocatalytic and piezo-promoted photocatalytic activities
The photocatalytic and piezo-promoted photocatalytic activities of the samples was determined by decomposition of MB. The ZnO powder with a given amount of 25 or 100 mg was mixed with 100 mL of an aqueous MB (5 ppm, 100 mL). The mixture was first sonicated in an ultrasonic bath (120 W, 40 kHz) for 2 min. To obtain adsorption–desorption equilibrium, the suspension was then stirred for 30 min under dark condition. For photocatalytic experiment, UVA light with peak wavelength of 365 nm and intensity of 940 μW cm−2 was exposed to the suspension with constant stirring. On the other hand, for piezo-promoted photocatalytic test, ultrasonic radiation (120 W, 40 kHz) was additionally applied to the samples. In both experiments, 5 mL aliquots were taken at certain intervals to evaluate the decomposition of MB. They were then centrifuged at 4000 rpm for 5 min to separate ZnO catalyst before UV-vis measurement of supernatants (Specord 50 plus). The amount of MB remaining in the solution was calculated by considering a Ct/C0 ratio, where C0 is the initial concentration of MB and Ct is the residual concentration after UV irradiation time of t. The C0 and Ct concentrations were determined by using the absorbance at 665 nm. The piezo-promoted photocatalyzed degradation of RhB and TB were evaluated similarly to MB. The concentration of dye and catalyst dosage were set at 5 ppm and 1.00 g L−1 (200
:
1 by weight of ZnO
:
MB), respectively. The absorbance at 554, 433 and 596 nm were recorded for RhB, TB (at pH 7) and TB (at pH 12), respectively. Total Organic Carbon (TOC) was analyzed with AJ-Analyzer multi N/C 3100 using standard method 5310.
Results and discussion
Crystallinity and morphology
XRD analysis was performed to confirm crystalline phase purity of the white precipitates. The XRD patterns of these samples are shown in Fig. S1 (ESI†). It is seen that the XRD peaks of all samples at 31.8°, 34.4°, 36.2°, 47.5°, and 56.6° are corresponding to the reflection from (100), (002), (101), (102), and (110) planes of the ZnO wurtzite structure (JCPDS 36-1451). In addition, there is no characteristic peak of other impurities or intermediate species. These characteristics imply that only single-phase ZnO was synthesized by our method.
The grain size and morphology of the ZnO powder were observed by using FE-SEM technique. The observed images are demonstrated in Fig. 1. The grain size of the particles was determined by using ImageJ software. It was found that the average grain size of ZnO powder prepared by a conventional precipitation method is approximately 235 nm with standard deviation (SD) of 87 nm (see ESI† for particle size distribution in Fig. S2,† lattice parameters and crystallite size in Table S1†). By adding TW at molar ratio of 0.05, the grain size of ZnOTW-0.05 nanoparticles significantly decreased to 92 nm (SD = 32 nm). The smallest average grain size of about 40 nm (SD = 10 nm) was obtained with ZnOTW-0.20. It is likely that no more reduction of grain size is observed even though the amount of TW was increased to 0.40 and 0.60 (as seen in Fig. S3† in ESI†). Therefore, the investigated results suggest that the 0.20 molar ratio is the optimum value for size reduction. Non-ionic surfactant TW was employed as the directing agent for the formation of ZnO nanoparticles. Dispersion of TW in the aqueous solution forms the micellar structures in which the hydrophobic tails reside inside the micellar core while hydrophilic heads point toward the aqueous solution (see ESI, Fig. S4†). The hydrophilic heads contain several hydroxyl and polyether which functions as a chelating ligand for Zn2+ ions. Consequently, the size of micelle plays an important role in the size of ZnO nanoparticle. After the addition of base, OH− ions react with Zn2+ ions to form zinc hydroxide in which the precipitation occurs when the pH value reaches the stoichiometric amount for the precipitation. Subsequently, heating the solution at 60 °C leads to the formation of ZnO nanoparticles.
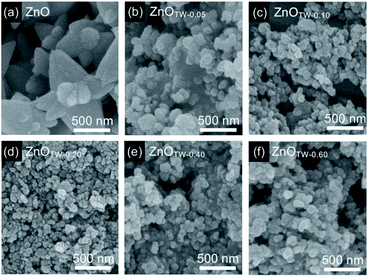 |
| Fig. 1 FE-SEM images of the ZnO nanopowder synthesized by using TW/Zn2+ molar ratio at (a) 0, (b) 0.05, (c) 0.10, (d) 0.20, (e) 0.40, and (f) 0.60. | |
TEM observation and SAED technique were carried out for further examining the particle size and crystallinity of ZnOTW-0.20 nanoparticles. The TEM image and the SAED pattern are shown in Fig. S5 (see ESI†). It is seen that the average primary particles size observed by TEM technique is at 25 nm (SD = 11). This value is slightly smaller than that of the one observed by FE-SEM technique due to the edges of the particles were more clearly visible in the images system leading to higher accuracy in measurement of primary particles size. Moreover, SAED pattern from a group of particles reveals ring spacing characteristics of ZnO wurtzite structure for (100), (002), (101), (102), and (110) planes. The SAED analysis confirmed the crystal structure as examined by XRD.
Surface properties
FT-IR was carried out to observe the surface properties of the synthesized ZnO powders (ZnOTW-0, ZnOTW-0.20, and ZnOTW-0.60) in comparison with pure TW as shown in Fig. 2. The bands at 1358 cm−1 and 1460 cm−1 in the FT-IR spectrum of pure TW are accounted for symmetrical and asymmetrical bending of –CH3, respectively. While, the asymmetrical and symmetrical stretching vibrations of –CH2– correspond to the bands at 2920 cm−1 and 2856 cm−1, respectively.34 For all ZnOTW samples, the broad bands at approximately 3383 cm−1 and the small peaks at 1641 cm−1 which is owing to surface O–H stretching and C
O stretching, respectively.35,36 Furthermore, the band at 1384 cm−1 refers to the asymmetric stretching of C
O from the ester group (–COO–).11,37 On the other hand, the characteristic wavenumber of 420 cm−1 was observed which is ascribed to Zn–O stretching.36,37 This observation implies that the adsorption of TW on the surface of ZnO nanoparticles is negligible.
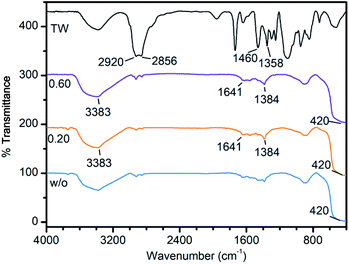 |
| Fig. 2 FT-IR spectra of pure TW and ZnO nanopowder synthesized using TW/Zn2+ with molar ratio at 0, 0.20, and 0.60. | |
Thermogravimetric analysis (TGA) curves (see Fig. S6 in ESI†) reveal that organic residues existed in the ZnO, ZnOTW-0.20, and ZnOTW-0.60 samples for 3.08%, 3.77%, and 4.09% by weight, respectively. These values imply that ZnOTW-0.60 contains slightly higher amount of residue comparing to ZnOTW-0.20 (4.09–3.77 = 0.32%) and ZnO (4.09–3.08 = 1.01%), respectively. The higher residue amount is attributed to larger molar percentage of TW molecules during the synthesis. Since particle size of those samples observed by FE-SEM (Fig. 1d and f) does not show significant difference, ZnOTW-0.20 is chosen for further investigation and application as single-component catalyst. The nitrogen adsorption–desorption isotherms analyzed by BET analysis for ZnOTW-0 and ZnOTW-0.20 nanoparticles are presented in Fig. S7† (see ESI†). The observed isotherms of ZnOTW-0 and ZnOTW-0.20 are corresponded to non-porous materials due to their low N2-absorbed volume even relative pressure reached a point of 0.95. However, ZnOTW-0.20 can also have the pore size distribution in the macropore region due to the intraparticular void generated from agglomeration and aggregation phenomena of nanoparticles which are measured above the relative pressure of 0.95. Therefore, the synthesized ZnO nanopowder is non-porous materials. In addition, it was found that the ZnOTW-0.20 achieved a BET surface area of 29.9 m2 g−1, which is significantly higher than that of the ZnOTW-0 (9.3 m2 g−1). This characteristic can be explained by the different in particle size as monitored by FE-SEM images in Fig. 1. Accordingly, the above analyses indicate that the use of TW molecules in a precipitation method can reduce the particle size of ZnO with organic residues of less than 5% by weight and significantly increase the BET surface area.
Photocatalytic activities of ZnOTW-0.20 nanoparticles
ZnOTW-0.20 nanoparticles were selected as single-component catalyst for photodegradation of MB due to their excellent surface properties among the ZnO and ZnOTW-60 as mentioned above. The ZnO catalyst dosages of 0.25 and 1.00 g L−1 (50
:
1 and 200
:
1 by weight of ZnO
:
MB, respectively) was used for initial aqueous MB solution of 5 ppm. The normalized concentration of MB in the presence of ZnOTW-0.20 was plotted against UV irradiation time in Fig. 3. There is no significant photodegradation of MB molecules for the solution without ZnO catalyst under UV irradiation for 120 min. As seen in Fig. 3a, the as-synthesized ZnOTW-0.20 nanoparticles with dosages of 0.25 g L−1 showed significant photodegradation in comparison with the one without catalyst. However, with increasing dosage to 1.00 g L−1 provides slightly higher photocatalytic activity than dosage of 0.25 g L−1. Even though the amount of catalyst was increased, there was no dramatic change in photodegradation. This phenomenon may be explained by the surface defects of ZnO nanoparticles (as observed by FT-IR and TGA analyses) which may serve as surface recombination centers for photogenerated electrons and holes, causing less hydroxyl radical formation.33
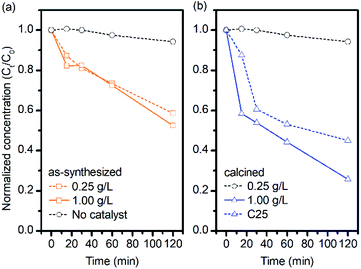 |
| Fig. 3 Photodegradation efficiency of MB solutions in 0.25 g L−1 and 1.00 g L−1 mixtures of (a) as-synthesized ZnOTW-0.20 and (b) calcined ZnOTW-0.20 under exposure to UV light. | |
In order to remove the surface residues on the catalyst, the ZnOTW-0.20 powder was calcined at 600 °C for 1 h. At such temperature, most of organic residuals were efficiently removed as confirmed by TGA analysis (Fig. S6†). Comparing the photocatalytic properties of the as-synthesized ZnOTW-0.20 nanoparticles, the calcined ZnOTW-0.20 catalyst achieved significant change in photodegradation of MB as seen in Fig. 3b. Especially, for calcined ZnOTW-0.20 with dosage of 1.00 g L−1, the residual MB concentration at 120 min was approximately half of the non-calcined one at the same conditions. These results suggested that our ZnOTW-0.20 nanoparticles present the photocatalytic activity and the photodegradation efficiency can be enhanced by calcination of the ZnO nanopowder.
In order to get insight into the effects of calcination on semiconducting properties, various spectroscopic techniques were carried out. PL spectra was recorded at room temperature to evaluate surface and native defects of the as-synthesized ZnOTW-0.20 and the calcined one. By using excitation wavelength of 345 nm, a band in the ultraviolet emission (370–400 nm) and a broadband in the visible region (400–700 nm) were observed. The experimental PL spectra were fitted with Voigt function and they were well divided into several curves as seen in Fig. 4. The PL emissions of the as-synthesized ZnOTW-0.20 can be seen in four different peaks (P1–P4), while the calcined ZnOTW-0.20 show only three peaks (P1–P3). The UV peak (P1) is related to near-band-edge (NBE) emissions which concern emissions of free exciton (FX), bound exciton (BX) and their corresponding phonon replicas.38–41 Red spectral shift (10 nm) and reduction in intensity for P1 peak were revealed after calcination. These behaviors were observed in some cases for post treatment of ZnO at high temperature.42–44 The spectral shift could not be related to the quantum confinement effect since the particle size of the ZnOTW-0.20 is much larger than the Bohr radius of ZnO (2.34 nm).45 However, possible reasons behind this spectral shift could be either different concentration of native defects38,41 in the calcined ZnOTW-0.20 or variation in electron-phonon coupling38,46 after calcination.
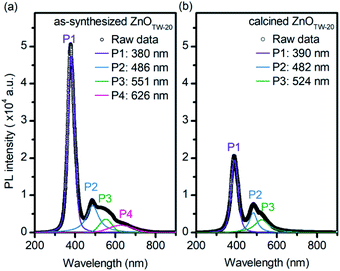 |
| Fig. 4 Room temperature PL spectra of (a) as-synthesized ZnOTW-0.20 and (b) calcined ZnOTW-0.20. The experimental raw data and the fitted curves are represented by open circles and solid lines, respectively. | |
On the other hand, blue (P2), green (P3), and orange-red (P4) emissions of ZnO have been well described by various reports.41,47,48 The blue P2 peak is often corresponded to oxygen vacancies49 or Zn interstitials47 which are the native defects of ZnO. The green P3 emission is attributed to the singly ionized oxygen vacancies.41,47 Both blue and green emissions were observed in the as-synthesized ZnOTW-0.20 and the calcined sample with comparable peak intensities, implying that the calcination did not significantly affect the presence of native defects. However, the orange-red P4 peak disappeared in the PL spectra of calcined ZnOTW-0.20. The orange-red emission was ascribed to the excess oxygen on the ZnO surface, which relates to the surface modifications and heat treatment.41,43,50 The observed behavior implies that the surface residuals of ZnOTW-0.20 was efficiently removed by the calcination as also confirmed by the TGA (Fig. S6†).
Bright-field TEM and FE-SEM observations were also used to investigate the particle morphology and size of the calcined ZnOTW-0.20 nanoparticles as demonstrated in Fig. 5. Calcination at 600 °C does not only remove organic residuals on the surfaces but also improve uniformity. After the calcination, the change from irregularly equiaxed grain (Fig. 1d) to a homogeneously spherical morphology (Fig. 5a) was observed. In addition, average grain size of calcined sample is increased (58 nm SD = 15 nm vs. 40 nm, SD = 10 nm for uncalcined). It was found that the calcination did not significantly affect the crystallinity as shown in Fig. 5a. The calculated crystallite size of calcined sample is negligible (see Table S1 in ESI†). Altogether, these results suggested that surface organic group creates surface trap as indicated by PL results. These centers increase the possibility for charge recombination and obstructs the contact of reactants and photogenerated charge carrier on catalyst surface.51
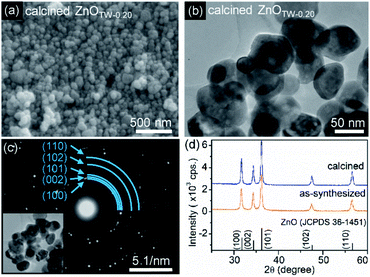 |
| Fig. 5 (a) A SEM image of the calcined ZnOTW-0.20, (b) a bright field TEM image taken from the calcined ZnOTW-0.20, (c) a diffraction pattern of a group of calcined ZnOTW-0.20 particles shown in the image inset (d) XRD patterns of calcined ZnOTW-0.20 and as-synthesized ZnOTW-0.20. | |
Piezo-promoted photocatalytic activities of ZnOTW-0.20 nanoparticles
Further improvement in the degradation of MB was performed by applying ultrasonic wave to promote the excitation of excitons and dynamics of photogenerated electrons and holes. The piezo-promoted photocatalysis of both as-synthesized ZnOTW-0.20 and calcined ZnOTW-0.20 under ultrasonic radiation and UV light is seen in Fig. 6. In addition, the rate constant (k) in photocatalysis and piezo-photocatalysis were determined as shown in Table S3 (see ESI†). As-synthesized ZnOTW-0.20 catalyst shows the degradation of MB at catalyst dosages of 1.00 g L−1 (200
:
1 by weight) faster than that of 0.25 g L−1 (50
:
1 by weight). These results indicate that catalyst dosage of 200 mg spends at the minimum of 120 min to degrade 1 mg of MB. It should be noted that the surface area is increased as the catalyst dosage increased. However, the interparticular quenching between piezo-induced positive charge and negative charge52 dominates over the higher active surface area.
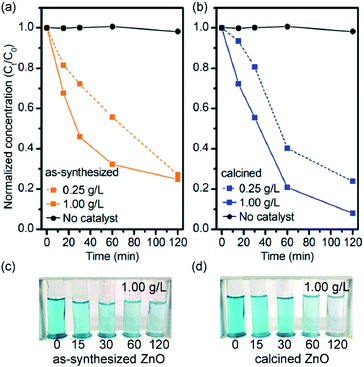 |
| Fig. 6 Piezo-promoted photodegradation efficiency of MB solutions in 0.25 g L−1 and 1.00 g L−1 mixtures of (a) as-synthesized ZnOTW-0.20 and (b) calcined ZnOTW-0.20 under ultrasonic radiation and UV light with their optical images of MB in 1.00 g L−1 mixtures solution in (c) and (d), respectively. | |
In addition, comparing the photodegradation efficiency of the as-synthesized ZnOTW-0.20 in the absence (Fig. 3a) and presence (Fig. 6a) of ultrasonic wave, MB was more efficiently degraded under dual ultrasonic and UV stimulation at both catalyst dosages. These characteristics may be explained by an increase in photogenerated electrons and holes due to piezoelectric-driven internal field.20,22 The internal field, generated by photoelectric effect under the presence of ultrasonic wave, promotes the charge extraction of photogenerated excitons into free electrons and free holes. Then, these free charge carriers move to the respective charged surfaces for reduction-oxidation of O2 and H2O molecules. Therefore, this behavior could reduce the drawbacks of ZnO surface residuals as mentioned above and enhance the reaction rate at the beginning. The mechanical force is even more pronounced in the case of the calcined ZnOTW-0.20 in which provided significantly higher degradation efficiency than that of the as-synthesized catalyst. The rate constant of as-synthesized catalyst is inferior to calcined catalyst with the same dosage (Fig. 6b) although they possessed higher surface area (see Table S2†). This result may be explained by drawbacks of surface residuals in as-synthesized catalysts. Therefore, mechanical force can improve MB degradation under photocatalytic even the low catalyst loading and shorter reaction time.
After having established piezo-promoted photocatalyst for the degradation of MB as a representative of small molecule cationic dye, the generality of the degradation by catalyst to other dyes was further investigated. Therefore, RhB and TB were selected as a candidate for large molecule cationic dye and anion dye, respectively. Piezo-enhanced photocatalytic degradations of RhB and TB with calcined ZnOTW-0.20 are illustrated in Fig. 7. Significant ZnO catalyzed degradation of RhB was found with almost 90% degradation. While the ZnO catalyzed degradation of TB is slightly less effective (ca. 81% degradation). It should be noted that the TB degradation of 48% was also found in the control experiment suggesting the photolysis of TB. In addition, due to the overlapped absorption of TB and residual ZnO, pH of photodegraded TB solution was adjusted from 7 to 12, resulting in the color changes from yellow to blue. The Ct and C0 values of TB could be determined from the absorbance at 596 nm. The normalized concentration of TB obtained after pH adjustment to 12 was consistent to the one of pH at 7 as seen in Fig. 7. According to the MB degradation in Fig. 6b, the degradation efficiency of RhB and TB is comparable to that of MB within 2 hours (Table S4†). Therefore, ZnO effectively catalyzed the degradation of cationic and anion dyes via piezo-enhanced photocatalysis. The decolorization of organic dyes may not imply that the dye is completely degraded into CO2 and H2O. TOC values related to the total concentration of organic content in the solution and reflects the degradation efficiency of the photocatalytic process. After the complete decolorization of the solution, the TOC values slightly decreased (Table S4†). This result suggests that the products obtained from the piezo-promoted photocatalytic process presumably are CO2, small organic molecules and degraded dyes.
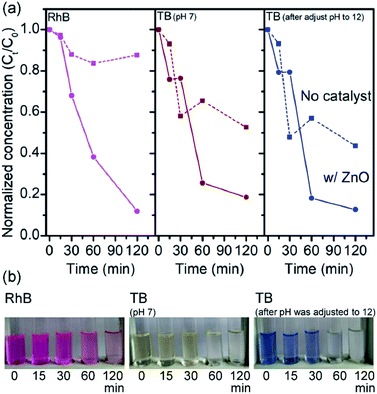 |
| Fig. 7 (a) Piezo-promoted photodegradation of RhB, TB at pH 7, and the photodegraded TB solution after pH was adjusted to 12 with their corresponding optical images in (b). | |
This work demonstrated that ZnO nanoparticles obtained from TW-assisted precipitation exhibited appreciable organic-dye degradation efficiency under piezo-promoted photocatalysis. Dye degradation efficiencies are comparable to ones reported by other previous works as shown in Table S5.† In addition, this work also highlights the merit of single-component catalyst and simple synthetic method which is alternative to other complex synthetic processes.
Dye degradation mechanisms
The mechanism of piezo-enhanced photocatalytic degradation of organic dyes by ZnO nanoparticles are tentatively proposed in Fig. 8. When UV light irradiates to ZnO semiconductors, excited electrons at the conduction band (CB) and holes at the valence band (VB) are created (Fig. 8a), forming electron–hole pairs or excitons. These bound charge carriers are required to be separated into free charges within their lifetimes for participating in the reduction–oxidation reaction to generate superoxide and hydroxyl radicals. However, the charge recombination rate in ZnO is faster because there is no driving force for separating and mobilizing electrons and holes to the surfaces of ZnO nanoparticles. However, for the dual stimulation by photon and ultrasonic wave on ZnO catalyst, the built-in electric field created by charge polarization provides driving force for both excitons excitation and excitons separation as shown in Fig. 8b. Due to the suppression in charge recombination by this internal field, there are more free charge carriers acting as oxidant and reductant simultaneously, thereby providing access to reactive species such as superoxide radical (O2˙−) and hydroxyl radicals (·OH). Then, the degradation of organic dyes possibly involves to those reactive species. However, free charge carriers may be trapped at the surface states formed by the organic residues or surface defects, leading to reduction in reactive species. Therefore, removal of the surface residues is crucial for enhancing the dye degradation performance.
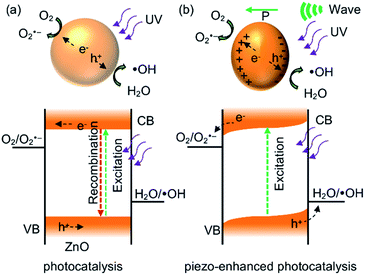 |
| Fig. 8 Schematic representation of mechanisms in (a) photocatalysis and (b) piezo-enhanced photocatalysis of ZnO nanoparticles. | |
To investigate how these reactive species were involved in the process, several experiments were conducted. At first, degradation of MB was carried out in the presence of ZnO and different scavengers of the reactive species. The presence of AgNO3 (electron scavenger) improves the catalytic activity. It can be explained by Ag+ accepts an electron to generate silver which can serve as a cocatalyst in dye degradation. Indeed, Ag–ZnO composites are known to photocatalyze MB degradation.53 The addition of t-butanol (hydroxyl radical scavenger) inhibits the photocatalytic activity. This result indicates the generation of hydroxyl radical is a responsible species for the dye degradation.
Conclusions
We reported a single-component ZnO nanoparticle catalyst, synthesized by surfactant-assisted precipitation at low temperature (60 °C), for dye wastewater treatment via piezo-enhanced photocatalysis. The applied mechanical force on ZnO nanoparticles was found to remarkably enhance photocatalytic degradation. After thoroughly characterizations by various spectroscopic techniques, it was found that organic surface residuals in as-synthesized ZnO hindered the photocatalytic degradation efficiency. Calcined ZnO nanoparticles showed better photocatalytic and piezo-enhanced photocatalytic performances. In addition, surface properties of ZnO shed light on catalytic activities and piezo-enhanced photocatalytic degradation mechanisms. Therefore, photocatalytic MB degradation efficiencies could be synergistically enhanced by the vibrational force with the low catalyst loading and shorter reaction time. Additionally, piezo-promoted photocatalyst does not only degrade cationic dye but also applicable for anion dye. This work demonstrated that single-component ZnO nanoparticle catalyst could be alternative to commonly used ZnO nanowires and nanosheets.
Conflicts of interest
The authors declare that they have no competing interests.
Acknowledgements
This work was financially supported by Chiang Mai University. YC, SY and PR thanks Development and Promotion of Science and Technology Talent Projects (DPST) through research fund for DPST graduates with first placements. The authors would like to acknowledge Dr Pimluck Kijjanapanich of Faculty of Engineering, Chiang Mai University.
Notes and references
- J. L. Gomez and O. Tigli, Zinc oxide nanostructures: from growth to application, J. Mater. Sci., 2013, 48, 612–624 CrossRef CAS.
- Q. Zhang, C. S. Dandeneau, X. Zhou and C. Cao, ZnO nanostructures for dye-sensitized solar cells, Adv. Mater., 2009, 21, 4087–4108 CrossRef CAS.
- A. Naveed Ul Haq, A. Nadhman, I. Ullah, G. Mustafa, M. Yasinzai and I. Khan, Synthesis Approaches of Zinc Oxide Nanoparticles: The Dilemma of Ecotoxicity, J. Nanomater., 2017, 2017, 1–14 CrossRef.
- P. Ruankham, D. Wongratanaphisan, A. Gardchareon, S. Phadungdhitidhada, S. Choopun and T. Sagawa, Full coverage of perovskite layer onto ZnO nanorods via a modified sequential two-step deposition method for efficiency enhancement in perovskite solar cells, Appl. Surf. Sci., 2017, 410, 393–400 CrossRef CAS.
- F. Yang, D.-W. Kang and Y.-S. Kim, Improved interface of ZnO/CH3NH3PbI3 by a dynamic spin-coating process for efficient perovskite solar cells, RSC Adv., 2017, 7, 19030–19038 RSC.
- F. Fleischhaker, V. Wloka and I. Hennig, ZnO based field-effect transistors (FETs): solution-processable at low temperatures on flexible substrates, J. Mater. Chem., 2010, 20, 6622–6625 RSC.
- L. Wang, S. Liu, Z. Wang, Y. Zhou, Y. Qin and Z. L. Wang, Piezotronic Effect Enhanced Photocatalysis in Strained Anisotropic ZnO/TiO2 Nanoplatelets via Thermal Stress, ACS Nano, 2016, 10, 2636–2643 CrossRef CAS PubMed.
- X. Xu, Y. Jia, L. Xiao and Z. Wu, Strong vibration-catalysis of ZnO nanorods for dye wastewater decolorization via piezo-electro-chemical coupling, Chemosphere, 2018, 193, 1143–1148 CrossRef CAS PubMed.
- M. Thepnurat, T. Chairuangsri, N. Hongsith, P. Ruankham and S. Choopun, Realization of Interlinked ZnO Tetrapod Networks for UV Sensor and Room-Temperature Gas Sensor, ACS Appl. Mater. Interfaces, 2015, 7(43), 24177–24184 CrossRef CAS PubMed.
- S. A. Ansari, M. M. Khan, M. O. Ansari, J. Lee and M. H. Cho, Biogenic synthesis, photocatalytic, and photoelectrochemical performance of Ag–ZnO nanocomposite, J. Phys. Chem. C, 2013, 117, 27023–27030 CrossRef CAS.
- V. Rajendar, C. H. Shilpa Chakra, B. Rajitha, K. Venkateswara Rao, M. Chandra Sekhar, B. Purusottam Reddy and S. H. Park, Effect of TWEEN80 on the morphology and antibacterial properties of ZnO nanoparticles, J. Mater. Sci.: Mater. Electron., 2017, 28, 3272–3277 CrossRef CAS.
- P. Petkova, A. Francesko, M. M. Fernandes, E. Mendoza, I. Perelshtein, A. Gedanken and T. Tzanov, Sonochemical coating of textiles with hybrid ZnO/chitosan antimicrobial nanoparticles, ACS Appl. Mater. Interfaces, 2014, 6, 1164–1172 CrossRef CAS PubMed.
- C. Tian, Q. Zhang, A. Wu, M. Jiang, Z. Liang, B. Jiang and H. Fu, Cost-effective large-scale synthesis of ZnO photocatalyst with excellent performance for dye photodegradation, Chem. Commun., 2012, 48, 2858–2860 RSC.
- S. G. Kumar and K. S. R. K. Rao, Zinc oxide based photocatalysis: tailoring surface-bulk structure and related interfacial charge carrier dynamics for better environmental applications, RSC Adv., 2015, 5, 3306–3351 RSC.
- S. Lan, L. Liu, R. Li, Z. Leng and S. Gan, Hierarchical hollow structure ZnO: synthesis, characterization, and highly efficient adsorption/photocatalysis toward congo red, Ind. Eng. Chem. Res., 2014, 53, 3131–3139 CrossRef CAS.
- D. Lin, H. Wu, R. Zhang and W. Pan, Enhanced photocatalysis of electrospun Ag–ZnO heterostructured nanofibers, Chem. Mater., 2009, 21, 3479–3484 CrossRef CAS.
- M. Kubo, H. Fukuda, X. J. Chua and T. Yonemoto, Kinetics of Ultrasonic Degradation of Phenol in the Presence
of Composite Particles of Titanium Dioxide and Activated Carbon, Ind. Eng. Chem. Res., 2007, 46, 699–704 CrossRef CAS.
- D. H. Wi, S. Y. Park, S. Lee, J. Sung, J. W. Hong and S. W. Han, Metal-semiconductor ternary hybrids for efficient visible-light photocatalytic hydrogen evolution, J. Mater. Chem. A, 2018, 6, 13225–13235 RSC.
- Y. Zhang, X. Huang and J. Yeom, A Floatable Piezo-Photocatalytic Platform Based on Semi-Embedded ZnO Nanowire Array for High-Performance Water Decontamination, Nano-Micro Lett., 2019, 11, 11 CrossRef CAS.
- Z. Wang, T. Hu, H. He, Y. Fu, X. Zhang, J. Sun, L. Xing, B. Liu, Y. Zhang and X. Xue, Enhanced H2 Production of TiO2/ZnO Nanowires Co-Using Solar and Mechanical Energy through Piezo-Photocatalytic Effect, ACS Sustainable Chem. Eng., 2018, 6, 10162–10172 CrossRef CAS.
- E. S. Nour, O. Nur and M. Willander, Zinc oxide piezoelectric nano-generators for low frequency applications, Semicond. Sci. Technol., 2017, 32, 64005 CrossRef.
- W. Qian, K. Zhao, D. Zhang, C. R. Bowen, Y. Wang and Y. Yang, Piezoelectric Material-Polymer Composite Porous Foam for Efficient Dye Degradation via the Piezo-Catalytic Effect, ACS Appl. Mater. Interfaces, 2019, 11, 27862–27869 CrossRef CAS PubMed.
- Y. Feng, H. Li, L. Ling, S. Yan, D. Pan, H. Ge, H. Li and Z. Bian, Enhanced Photocatalytic Degradation Performance by Fluid-Induced Piezoelectric Field, Environ. Sci. Technol., 2018, 52, 7842–7848 CrossRef CAS PubMed.
- W. Yang, Y. Yu, M. B. Starr, X. Yin, Z. Li, A. Kvit, S. Wang, P. Zhao and X. Wang, Ferroelectric Polarization-Enhanced Photoelectrochemical Water Splitting in TiO2 –BaTiO3 Core–Shell Nanowire Photoanodes, Nano Lett., 2015, 15, 7574–7580 CrossRef CAS PubMed.
- D. Hong, W. Zang, X. Guo, Y. Fu, H. He, J. Sun, L. Xing, B. Liu and X. Xue, High Piezo-photocatalytic Efficiency of CuS/ZnO Nanowires Using Both Solar and Mechanical Energy for Degrading Organic Dye, ACS Appl. Mater. Interfaces, 2016, 8, 21302–21314 CrossRef CAS PubMed.
- X. Guo, Y. Fu, D. Hong, B. Yu, H. He, Q. Wang, L. Xing and X. Xue, High-efficiency sono-solar-induced degradation of organic dye by the piezophototronic/photocatalytic coupling effect of FeS/ZnO nanoarrays, Nanotechnology, 2016, 27, 375704 CrossRef PubMed.
- H. You, Z. Wu, Y. Jia, X. Xu, Y. Xia, Z. Han and Y. Wang, High-efficiency and mechano-/photo- bi-catalysis of piezoelectric-ZnO@ photoelectric-TiO2 core–shell nanofibers for dye decomposition, Chemosphere, 2017, 183, 528–535 CrossRef CAS PubMed.
- M. M. Hossain, B. C. Ku and J. R. Hahn, in Applied Surface Science, Elsevier B.V., 2015, vol. 354, pp. 55–65 Search PubMed.
- H. Shima, M. M. Hossain, I. Lee, S. Son and J. R. Hahn, Carbon–ZnO core–shell nanospheres: facile fabrication and application in the visible-light photocatalytic decomposition of organic pollutant dyes, Mater. Chem. Phys., 2017, 185, 73–82 CrossRef CAS.
- M. Law, L. E. Greene, J. C. Johnson, R. Saykally and P. Yang, Nanowire dye-sensitized solar cells, Nat. Mater., 2005, 4, 455–459 CrossRef CAS PubMed.
- E. Hosono, S. Fujihara, I. Honma and H. Zhou, The Fabrication of an Upright-Standing Zinc Oxide Nanosheet for Use in Dye-Sensitized Solar Cells, Adv. Mater., 2005, 17, 2091–2094 CrossRef CAS.
- X. Guo, Y. Fu, D. Hong, B. Yu, H. He, Q. Wang, L. Xing and X. Xue, High-efficiency sono-solar-induced degradation of organic dye by the piezophototronic/photocatalytic coupling effect of FeS/ZnO nanoarrays, Nanotechnology, 2016, 27, 1–11 CAS.
- J. Theerthagiri, S. Salla, R. A. Senthil, P. Nithyadharseni, A. Madankumar, P. Arunachalam, T. Maiyalagan and H.-S. Kim, A review on ZnO nanostructured materials: energy, environmental and biological applications, Nanotechnology, 2019, 30, 392001 CrossRef CAS PubMed.
- J. Xiong, S. Xiong, Z. Guo, M. Yang, J. Chen and H. Fan, Ultrasonic dispersion of nano TiC powders aided by Tween80 addition, Ceram. Int., 2012, 38, 1815–1821 CrossRef CAS.
- S. K. Sehmi, S. Noimark, S. D. Pike, J. C. Bear, W. J. Peveler, C. K. Williams, M. S. P. Shaffer, E. Allan, I. P. Parkin and A. J. MacRobert, Enhancing the Antibacterial Activity of Light-Activated Surfaces Containing Crystal Violet and ZnO Nanoparticles: Investigation of Nanoparticle Size, Capping Ligand, and Dopants, ACS Omega, 2016, 1, 334–343 CrossRef CAS PubMed.
- D. Ramimoghadam, M. Z. Bin Hussein and Y. H. Taufiq-Yap, Hydrothermal synthesis of zinc oxide nanoparticles using rice as soft biotemplate, Chem. Cent. J., 2013, 7, 136 CrossRef PubMed.
- M. Zare, K. Namratha, K. Byrappa, D. M. Surendra, S. Yallappa and B. Hungund, Surfactant assisted solvothermal synthesis of ZnO nanoparticles and study of their antimicrobial and antioxidant properties, J. Mater. Sci. Technol., 2018, 34, 1035–1043 CrossRef.
- H. Ding, Z. Zhao, G. Zhang, Y. Wu, Z. Gao, J. Li, K. Zhang, N. Pan and X. Wang, Oxygen vacancy: an electron-phonon interaction decoupler to modulate the near-band-edge emission of ZnO nanorods, J. Phys. Chem. C, 2012, 116, 17294–17299 CrossRef CAS.
- A. Singh, K. Senapati, B. Satpati and P. K. Sahoo, Suppression of near band edge emission in specially engineered ZnO twin nanorods, Phys. Chem. Chem. Phys., 2017, 19, 14012–14019 RSC.
- V. V. Khomyak, M. M. Slyotov, I. I. Shtepliuk, G. V. Lashkarev, O. M. Slyotov, P. D. Marianchuk and V. V. Kosolovskiy, Annealing effect on the near-band edge emission of ZnO, J. Phys. Chem. Solids, 2013, 74, 291–297 CrossRef CAS.
- A. B. Djurišić and Y. H. Leung, Optical properties of ZnO nanostructures, Small, 2006, 2, 944–961 CrossRef PubMed.
- D. J. Qiu, H. Z. Wu, A. M. Feng, Y. F. Lao, N. B. Chen and T. N. Xu, Annealing effects on the microstructure and photoluminescence properties of Ni-doped ZnO films, Appl. Surf. Sci., 2004, 222, 263–268 CrossRef CAS.
- B. Panigrahy, M. Aslam, D. S. Misra, M. Ghosh and D. Bahadur, Defect-related emissions and magnetization properties of ZnO Nanorods, Adv. Funct. Mater., 2010, 20, 1161–1165 CrossRef CAS.
- R. Raji and K. G. Gopchandran, ZnO nanostructures with tunable visible luminescence: effects of kinetics of chemical reduction and annealing, J. Sci. Adv. Mater. Devices, 2017, 2, 51–58 CrossRef.
- Y. Gu, I. L. Kuskovsky, M. Yin, S. O'Brien and G. F. Neumark, Quantum confinement in ZnO nanorods, Appl. Phys. Lett., 2004, 85, 3833–3835 CrossRef CAS.
- M. Šćepanović, M. Grujić-Brojčin, K. Vojisavljević and T. Srećković, Defect induced variation in vibrational and optoelectronic properties of nanocrystalline ZnO powders, J. Appl. Phys., 2011, 109, 34313 CrossRef.
- M. J. Al-Saadi, S. H. Al-Harthi, H. H. Kyaw, M. T. Z. Myint, T. Bora, K. Laxman, A. Al-Hinai and J. Dutta, Influence of Atomic Hydrogen, Band Bending, and Defects in the Top Few Nanometers of Hydrothermally
Prepared Zinc Oxide Nanorods, Nanoscale Res. Lett., 2017, 12, 22 CrossRef PubMed.
- C. Belkhaoui, N. Mzabi, H. Smaoui and P. Daniel, Enhancing the structural, optical and electrical properties of ZnO nanopowders through (Al + Mn) doping, Results Phys., 2019, 12, 1686–1696 CrossRef.
- C. Belkhaoui, N. Mzabi, H. Smaoui and P. Daniel, Enhancing the structural, optical and electrical properties of ZnO nanopowders through (Al + Mn) doping, Results Phys., 2019, 12, 1686–1696 CrossRef.
- Y. Zuo, S. Ge, Z. Chen, L. Zhang, X. Zhou and S. Yan, Morphology, optical and magnetic properties of Zn1−xNixO nanorod arrays fabricated by hydrothermal method, J. Alloys Compd., 2009, 470, 47–50 CrossRef CAS.
- S. Zhu and D. Wang, Photocatalysis: Basic Principles, Diverse Forms of Implementations and Emerging Scientific Opportunities, Adv. Energy Mater., 2017, 7(23), 1700841 CrossRef.
- S. Wang, Z. Wu, J. Chen, J. Ma, J. Ying, S. Cui, S. Yu, Y. Hu, J. Zhao and Y. Jia, Lead-free sodium niobate nanowires with strong piezo-catalysis for dye wastewater degradation, Ceram. Int., 2019, 45, 11703–11708 CrossRef CAS.
- M. J. Height, S. E. Pratsinis, O. Mekasuwandumrong and P. Praserthdam, Ag–ZnO catalysts for UV-photodegradation of methylene blue, Appl. Catal., B, 2006, 63, 305–312 CrossRef CAS.
Footnote |
† Electronic supplementary information (ESI) available. See DOI: 10.1039/d0ra04746e |
|
This journal is © The Royal Society of Chemistry 2020 |
Click here to see how this site uses Cookies. View our privacy policy here.