DOI:
10.1039/C8RA03059F
(Paper)
RSC Adv., 2018,
8, 23323-23331
Novel Eu3+-activated Ba2Y5B5O17 red-emitting phosphors for white LEDs: high color purity, high quantum efficiency and excellent thermal stability†
Received
10th April 2018
, Accepted 23rd May 2018
First published on 27th June 2018
Abstract
Eu3+-activated Ba2Y5B5O17 (Ba2Y5−xEuxB5O17; x = 0.1–1) red-emitting phosphors were synthesized by the conventional high temperature solid-state reaction method in an air atmosphere. Powder X-ray diffraction (XRD) analysis confirmed the pure phase formation of the as-synthesized phosphors. Morphological studies were performed using field emission-scanning electron microscopy (FE-SEM). The photoluminescence spectra, lifetimes, color coordinates and internal quantum efficiency (IQE) as well as the temperature-dependent emission spectra were investigated systematically. Upon 396 nm excitation, Ba2Y5−xEuxB5O17 showed red emission peaking at 616 nm which was attributed to the 5D0 → 7F2 electric dipole transition of Eu3+ ions. Meanwhile, the influences of different concentrations of Eu3+ ions on the PL intensity were also discussed. The optimum concentration of Eu3+ ions in the Ba2Y5−xEuxB5O17 phosphors was found to be x = 0.8. The concentration quenching mechanism was attributed to the dipole–dipole interaction and the critical distance (Rc) for energy transfer among Eu3+ ions was determined to be 5.64 Å. The asymmetry ratio [(5D0 → 7F2)/(5D0 → 7F1)] of Ba2Y4.2Eu0.8B5O17 phosphors was calculated to be 3.82. The fluorescence decay lifetimes were also determined for Ba2Y5−xEuxB5O17 phosphors. In addition, the CIE color coordinates of the Ba2Y4.2Eu0.8B5O17 phosphors (x = 0.653, y = 0.345) were found to be very close to the National Television System Committee (NTSC) standard values (x = 0.670, y = 0.330) of red emission and also showed high color purity (∼94.3%). The corresponding internal quantum efficiency of the Ba2Y4.2Eu0.8B5O17 sample was measured to be 47.2%. Furthermore, the as-synthesized phosphors exhibited good thermal stability with an activation energy of 0.282 eV. The above results revealed that the red emitting Ba2Y4.2Eu0.8B5O17 phosphors could be potential candidates for application in near-UV excited white light emitting diodes.
1. Introduction
In recent times, scientific research has been focused on new illumination sources to meet the demands of energy conservation and environmental issues.1 Solid-state lighting (SSL) is a major revolution in the lighting industry.2 Among SSL, inorganic phosphor-converted white light-emitting diodes (pc-WLEDs) are found to prevail in the lighting market owing to an extensive range of applications in versatile fields, such as flat panel displays, WLEDs, bio-markers, solid state lasers, sensors, and high energy radiation detectors.3–10 In particular, WLEDs have attracted much attention due to their better performance such as high luminous efficiency, long operational lifetime, high rendering indices, compactness, low-cost, energy saving and environmental protection to surpass traditional incandescent and fluorescent lamps.11,12
The most currently used WLEDs are fabricated by the combination of InGaN-based blue LED chips with the yellow emitting Y3Al5O12:Ce3+ (YAG:Ce3+) phosphors.13 However, there exist some drawbacks in its practical application, such as poor color rendering index (Ra < 80), and the high correlated color temperature (CCT ∼ 7750 K) owing to the lack of a red spectral component in the visible region.4,14 In order to obtain high-efficiency WLEDs with appropriate CCT, high CRI and better color stability, alternative method is proposed, which combines the near-ultraviolet (n-UV) LED chips with a mixture of red, green and blue emitting phosphors.9 There are some commercial BaMgAl10O17:Eu2+ (blue), SrSi2O2N2:Eu2+ and Ba2SiO4:Eu2+ (green) phosphors, but a high-efficiency, low-cost red-emitting phosphor is still needed.2,15,16 The red-emitting phosphors are an essential component for creating high-quality white light. Currently, the lanthanide-activated sulfide and nitride (e.g., Y2O2S:Eu3+, SrGa2S4:Eu2+, CaS:Eu2+, Sr2Si5N8:Eu2+ and CaAlSiN3:Eu2+) red phosphors are extensively being used for WLEDs.4,12 However, the commercially available red-emitting sulfide phosphors are chemically unstable with low luminous efficiency. Meanwhile, the nitride based phosphors have critical synthesis conditions, such as high temperature (>1800 °C) and high pressure (0.5 MPa N2 pressure).12,13,17,18 The search for non-toxic and highly efficient host materials has attracted researchers. Therefore, it is an urgent task to investigate new red-emitting phosphors for WLEDs with high efficiency, excellent thermal stability and high color purity, which can be excited by n-UV LEDs.
Rare earth ions activated inorganic materials have attractive optical properties because of their distinctive intra-configurational f → f transitions.9 Among all the rare earth ions, Eu3+ ion is the most often used red-emitting activator and exhibits a series of characteristic sharp emissions ascribed to the electronic transitions of 5D0 → 7FJ (J = 0, 1, 2, 3, and 4).19–24 Furthermore, the Eu3+ ion is expected to be one of the most hopeful species that offers optical devices in the red color region and numerous investigations have been executed in different host materials such as silicates25 and phosphates26,27 for the WLEDs application.
As an essential member of the family of luminescent materials, rare earth ions activated borate compounds have been widely investigated, due to their special structure, low sintering temperature, good physical and chemical stability, low cost, excellent optical damage threshold, large band gap, and strong absorption in the n-UV region.28–30 Recently, blue-emitting Ba2Y5B5O17:Ce3+ (ref. 31) and green-emitting Ba2Y5B5O17:Ce3+,Tb3+ (ref. 32) phosphors have been reported. Zhang et al. investigated the Ce3+/Tb3+/Eu3+ tri-doped Ba2Y5B5O17 multi-color emitting phosphors.33
The searching of high-performance red-emitting phosphors for WLEDs, we have systematically investigated in detail luminescence properties of Eu3+ activated Ba2Y5B5O17 red emitting phosphors. To the best of our knowledge, the elaborated spectroscopic properties of Eu3+ singly doped Ba2Y5B5O17 and its potential application in WLEDs have not yet been reported. The phosphors showed intense red emission peaking at 616 nm under a 396 nm excitation. The Ba2Y4.2Eu0.8B5O17 phosphor was found to show the highest emission intensity with internal quantum efficiency (IQE) of 47.2%. Moreover, the Ba2Y4.2Eu0.8B5O17 phosphor also exhibited good thermal stability and high color purity of red emission. The obtained results suggest that the Ba2Y5B5O17:Eu3+ phosphor has potential application for n-UV-pumped WLEDs.
2. Experimental
2.1. Materials and synthesis
Polycrystalline samples of Ba2Y5−xEuxB5O17 (x = 0–1.0) phosphors were synthesized using conventional high temperature solid-state reaction method. Stoichiometric amounts of starting materials BaCO3 (Analytical Reagent, A.R.), Y2O3 (99.99%), H3BO3 (A.R.) and Eu2O3 (99.99%) were weighed, and ground using an agate mortar and pestle to form homogeneous mixtures. The obtained mixtures were then transferred into an alumina crucible and pre-heated at 450 °C for 4 h in air. After that, the samples were reground and sintered in air at 1200 °C for 8 h. Finally, the as-prepared samples were gradually cooled down to room temperature in the furnace and were re-ground into homogeneous powder for further measurements.
2.2. Measurements and characterization
The crystalline phase formation of as-synthesized samples were identified by the powder X-ray diffraction (XRD) patterns using Bruker D8 advance powder diffractometer operated at 40 kV and 40 mA with CuKα radiation (λ = 1.5406 Å). The measurements were carried out in the diffraction angle (2θ) ranging from 10 to 80° with a scanning step of 0.02°. The structural parameters of Ba2Y5B5O17 and Ba2Y4.2Eu0.8B5O17 were refined by the Rietveld refinement method using FullProf_Suite program. The morphology and elemental mapping analyzes of the phosphors were performed using a field-emission scanning electron microscope (FE-SEM; MAIA3 TESCAN). The photoluminescence (PL) and photoluminescence excitation (PLE) spectra as well as the decay curves were recorded using Edinburgh FS5 spectrofluorometer equipped with a 150 W continuous wavelength Xenon lamp and a pulsed Xenon lamp as the excitation source. The IQE of sample was measured by employing a barium sulfate coated integrating sphere attached to the Edinburgh FS5 spectrofluorometer. All the above measurements were carried out at room temperature. Furthermore, the temperature-dependent PL spectra of the phosphors within the temperature range from 303 to 483 K were obtained on the same instrument with a temperature controlling system.
3. Results and discussion
3.1. Phase identification
The XRD patterns of the Ba2Y5−xEuxB5O17 (x = 0, 0.1, 0.4, 0.6, 0.8, and 1.0) phosphors are shown in Fig. 1(a). All the observed diffraction peaks of the as-obtained samples were well consistent with the Powder Diffraction File (PDF No. 00-056-0113) for Ba2Y5B5O17, and also matched well with the reference data.33 No other phase or impurity were noticed in the compositions, and the result distinctly suggested that the as-prepared samples were in single phase and Eu3+ doping did not significantly influence the crystalline structure of the host due to the similar valence and ionic radius of Eu3+ and Y3+ [Eu3+ (r = 1.07 Å), Y3+ (r = 1.02 Å)].33 In the meantime, a small shift in the diffraction peaks were observed after increasing Eu3+ content, (depicted in Fig. 1(b)) because of the ionic radius between Y3+ and Eu3+ [Y3+ (r = 1.02 Å), Eu3+ (r = 1.07 Å)]. According to previous report, the Ba2Y5B5O17 crystallizes as an orthorhombic structure with the space group Pbcn (no. 60).31–33 In order to investigate the crystal structure, the structural parameters of Ba2Y5B5O17 and Ba2Y4.2Eu0.8B5O17 were refined by the Rietveld refinement method using FullProf_Suite program. The observed, calculated and difference of XRD patterns were shown in Fig. 2(a) and (b). The structural parameters are listed in Table 1. All of the observed diffraction peaks showed a good agreement between observed and calculated patterns. The results reveals that Ba2Y5B5O17 crystallizes as an orthorhombic structure with space group Pbcn (no. 60), and its lattice parameters were determined to be a = 17.4486(8) Å, b = 6.64715(29) Å and c = 13.0128(6) Å and V = 1509.28(19) Å3. The refinement factors finally converged to Rp = 2.54%, and Rwp = 3.67%, which was shown in Table S1 (ESI†). Similarly, the refinement results confirms that the cell parameters of Ba2Y4.2Eu0.8B5O17, a = 17.45484(18) Å, b = 6.66655(9) Å, c = 13.06090(18) Å and V = 1519.813(34) Å3, the refinement factors finally converged to Rp = 1.99%, and Rwp = 2.70%. The atomic coordinates were provided in the Table 2. Furthermore, the unit cell structure of Ba2Y5B5O17 is depicted in Fig. 3.
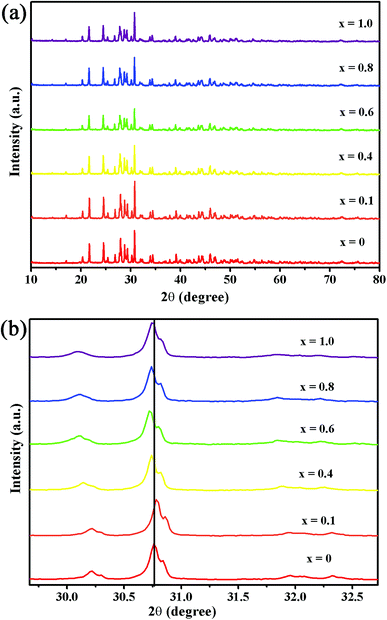 |
| Fig. 1 (a) XRD patterns of as-prepared Ba2Y5−xEuxB5O17 (x = 0, 0.1, 0.4, 0.6, 0.8 and 1.0) phosphors. (b) Magnified XRD patterns for Ba2Y5−xEuxB5O17 (x = 0, 0.1, 0.4, 0.6, 0.8 and 1.0) phosphors. | |
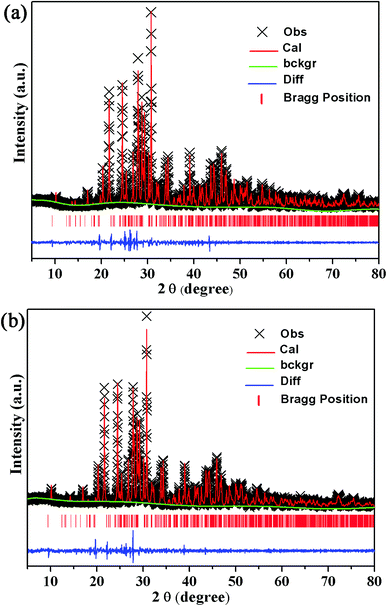 |
| Fig. 2 Rietveld refinement of observed, calculated and difference of the powder XRD patterns of (a) Ba2Y5B5O17 and (b) Ba2Y4.2Eu0.8B5O17. | |
Table 1 Crystal structure data of Ba2Y5B5O17 and Ba2Y4.2Eu0.8B5O17 from the Rietveld refinement
Compound |
Ba2Y5B5O17 |
Ba2Y4.2Eu0.8B5O17 |
Crystal system |
Orthorhombic |
Orthorhombic |
Space group |
Pbcn (no. 60) |
Pbcn (no. 60) |
a (Å) |
17.4486 (8) |
17.45484 (18) |
b (Å) |
6.64715 (29) |
6.66655 (9) |
c (Å) |
13.0128 (6) |
13.06090 (18) |
α = β = γ (°) |
90 |
90 |
V (Å3) |
1509.28 (19) |
1519.813 (34) |
Z |
4 |
4 |
Rp (%) |
2.54 |
1.99 |
Rwp (%) |
3.67 |
2.70 |
Table 2 The atomic coordinations, occupancies and isotropic displacement parameters as determined by Rietveld refinement for Ba2Y4.2Eu0.8B5O17
Atom |
x |
y |
z |
Occ. |
Uiso (Å2) |
Wyck. |
Ba1 |
0.33581 |
0.02396 |
0.18393 |
0.90943 |
−0.00532 |
8d |
Y1 |
0.33581 |
0.02396 |
0.18393 |
0.90943 |
−0.00532 |
8d |
Ba2 |
0.5 |
0.41124 |
0.75 |
0.2 |
0.01275 |
4c |
Eu |
0.5 |
0.41124 |
0.75 |
0.8 |
0.01275 |
4c |
Y3 |
0.18165 |
0.04629 |
0.41451 |
1 |
−0.02461 |
8d |
Y4 |
0.01607 |
0.76051 |
0.47887 |
1 |
0.0088 |
8d |
B1 |
0.15138 |
0.52964 |
0.40624 |
1 |
−0.09 |
8d |
O1 |
0.19532 |
0.38553 |
0.41003 |
1 |
−0.072 |
8d |
O2 |
0.14487 |
0.7102 |
0.40012 |
1 |
0.01301 |
8d |
O3 |
0.08387 |
0.45757 |
0.49893 |
1 |
0.0003 |
8d |
B2 |
0.69329 |
0.58445 |
0.77545 |
1 |
−0.06048 |
8d |
O4 |
0.67945 |
0.4438 |
0.75562 |
1 |
0.0293 |
8d |
O5 |
0.61289 |
0.3519 |
0.8842 |
1 |
−0.00301 |
8d |
O6 |
0.69615 |
0.58683 |
0.91213 |
1 |
−0.03713 |
8d |
O7 |
0.94229 |
0.89496 |
0.5666 |
1 |
−0.0122 |
8d |
O8 |
0.0274 |
0.26356 |
0.67037 |
1 |
−0.055 |
8d |
O9 |
0.5 |
0.94764 |
0.25 |
1 |
−0.04744 |
4c |
B3 |
0.0 |
0.63778 |
0.25 |
1 |
−0.09 |
4c |
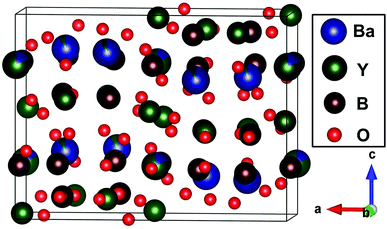 |
| Fig. 3 Crystal structure of Ba2Y5B5O17. | |
As reported previously, the Ba2Y5B5O17 host lattice contains two crystallographically independent, fully occupied Y3+ sites. Specifically, a distorted octahedron and a distorted capped trigonal prism around Y3 and Y4 site. The two Y3+ sites are shared with Ba2+, namely, Ba1/Y1 with a majority Ba (CN = 10) and Y2/Ba2 with a majority Y (CN = 7), respectively. All of the boron atoms are three-coordinated by oxygen, making slightly distorted trigonal planar units.31–33
3.2. Morphology analysis
Fig. 4(a) and (b) depicts the FE-SEM micrographs of the Ba2Y4.2Eu0.8B5O17 phosphors. The as-obtained micrographs exposed that the particles were agglomerated with irregular morphology. The estimated size of the particles could be in micrometer range which is suitable practical application for WLEDs.34 Meanwhile, the elemental mapping results also suggested that the Eu were evenly distributed throughout the entire particles, as indicated in Fig. 4(c). The remaining mapping images of Ba, Y, B, and O was provided in Fig. S1 (ESI†).
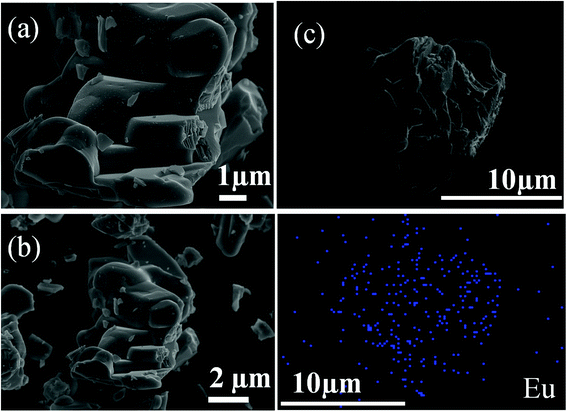 |
| Fig. 4 FE-SEM micrographs (a) and (b). The elemental mapping (c) of europium for the Ba2Y4.2Eu0.8B5O17 phosphors. | |
3.3. Photoluminescence properties
Fig. 5 shows the PLE and PL spectra of the Ba2Y4.2Eu0.8B5O17 phosphors. The excitation spectrum was monitored at 616 nm corresponding to 5D0 → 7F2 transition of Eu3+. The PLE spectrum consisted of two parts namely a broad excitation band in the wavelength range from 230 to 310 nm, and a series of sharp PLE peaks located between 315 to 550 nm. It can be clearly noted that the broad band centered at 277 nm was assigned to the O2− → Eu3+ charge transfer band (CTB), corresponding to the electron transfer from the completely filled 2p orbital of O2− ions to the empty states of 4f orbital of Eu3+ ions. In addition, the several sharp excitation peaks, associated with the typical intra-configurational 4f–4f forbidden transitions of Eu3+ ions were found with peaks positioned at 321 nm (7F0 → 5H6), 364 nm (7F0 → 5D4), 383 nm (7F0 → 5L7), 396 nm (7F0 → 5L6), 418 nm (7F0 → 5D3), 466 nm (7F0 → 5D2) and 537 nm (7F0 → 5D1).9,30,35 Among all the sharp PLE peaks, the strongest excitation peak located at 396 nm matches well with the n-UV LED chip for WLEDs applications. The PL spectrum of Ba2Y4.2Eu0.8B5O17 phosphor (λex = 396 nm) exhibited the well-known emission characteristics of Eu3+ in the spectral region from 500 nm to 750 nm. The sharp emission peaks at 580, 592, 616, 650 and 705 nm can be assigned to the transitions from the excited state 5D0 to ground state 7FJ (J = 0, 1, 2, 3 and 4) levels of Eu3+ ions.30,36 From this spectrum, it can be ascertained that the intensity of electric dipole (ED) 5D0 → 7F2 transition at around 616 nm was much higher than that of the magnetic dipole (MD) 5D0 → 7F1 transition at around 592 nm. When the Eu3+ ion is situated at the crystallographic site with inversion symmetry, the 5D0 → 7F1 (MD) transition is dominant while in a site without inversion symmetry, the 5D0 → 7F2 (ED) transition will be dominant.37,38 The above result suggested that the local symmetry of Eu3+ sites belong to the non-centrosymmetric in Ba2Y5B5O17 host lattice.
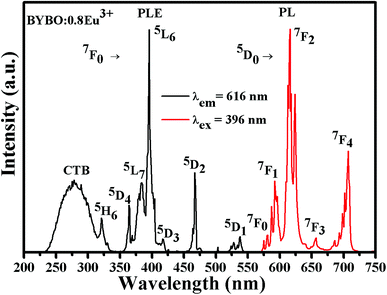 |
| Fig. 5 The PLE (λem = 616 nm) and PL (λex = 396 nm) spectra of Ba2Y4.2Eu0.8B5O17 phosphors. | |
3.4. Concentration quenching and energy transfer of Ba2Y5−xEuxB5O17 phosphors
The PL spectra of Ba2Y5−xEuxB5O17 (0.1 ≤ x ≤ 1.0) phosphors with different Eu3+ concentrations under 396 nm excitation were shown in Fig. 6(a). The shapes and peak positions of the PL spectra remained unchanged with different concentration of Eu3+ ions, except for the PL intensity (see Fig. 6(b)). The inset of Fig. 6(a) illustrates the dependence of PL intensity of various Eu3+ concentrations under λex = 396 nm. It can be seen that the PL intensity enhanced with the amount of Eu3+ until it reached a maximum intensity at x = 0.8, followed by a gradual decrease with a further increase in Eu3+ ion content owing to the concentration quenching effect.13 In general, the concentration quenching phenomenon commonly takes place as a consequence of exchange interaction, radiation re-absorption or multipole–multipole interaction. In addition, the non-radiative energy transfer mechanism among Eu3+ ions is strongly dependent on the critical transfer distance (Rc), which was calculated by using the concentration quenching method. The Rc can be calculated according to the equation proposed by Blasse:39 |
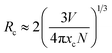 | (1) |
where V represents the volume of the unit cell, xc is the critical concentration of Eu3+ ions, and N is the number of host cations in the unit cell. In the case of Ba2Y5−xEuxB5O17 phosphors, the values of V, xc and N were 1509.28 Å3, 0.8 and 20, respectively,32,33 and thus the Rc was determined to be 5.64 Å. The short-distance exchange interaction becomes ineffective when the critical distance is larger than 5 Å. The radiation re-absorption is unlikely to take place as there is no overlap between PL and PLE spectra in the present case. Therefore, the concentration quenching mechanism of Eu3+ ions in Ba2Y5B5O17 system was dominated by the multipole–multipole interaction. There are three types of electric multipole interactions, including dipole–dipole (d–d), dipole–quadrupole (d–q) or quadrupole–quadrupole (q–q). Based on Dexter's theory,40 the emission intensity (I) per activator ion can be determined by the following equation: |
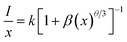 | (2) |
where I/x represents the emission intensity per activator concentration, x stands for the Eu3+ ions concentration; k and β are constants for the given host under the same excitation conditions; θ is a function of multipolar interaction, and θ = 6, 8 and 10 corresponds to d–d, d–q or q–q interactions, respectively.41 The above mentioned equation can be rearranged further by assuming β(x) ≫ 1:42 |
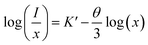 | (3) |
where K′ = log
k − log
β. The dependence of log(I/x) on log(x), as shown in Fig. 7, which was found to be linear and the slope was determined to be −1.91369. Therefore, the value of θ can be calculated as ∼5.74, which approximately equals to 6, indicating that the non-radiative energy transfer mechanism among the Eu3+ ions can be ascribed to d–d interaction in Ba2Y5−xEuxB5O17 phosphors.
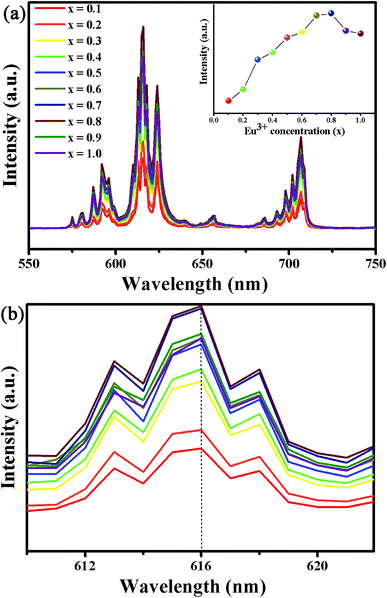 |
| Fig. 6 (a) The PL spectra of Ba2Y5−xEuxB5O17 (0.1 ≤ x ≤ 1.0) phosphors under 396 nm excitation. The inset shows the corresponding PL intensity as a function of Eu3+ concentrations (x). (b) The magnified PL spectra for Ba2Y5−xEuxB5O17 (0.1 ≤ x ≤ 1.0) phosphors. | |
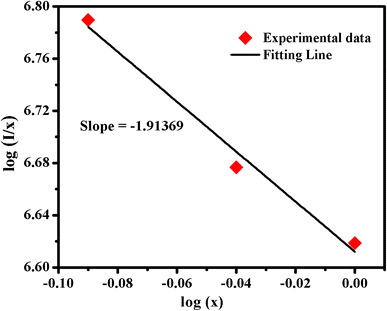 |
| Fig. 7 Dependence of log(I/x) on log(x) for Ba2Y5−xEuxB5O17 phosphors (λex = 396 nm). | |
Generally, the ED transition 5D0 → 7F2 is a hypersensitive transition as this type of transition is very sensitive to the local environment, while the MD transition 5D0 → 7F1 is insensitive to the local environment at Eu3+ site.38,43 Meanwhile, the ratio of integrated emission intensity (asymmetry ratio (R)) of ED (5D0 → 7F2) to MD (5D0 → 7F1) transitions can be used to evaluate the site symmetry around the Eu3+ ions. In general, the high value of R > 1 indicates that the local symmetry around Eu3+ ion is non-centrosymmetric.44 Fig. 8 shows the plot of integrated emission intensity [(5D0 → 7F2)/(5D0 → 7F1)] ratio values on different Eu3+ concentrations. The R values decreased with increasing Eu3+ ion concentration. The calculated R values were found to be 4.36, 4.16, 4.02, 3.95, 3.89, 3.83, 3.80, 3.82, 3.80 and 3.83 for different concentration of (0.1 ≤ x ≤ 1.0) Eu3+ activated phosphors, indicating that Eu3+ occupied the distorted cation sites with non-centrosymmetric.44 Particularly, the calculated R value (3.82) of Ba2Y4.2Eu0.8B5O17 phosphors was found to be practically better than several previous Eu3+-doped red phosphors, as shown in Table 3. The lack of an inversion center the Eu3+ ion is favorable in achieving a bright red phosphor with high color purity.36
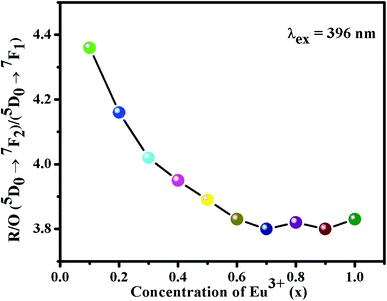 |
| Fig. 8 The asymmetry ratio (R) values with various concentrations of Eu3+ in Ba2Y5−xEuxB5O17 (0.1 ≤ x ≤ 1.0) phosphors. | |
Table 3 Asymmetry ratio (R) of (5D0 → 7F2/5D0 → 7F1) transitions of Eu3+ activated different red phosphors
Red phosphors |
Asymmetry ratio (R) (5D0 → 7F2/5D0 → 7F1) |
Ref. |
Ba2Y4.2Eu0.8B5O17 |
3.82 |
This work |
NaSrB5O9:7%Eu3+ |
2.73 |
5 |
Ba2CaZn2Si6O17:0.9Eu3+ |
2.36 |
6 |
Li6Y(BO3)3:0.5%Eu3+ |
1.71 |
7 |
BaTa2O6:30%Eu3+ |
1.40 |
8 |
Na3Sc2(PO4)3:0.35Eu3+ |
1.37 |
9 |
3.5. PL decay curves of Ba2Y5−xEuxB5O17 phosphors
Fig. 9 illustrates the room-temperature PL decay curves of Ba2Y5−xEuxB5O17 phosphors with different Eu3+ concentrations (0.1 ≤ x ≤ 1.0) under excitation at 396 nm and monitored at 616 nm. All the decay curves can be fitted well based on a first-order exponential decay function as given by the following equation: |
I(t) = I0 + A exp(−t/τ)
| (4) |
where I(t) and I0 are the luminescence intensities at time t and t = 0, respectively; A is a constant, and τ represents the characteristic decay lifetime for the exponential component.9,13 According to eqn (4), the calculated lifetime values of the 5D0 emitting level of Eu3+ ions in Ba2Y5−xEuxB5O17 (0.1 ≤ x ≤ 1.0) phosphors were 1.347, 1.365, 1.388, 1.426, 1.461, 1.484, 1.519, 1.546, 1.580 and 1.593 ms, respectively. Obviously, the PL lifetime values slightly increased with increasing various Eu3+ concentrations, which can be attributed to energy migration between Eu3+ ions.13,45–47
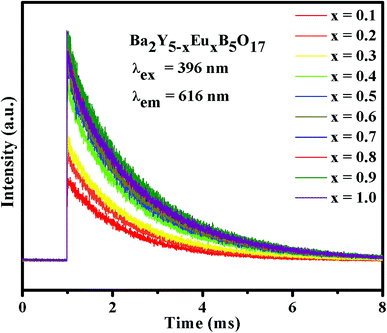 |
| Fig. 9 PL decay curves of Ba2Y5−xEuxB5O17 (0.1 ≤ x ≤ 1.0) phosphors excited at 396 nm and monitored at 616 nm. | |
3.6. The CIE chromaticity coordinates of Ba2Y4.2Eu0.8B5O17
Fig. 10 presents the CIE chromaticity diagram of Ba2Y4.2Eu0.8B5O17 phosphors under excitation at 396 nm. According to the PL spectrum, the CIE coordinates were calculated as (0.653, 0.345), which was much closer to the NTSC standard red light (0.670, 0.330) than that of the commercial red-emitting phosphor of Y2O2S:Eu3+ (0.637, 0.327) and better than other Eu3+ activated phosphors Na3Sc2(PO4)3:0.35Eu3+ (0.642, 0.353) Ca0.9Eu0.1TiO3 (0.630, 0.370) CaLa0.65Eu0.35B7O13 (0.611, 0.388).9,48,49 Furthermore, to better understand the red emission of the Eu3+-activated Ba2Y4.2Eu0.8B5O17 phosphors, the color purity was calculated according to the following expression:9,50 |
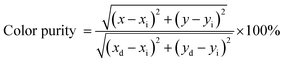 | (5) |
where (x, y), (xi, yi) and (xd, yd) refers the color coordinates of emission light, the equal energy point and color coordinates of the dominant wavelength points, respectively. In the present work, (x = 0.653, y = 0.345), (xi = 0.310, yi = 0.316,) and (xd = 0.675, yd = 0.324). According to eqn (5), the color purity of Ba2Y4.2Eu0.8B5O17 red phosphors was determined to be around 94.3%. We can find that the Ba2Y4.2Eu0.8B5O17 phosphor showed strong red emission intensity with good CIE chromaticity coordinates and eminent color purity. In addition, the IQE is an essential parameter for practical application. The IQE of the optimized phosphor was measured using an integrating sphere. The IQE value can be calculated according to the equation:51,52 |
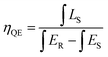 | (6) |
where LS is the luminescence emission spectrum of the sample, ES is the spectrum of the light used for exciting the sample, and ER is the spectrum of the excitation light without the sample in the sphere, respectively (see Fig. 11). Under the excitation of 396 nm, the IQE of Ba2Y4.2Eu0.8B5O17 red-emitting phosphor was determined to be 47.2%, which was higher than the commercial Y2O2S:Eu3+ phosphors (IQE: 35%).4,9 These results suggest that the Ba2Y5−xEuxB5O17 phosphor could be a promising red phosphor for n-UV-based WLEDs.
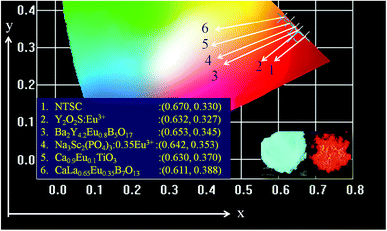 |
| Fig. 10 CIE color coordinates of the NTSC, commercial Y2O2S:Eu3+, Ba2Y4.2Eu0.8B5O17, Na3Sc2(PO4)3:0.35Eu3+, Ca0.9Eu0.1TiO3 and CaLa0.65Eu0.35B7O13 red phosphors. The inset shows the digital photographs of Ba2Y4.2Eu0.8B5O17 phosphors at daylight (left) and under the 365 nm UV lamp (right). | |
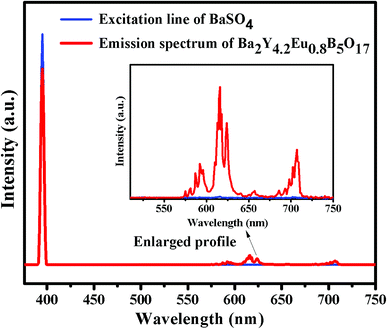 |
| Fig. 11 Excitation line of BaSO4 and emission spectrum of the Ba2Y4.2Eu0.8B5O17 phosphors recorded by a spectrofluorometer equipped with an integrating sphere. The inset shows the magnified emission spectrum in the wavelength range 500–750 nm. | |
3.7. Thermal stability of the Eu3+-activated Ba2Y4.2Eu0.8B5O17 phosphors
The thermal stability of phosphor is one of the essential parameter to find potential application in the high-power WLEDs.13 Fig. 12(a) shows the PL spectra of Ba2Y4.2Eu0.8B5O17 phosphors recorded at various temperatures ranging from 303–483 K under excitation at 396 nm. The spectra clearly indicated that the PL intensity decreased with increasing temperature owing to thermal quenching effect. Generally, the PL intensity of phosphors at 423 K with respect to that at room temperature (303 K) is utilized to assess the thermal stability.9 It is evident that the PL intensity decreased to 68.7% at 423 K in comparison with that of its initial PL intensity at room temperature (303 K), as shown in Fig. 12(b). To better understand the temperature effect on the PL intensity of the phosphors, the activation energy was calculated according to the Arrhenius equation, which can be illustrated below:9 |
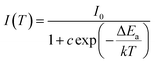 | (7) |
where I0 and I(T) stands for the initial PL intensity and the PL intensity at different given temperature T, ΔEa represents the activation energy for thermal quenching, c is a constant for the host, and k is the Boltzmann constant (8.629 × 10−5 eV K−1). The value of ΔEa can be estimated through the eqn (8) which is modified by the eqn (7) as follows:53 |
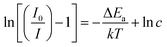 | (8) |
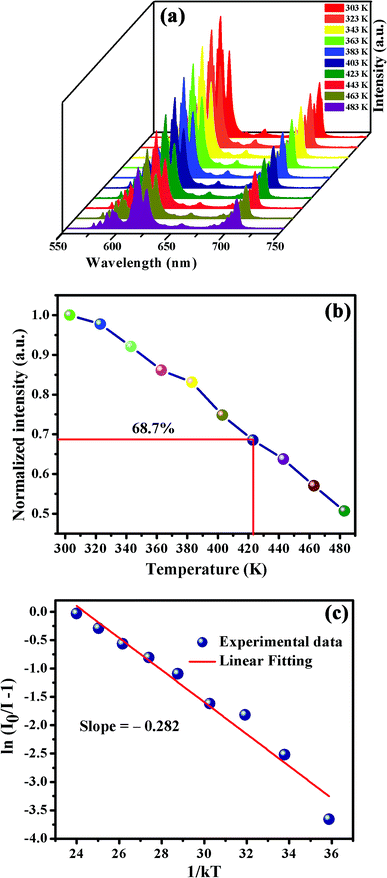 |
| Fig. 12 (a) Temperature dependent PL spectra of Ba2Y4.2Eu0.8B5O17 phosphor (λex = 396 nm). (b) Normalized PL intensity of Ba2Y4.2Eu0.8B5O17 phosphors as a function of different temperatures. (c) The relationship of ln(I0/I − 1) versus 1/kT of the Ba2Y4.2Eu0.8B5O17 phosphors. | |
The plot of ln[(I0/I) − 1] versus 1/kT yielded a straight line, as illustrated in Fig. 12(c). The slope of the fitting line is around −0.282 and thus the activation energy ΔEa was determined to be 0.282 eV for Ba2Y4.2Eu0.8B5O17 phosphors, which was higher than the other Eu3+ activated Na3Sc2(PO4)3, Ca3La(GaO)3(BO3)4 and BaZrGe3O9:Eu3+ red phosphors (ΔEa: 0.23,0.25 and 0.175 eV).9,37,54 This high activation energy suggested that the obtained phosphors have good thermal stability and thus they are suitable for applying in WLEDs.
4. Conclusion
In summary, Eu3+-activated Ba2Y5−xEuxB5O17 (0.1 ≤ x ≤ 1.0) red emitting phosphors have been synthesized by a high-temperature solid-state reaction. Under excitation at 396 nm, the Ba2Y5−xEuxB5O17 phosphors exhibited intense red emission at 616 nm due to the electric dipole transition 5D0 → 7F2 of Eu3+. The optimum concentration of Eu3+ in the Ba2Y5−xEuxB5O17 phosphor was determined to be x = 0.8. The corresponding concentration quenching mechanism was evidenced to be d–d interaction. Meanwhile, the Ba2Y4.2Eu0.8B5O17 red phosphor also exhibited impressive thermal stability, which showed about 68.7% at 423 K of its initial emission intensity at room temperature 303 K. As a result, the optimal Ba2Y4.2Eu0.8B5O17 phosphor presented significant red emission intensity, excellent color purity with color coordinates (0.653, 0.345) and the IQE of 47.2%. These results imply that the Ba2Y4.2Eu0.8B5O17 phosphors can serve as potential red emitting phosphors for n-UV pumped WLEDs.
Conflicts of interest
There are no conflicts to declare.
Acknowledgements
This work was supported by the National Natural Science Foundation of China (No. 51502190, 61674074, 51402148 and 61405089), National Key Research and Development Program of China administrated by the Ministry of Science and Technology of China (No. 2016YFB0401702), Shenzhen Peacock Team Project (No. KQTD2016030111203005), Shenzhen Innovation Project (No. JCYJ20160301113356947, JCYJ20160301113537474, JCYJ20150630145302223), Distinguished Young Scholar of Natural Science Foundation of Guangdong (No. 2017B030306010), Foshan Innovation Project (No. 2014IT100072), the start-up fund from Southern University of Science and Technology, and the Open Fund of the State Key Laboratory of Luminescent Materials and Devices (South China University of Technology, No. 2017-skllmd-01).
References
- X. Wang, Z. Zhao, Q. Wu, Y. Li and Y. Wang, Inorg. Chem., 2016, 55, 11072–11077 CrossRef PubMed.
- M. Janulevicius, P. Marmokas, M. Misevicius, J. Grigorjevaite, L. Mikoliunaite, S. Sakirzanovas and A. Katelnikovas, Sci. Rep., 2016, 6, 26098 CrossRef PubMed.
- Z. Xia, C. Ma, M. S. Molokeev, Q. Liu, K. Rickert and K. R. Poeppelmeier, J. Am. Chem. Soc., 2015, 137, 12494–12497 CrossRef PubMed.
- X. Huang, S. Wang, B. Li, Q. Sun and H. Guo, Opt. Lett., 2018, 43, 1307–1310 CrossRef PubMed.
- G. Dillip, K. Mallikarjuna, S. Dhoble and B. D. P. Raju, J. Phys. Chem. Solids, 2014, 75, 8–14 CrossRef.
- G. Annadurai and S. M. M. Kennedy, J. Lumin., 2016, 169, 690–694 CrossRef.
- M. M. Yawalkar, G. Zade, K. Dabre and S. Dhoble, Luminescence, 2016, 31, 1037–1042 CrossRef PubMed.
- M. İlhan, M. K. Ekmekçi, A. Mergen and C. Yaman, J. Fluoresc., 2016, 26, 1671–1678 CrossRef PubMed.
- H. Guo, X. Huang and Y. Zeng, J. Alloys Compd., 2018, 741, 300–306 CrossRef.
- X. Huang, B. Li and H. Guo, J. Alloys Compd., 2017, 695, 2773–2780 CrossRef.
- X. Zhang, Y. Fu, Z. Zhao, J. Yang, N. Li and M. Zhang, J. Lumin., 2018, 194, 311–315 CrossRef.
- H. Zhu, M. Fang, Z. Huang, K. Chen, X. Min, Y. Mao and M. Wang, J. Lumin., 2016, 172, 180–184 CrossRef.
- Y. Zhang, W. Gong and G. Ning, New J. Chem., 2016, 40, 10136–10143 RSC.
- Q. Liu, Z. Zheng, X. Zhang and Z. Bai, J. Alloys Compd., 2015, 628, 298–302 CrossRef.
- V. Bachmann, C. Ronda, O. Oeckler, W. Schnick and A. Meijerink, Chem. Mater., 2008, 21, 316–325 CrossRef.
- M. A. Lim, J. K. Park, C. H. Kim, H. D. Park and M. W. Han, J. Mater. Sci. Lett., 2003, 22, 1351–1353 CrossRef.
- S. Zhang, Y. Hu, L. Chen, X. Wang, G. Ju and Y. Fan, J. Mater. Res., 2013, 28, 3130–3136 CrossRef.
- S. Kasturi and V. Sivakumar, Mater. Chem. Front., 2017, 1, 550–561 RSC.
- Y. Zhang, X. Li, K. Li, H. Lian, M. Shang and J. Lin, ACS Appl. Mater. Interfaces, 2015, 7, 2715–2725 CrossRef PubMed.
- A. Kruopyte, R. Giraitis, R. Juskenas, D. Enseling, T. Jüstel and A. Katelnikovas, J. Lumin., 2017, 192, 520–526 CrossRef.
- X. Huang, Nat. Photonics, 2014, 8, 748 CrossRef.
- P. Du, X. Huang and J. S. Yu, Chem. Eng. J., 2018, 337, 91–100 CrossRef.
- H. Deng, Z. Gao, N. Xue, J. H. Jeong and R. Yu, J. Lumin., 2017, 192, 684–689 CrossRef.
- Z. Gao, N. Xue, J. H. Jeong and R. Yu, Mater. Res. Bull., 2017, 95, 497–502 CrossRef.
- Y. Zhang, J. Xu, Q. Cui and B. Yang, Sci. Rep., 2017, 7, 42464 CrossRef PubMed.
- D. V. Deyneko, V. A. Morozov, J. Hadermann, A. E. Savon, D. A. Spassky, S. Y. Stefanovich, A. A. Belik and B. I. Lazoryak, J. Alloys Compd., 2015, 647, 965–972 CrossRef.
- R. Cao, T. Fu, Y. Cao, H. Ao, S. Guo and G. Zheng, Mater. Lett., 2015, 155, 68–70 CrossRef.
- W. Xiao, X. Zhang, Z. Hao, G.-H. Pan, Y. Luo, L. Zhang and J. Zhang, Inorg. Chem., 2015, 54, 3189–3195 CrossRef PubMed.
- P. Chen, F. Mo, A. Guan, R. Wang, G. Wang, S. Xia and L. Zhou, Appl. Radiat. Isot., 2016, 108, 148–153 CrossRef PubMed.
- R. Guo, S. Tang, B. Cheng and D. Tan, J. Lumin., 2013, 138, 170–173 CrossRef.
- M. Hermus, P.-C. Phan and J. Brgoch, Chem. Mater., 2016, 28, 1121–1127 CrossRef.
- Y. Xiao, Z. Hao, L. Zhang, W. Xiao, D. Wu, X. Zhang, G.-H. Pan, Y. Luo and J. Zhang, Inorg. Chem., 2017, 56, 4538–4544 CrossRef PubMed.
- X. Zhang, J. Zhang and Y. Chen, Dyes Pigm., 2018, 149, 696–706 CrossRef.
- B. Han, J. Zhang, Z. Wang, Y. Liu and H. Shi, J. Lumin., 2014, 149, 150–154 CrossRef.
- H.-Y. Chen, H.-L. Lai, R.-Y. Yang and S.-J. Chang, J. Mater. Sci.: Mater. Electron., 2016, 27, 2963–2967 CrossRef.
- W. Dai, M. Zhou, Z. Xian and L. Zeng, RSC Adv., 2014, 4, 25470–25478 RSC.
- Q. Zhang, X. Wang, X. Ding and Y. Wang, Inorg. Chem., 2017, 56, 6990–6998 CrossRef PubMed.
- X. Huang, B. Li, H. Guo and D. Chen, Dyes Pigm., 2017, 143, 86–94 CrossRef.
- G. Blasse, J. Solid State Chem., 1986, 62, 207–211 CrossRef.
- D. L. Dexter, J. Chem. Phys., 1953, 21, 836–850 CrossRef.
- L. Van Uitert, J. Electrochem. Soc., 1967, 114, 1048–1053 CrossRef.
- D. Dexter and J. H. Schulman, J. Chem. Phys., 1954, 22, 1063–1070 CrossRef.
- P. Du and J. S. Yu, Dyes Pigm., 2017, 147, 16–23 CrossRef.
- T. L. Francis, P. P. Rao, M. Thomas, S. Mahesh and V. Reshmi, J. Mater. Sci.: Mater. Electron., 2014, 25, 2387–2393 CrossRef.
- A. Fu, A. Guan, F. Gao, X. Zhang, L. Zhou, Y. Meng and H. Pan, Opt. Laser Technol., 2017, 96, 43–49 CrossRef.
- J. Grigorjevaite and A. Katelnikovas, ACS Appl. Mater. Interfaces, 2016, 8, 31772–31782 CrossRef PubMed.
- L. Mengting and J. Baoxiang, J. Rare Earths, 2015, 33, 231–238 CrossRef.
- D. K. Singh and J. Manam, J. Mater. Sci.: Mater. Electron., 2016, 27, 10371–10381 CrossRef.
- F. Xiong, J. Luo, H. Lin, X. Meng, Y. Wang, H. Shen and W. Zhu, Optik, 2018, 156, 31–38 CrossRef.
- P. Du, Y. Guo, S. H. Lee and J. S. Yu, RSC Adv., 2017, 7, 3170–3178 RSC.
- J. Zhong, D. Chen, Y. Zhou, Z. Wan, M. Ding, W. Bai and Z. Ji, Dalton Trans., 2016, 45, 4762–4770 RSC.
- T. Wei, Q. Ren, X. Wu, X. Shi, B. Wang and Z. Huo, Opt. Laser Technol., 2016, 85, 7–13 CrossRef.
- X. Huang, B. Li and H. Guo, Ceram. Int., 2017, 43, 10566–10571 CrossRef.
- J. Liang, P. Du, H. Guo, L. Sun, B. Li and X. Huang, Dyes Pigm., 2018, 157, 40–46 CrossRef.
Footnote |
† Electronic supplementary information (ESI) available. See DOI: 10.1039/c8ra03059f |
|
This journal is © The Royal Society of Chemistry 2018 |
Click here to see how this site uses Cookies. View our privacy policy here.