DOI:
10.1039/C7RA08915E
(Paper)
RSC Adv., 2017,
7, 51426-51435
Surface adsorption and thermodynamic properties of mixed system of ionic liquid surfactants with cetyltrimethyl ammonium bromide†
Received
12th August 2017
, Accepted 17th October 2017
First published on 6th November 2017
Abstract
The effects of temperature and concentration of CTAB added into imidazolium-based ionic liquids (CnmimBr, n = 10, 12, 16) solution were investigated, and surface active parameters and aggregation of these surfactants were explored by surface tension and conductivity measurements, which include critical micelle concentration (CMC), maximum surface excess concentration (Γmax), minimum surface area per surfactant molecule (Amin), surface tension at the CMC (γCMC), adsorption efficiency (pC20), and surface pressure (ΠCMC), as well as thermodynamic parameters of micellization. The results were reported in the presence of CTAB at 0, 4, 8, 12, and 16 mM for C10mimBr, 0, 0.2, 0.4, 0.8, and 1.2 mM for C12mimBr, and 0, 0.04, 0.08, 0.12, and 0.16 mM for C16mimBr in mixed systems. The increase of the CMCs with temperature were observed, while pC20, Γmax, and standard entropy of aggregation were decreased, indicating that the aggregation is an enthalpy-driven process within the temperature limits of the present study. FT-IR and 1H NMR measurements revealed that the changes mentioned above were controlled by the electrostatic effect and hydrophobic interactions between CTAB and ILs molecules.
1 Introduction
Ionic liquids (ILs) have gained great attention for their promising role as alternative media in catalysis, separation, and electrochemical processes owing to their unique chemical and physical properties, such as negligible vapor pressure, wide liquidous range, excellent solvation, high thermal stability, ionic conductivity and wide electrochemical windows. Especially, as an environmentally friendly solvent, also called a “green solvent”, ILs make some contribution towards preventing environmental pollution by virtue of their non-volatility. Based on their inherent amphiphilic structure consisting of a non-polar hydrophobic tail and a polar cationic head group, a typical long-chain ILs could possess surface active properties similar to those of conventional cationic surfactants, so they have been extensively studied in the field of colloid and interface science in recent years. For instance, the surface tension and critical micelle concentration (CMC) of long-chain ILs decrease with alkyl chain length. ILs applications in wide fields are closely dependent on such self-assembly behavior.1 In addition, ILs with different cations and anions have been reported to understand the effect of the ions on aggregation behavior.2–4 However, it is unclear how the surface active and micellization features depend upon their molecular characteristics, concentration, temperature and additives etc. In view of this, study of the interaction and microstructural characteristics of micellar systems is always an important subject area in the discipline of colloid and interface science.
Numerous pioneering studies on multicomponent mixtures of ionic liquid have widened the application field of ILs. For instance, phase behaviour of aqueous two-phase systems containing an ionic liquid,5 molecular interactions and preparation of nanoparticles,6,7 and effects on properties of nanocomposites.8 In these studies, the binary mixture is relatively fascinating from an academic point of view wherein including common ionic–ionic systems,9–11 and ionic–nonionic systems.12–14 The CTAB/bmim-octylSO4 binary system in aqueous solution at two total concentrations and different CTAB mole fractions was investigated and found to form the CTA-octylSO4 catanionic complex,9 which is considered analogue to the effect of the addition of inorganic salts in such similar systems.15 The aggregation behaviour of the alkyl triphenylphosphonium bromide (CnTPPBr, n = 10, 12, 14, 16)/Triton X-100 system was studied by Thakkar et al.13 Furthermore, a generalized phase separation model was discussed in ionic–nonionic mixtures.16 The ionic liquid TEA(BF4) and non-ionic surfactant TX-100/Brij-56/Brij-58/Tween-20/Tween-80 in aqueous media at different temperatures were studied, respectively.17 Some other reports have just been published regarding the IL surfactant applications.18,19 Different measurement technologies were used to obtain the needed surface activities and thermodynamics functions, such as a small-angle neutron scattering (SANS),13,20 dynamic light scattering (DLS) and viscosity measurements,17 conductivity,12,21,22 surface tension measurements,23 as well different analytical methods, FT-IR,3 UV-visible spectrum,22,24 fluorescence spectroscopy,4,22,25,26 isothermal titration calorimeter25 and NMR technology.27,28
Apart from the self-assemblies and applications of ILs surfactants in various fields, the aggregation behavior of 1-alkyl-3-methylimidazolium (Cnmim+) in water has been presented by many research groups.13,15,29 The micellization behavior of the DTAB/bdmimCl system has been reported by Pal' group,27 also including their related work on tetradecyltrimethylammonium bromide TTAB/C5mim/PF6 system,30 CTAB/bdmimBr system,31 odmimCl/DTAB mixed system,21 and TTAB/bdmimBr mixture.28 However, in all these systems, all studied ILs as an additive were added into traditional surfactant solution to form IL-surfactant mixtures. The CMC values of the mixtures increase with increasing wt% IL content and temperature at the same IL concentration. So it would be interesting to observe the effect of CTAB as an additive on the micellization of IL as no reports have been found to date. Consequently the purpose of the present work is to study the effects of temperature and CTAB addition into ILs (CnmimBr, n = 10, 12, 16) solution on the surface properties of the mixture system and the thermodynamic properties of micellization. This prompted us to carry out systematic investigations with a focus mainly on examining the surface activity and aggregate formation of ionic liquids based on these long-chain ILs and to assess the effect of chain length and head group nature on the CMC, pC20, Γmax, Amin, and thermodynamic parameters of aggregation by surface tension and electrical conductance measurements for mixed systems. Conductivity and surface tension measurements were used to obtain the CMC values for three IL/CTAB solutions, and the thermodynamic parameters were used to analyze the driving force of micellization. In addition, 1H NMR and FT-IR measurements were done to understand the interactions between the IL and the surfactant molecules. The results obtained could be compared with similar systems for further applications of binary systems in some areas of separation, adsorption, and other surface research fields.
2 Materials and methods
2.1 Materials
Cetyltrimethyl ammonium bromide (CTAB, MW: 364.45, purity ≥ 99%, CAS number: 57-09-0) was obtained from Sinopharm Chemical Reagent Co., Ltd (Shanghai, China). 1-Hexadecyl-3-methylimidazolium bromide (C16mimBr, MW: 387.44, purity ≥ 99.9%, CAS: 132361-22-9), 1-dodecyl-3-methylimidazolium bromide (C12mimBr, MW: 331.33, purity ≥ 99.9%, CAS: 61546-00-7), and 1-decyl-3-methylimidazolium bromide (C10mimBr, MW: 303.28, purity ≥ 99.9%, CAS: 188589-32-4) were purchased from Shanghai Cheng Jie Chemical Co., Ltd., China, and these ILs were dried at 343 K for 24 h in vacuum drying oven (DZF-6050, Shanghai Shupei Laboratory Equipment Co., Ltd., China) prior to use. The molecular structures of the ILs and CTAB are shown in Fig. 1. D2O (D > 99.96%) was obtained from Sigma-Aldrich and used as the solvent in 1H NMR measurements.
 |
| Fig. 1 Molecular structures of ILs and CTAB. | |
2.2 Methods
2.2.1 Conductivity measurement. The electrical conductivities were measured at different temperatures using a digital conductivity meter (DDSJ-308A, Shanghai Leici Instrument Factory, China) equipped with a platinized platinum electrode. A stable temperature was maintained with a super-thermostatic water bath (ZC-18Q, Tianheng Instrument Factory, Ningbo, China). Before the measurements, the conductivity cell constant was calibrated with a known specific conductance aqueous solution of 0.01 M potassium chloride aqueous solutions. At least three measurements were made every time and the specific conductivity was calculated from the mean value. The conductance due to water ionization (5.83 μs cm−1, pH = 6.98) was subtracted from the measured data before data analysis. The uncertainty in conductivity measurements was less than ±0.4%. The cell assembly was dipped in glass vials kept in a water bath thermostatted at the studied temperature maintained to ±0.1 K.
2.2.2 Surface tension measurement. The surface tension for aqueous solutions of single surfactants and binary mixtures were assessed using the maximum bubble pressure method with a tensiometer (DP-AW-1, Nanjing SangLi Electronic Equipment Factory, China) under atmospheric pressure. The calibration was performed by measuring the surface tension of doubly distilled de-ionized water (71.97 mN m−1) before each experiment. The temperature was maintained within ±0.1 K by circulating water. The stock solutions were then diluted with fresh distilled water to desired concentrations. The uncertainty in the measured surface tensions was within ±0.03 mN m−1.The surface excess adsorption, Γmax, and the minimum area per surfactant molecule, Amin, at the air–solvent interface were calculated using surface tension measurement values according to the Gibbs adsorption isotherm equation, given by eqn (1) and (2):
|
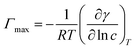 | (1) |
|
 | (2) |
where
R is the gas constant,
T is the absolute temperature,
γ is the surface tension, and
c is the IL concentration of mixed solutions.
L is Avogadro's constant. The adsorption efficiency (
pC20) is an important surface activity parameter, which is defined by
eqn (3):
|
pC20 = −log C20
| (3) |
Surface pressure at the CMC, ΠCMC, is a measure of the efficiency of the surfactant to lower the surface tension of the water, and is obtained by means of eqn (4):
where
C20 is the effective concentration of all surfactants in the mixed system required to reduce the surface tension of pure water by 20 mN m
−1 γ0 is the surface tension of pure water for the respective temperature, and
γCMC is the measured surface tension at the CMC.
The enthalpic contribution to the micellization process, ΔHm, was determined based on ΔGm, using the Gibbs–Helmholtz relation as shown in eqn (5) and (6). The standard entropy of the micellization, ΔSm, was calculated from eqn (7):
|
ΔGm = (2 − α)RT ln XCMC
| (5) |
|
 | (6) |
|
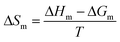 | (7) |
where
XCMC is the CMC in mole fraction, the degree of dissociation,
α, was associated to the degree of ionization, which represents the fraction of amphiphile ions in the aggregate neutralized by counter anions.
R is the gas constant, and
T is temperature. According to
eqn (5), Δ
Gm of micellization at different temperatures may be calculated using
XCMC. Thus, the curve of Δ
Gm/
T against 1/
T can be drawn, and Δ
Hm can be finally calculated from the slopes of tangential lines at various temperatures. The parameters thus obtained are presented in
Table 2.
2.2.3 FT-IR spectra measurement. The spectra of the samples were measured using a Thermo Scientific Nicolet IS10 FT-IR spectrometer equipped with a standard KBr beam splitter and DTGS detector. The mixture spectra of the [CnmimBr]/CTAB solution were scanned on the Smart iTR™ diamond ATR reflection accessory for qualitative analysis in this study. The spectra from samples (pure IL or mixture of IL and TX-100 without water) were collected in 40 seconds over the full mid-infrared range (4000 to 400 cm−1). The samples needed to be premixed (molar ratio 1
:
1) for a period of time before analysis. Instrument control, data collection and analysis were performed using the Thermo Scientific OMNIC software package.
2.2.4 1H NMR measurements. The NMR chemical shifts for various protons were observed with a Bruker AVANCE III HD 400 M NMR spectrometer at room temperature (about 298 K). Deuterium oxide (D2O) as a solvent for all the NMR measurements was used to determine chemical shift δ for the [CnmimBr]/CTAB solution.All the experiments were carried out in doubly distilled de-ionized water.
3 Results and discussion
3.1 Conductivity measurement
3.1.1 CMC determination. Conductivity measurements were carried out in solutions of ionic liquids over a wide concentration range at 298.15–338.5 K to obtain CMC values with the addition of the surfactant CTAB and without CTAB. Sufficient care was taken to avoid high IL concentrations to ensure that the solutions were not highly viscous and were free of air bubbles during the measurements.For pure ionic liquids solutions, the plots of conductivity against concentration of IL surfactant (Fig. 2) displayed the difference of the curves well, namely the slope in the pre-micellar region was higher than one in post-micellar region regardless of the pure IL surfactants, or mixture solutions. The presence of turning point is attributable to the change of aggregation state from CTAB molecules and IL molecules. Thus, the abscissa value corresponding to the turning point is the CMC value of the studied system, as listed in Table 1. Reasonably, the CMC decreased with increasing length of alkyl chain and such a systematic decrease could be due to the enhanced hydrophobic character of higher alkyl chains.2 Therefore, it can be concluded that the interfacial activity of the pure IL surfactants is in the order of C16mimBr > C12mimBr > C10mimBr. It is therefore suggested that the aggregation process of long-chain ILs in water needs to be close to that of the micellization process of classical ionic surfactants in aqueous media. Furthermore, the degree of dissociation (α) for these systems was obtained from the ratio of the slopes of the two linear lines drawn through regions corresponding to above and below the CMC value, as listed in Table 1.
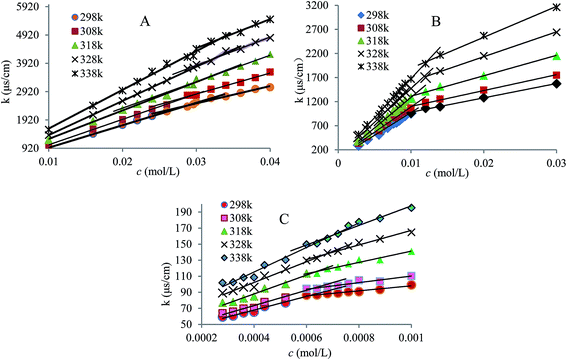 |
| Fig. 2 Conductivity of pure C10mimBr (A), C12mimBr (B) and C16mimBr (C) in aqueous solutions at different temperatures. | |
Table 1 Thermodynamic functions of CnmimBr in the absence of CTAB (conductivity method)a
T K |
C10mimBr |
C12mimBr |
C16mimBr |
CTAB (mM) |
α |
CMC (mM) |
CTAB (mM) |
α |
CMC (mM) |
CTAB (mM) |
α |
CMC (mM) |
Standard uncertainties s are s(CMC) = ±0.03 (mM). The value is from the literature.25 The value is from the literature.4 |
298.15 |
0 |
0.321 |
28.9, 29.3b |
0 |
0.321 |
10.1, 10.9b |
0 |
0.316 |
0.611, 0.55c |
308.15 |
|
0.417 |
29.8 |
|
0.415 |
10.2 |
|
0.406 |
0.626 |
318.15 |
|
0.481 |
31.4 |
|
0.483 |
10.3 |
|
0.478 |
0.637 |
328.15 |
|
0.504 |
32.4 |
|
0.509 |
11.6 |
|
0.499 |
0.673 |
338.15 |
|
0.511 |
33.1 |
|
0.514 |
12.2 |
|
0.507 |
0.703 |
298.15 |
4 |
0.86 |
28.1 |
0.2 |
0.362 |
9.6 |
0.04 |
0.322 |
0.561 |
308.15 |
|
0.825 |
29.4 |
|
0.437 |
10.2 |
|
0.354 |
0.575 |
318.15 |
|
0.872 |
30.8 |
|
0.481 |
10.6 |
|
0.441 |
0.605 |
328.15 |
|
0.859 |
31.4 |
|
0.523 |
10.9 |
|
0.549 |
0.641 |
338.15 |
|
0.881 |
31.7 |
|
0.547 |
11.4 |
|
0.721 |
0.652 |
298.15 |
8 |
0.971 |
26.6 |
0.4 |
0.520 |
9.4 |
0.08 |
0.347 |
0.545 |
308.15 |
|
0.914 |
27.1 |
|
0.568 |
9.9 |
|
0.461 |
0.556 |
318.15 |
|
0.884 |
28.2 |
|
0.617 |
10.4 |
|
0.502 |
0.583 |
328.15 |
|
0.823 |
29.6 |
|
0.624 |
10.8 |
|
0.528 |
0.625 |
338.15 |
|
0.927 |
30.6 |
|
0.657 |
11.2 |
|
0.661 |
0.641 |
298.15 |
12 |
0.823 |
25.7 |
0.8 |
0.605 |
9.3 |
0.12 |
0.376 |
0.534 |
308.15 |
|
0.865 |
26.4 |
|
0.642 |
9.8 |
|
0.524 |
0.544 |
318.15 |
|
0.906 |
27.3 |
|
0.680 |
10.1 |
|
0.418 |
0.558 |
328.15 |
|
0.964 |
28.5 |
|
0.715 |
10.5 |
|
0.498 |
0.574 |
338.15 |
|
0.819 |
30.3 |
|
0.744 |
10.8 |
|
0.601 |
0.601 |
298.15 |
16 |
0.747 |
24.2 |
1.2 |
0.662 |
8.7 |
0.16 |
0.407 |
0.529 |
308.15 |
|
0.796 |
25.1 |
|
0.689 |
9.1 |
|
0.351 |
0.533 |
318.15 |
|
0.828 |
25.3 |
|
0.699 |
9.3 |
|
0.443 |
0.55 |
328.15 |
|
0.827 |
25.4 |
|
0.716 |
10.3 |
|
0.495 |
0.568 |
338.15 |
|
0.869 |
25.8 |
|
0.735 |
10.7 |
|
0.587 |
0.587 |
Considering the CMC of CTAB is about 0.90 mM,9,27 the concentrations values of CTAB that lie on both sides of the CMC of CTAB were selected to investigate the effect of adding CTAB monomer or micelles to IL aqueous solution on the CMC values and surface activities of mixed systems. The representative plots of the conductance of the mixed system (C12mimBr/CTAB system) versus IL concentration on addition of different concentrations of CTAB in aqueous medium are shown in Fig. 3, while conductivity measurement results for C10mimBr/CTAB and C16mimBr/CTAB are depicted in Fig. S1 and S2 (ESI†) and the measured data are given in Table 1. A similar change to that observed in Fig. 3 can be found and the CMCs of the mixed systems can be calculated.
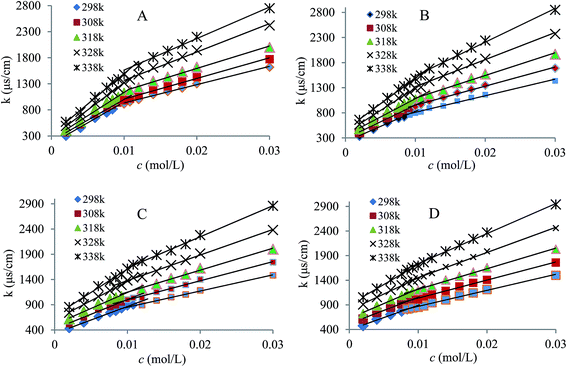 |
| Fig. 3 Conductivity of the mixed system of C12mimBr/CTAB at different temperatures. cCTAB (A–D): 0.2, 0.4, 0.8 and 1.2 mM. | |
According to the data from Fig. 3, S1 and S2,† the CMCs, the degree of dissociation (α) and the thermodynamic parameters of aggregation for ILs/CTAB in water were calculated at five different temperatures of 298.15, 308.15, 318.15, 328.15 and 338.15 K. The corresponding data are listed in Table 1. The data from Table 1 show that the CMCs value of the CnmimBr aqueous systems decreased with the increase in the alkyl chain length and increased with increasing temperature,27,32 which is consistent with the description in the literature33 and the results for other IL solutions.25 This can be interpreted as elevated temperature causes the disruption of the water structure leading to low solubility of the surfactant. Besides, the elevated temperature causes difficulties in forming the micelles, mainly due to the hydration of the ion being greatly enhanced, which significantly weakens the electrostatic interaction of micellization.
In addition, the CMCs of the CnmimBr/CTAB aqueous systems decline with increasing CTAB concentration at the same temperature. Although the positive charges on the head group of CTAB and the imidazolium ring of the IL repel each other, in fact, electrostatic attraction effect between the counter-ion of the IL, Br−, and the head group of the CTAB molecules may reinforce micelle formation to make the CMC value low or the surface activity high. At the same time, the hydrophobic interaction between the tail of the CTAB and the alkyl chain of the IL cation promote the procession of micellization. This finding differs from the CTAB/bmim-octylSO4 system reported by other authors,9 who thought the formation of the pseudo-double chain catanionic surfactant (CTA-octylSO4) led to a much lower critical aggregation concentration (CAC) and surface tension value at the CAC for the mixtures than for the individual components, whereas similar results in CTAB/SDS system have been obtained showing that the electrostatic repulsion of the head groups decreases and consequently the CMC is reduced when SDS gets into the solution.34 This behavior is also similar to the effect of electrolytes such as sodium chloride (NaCl) when added to surfactant solutions. Liu et al. found that the CMC values of C12mimBr aqueous solution decreased with increasing NaBr concentration, and illustrated that the phenomenon arises from the reduction of electrostatic repulsion between the surfactant head groups caused by the additional ions in the solution.15 Additionally, the degree of dissociation (α) decreased with the increase in the alkyl chain length. The small value of α in general indicates that the aggregate structures are compact for CnmimBr/CTAB aqueous systems in water.
3.1.2 Thermodynamic functions. The temperature dependence of the CMC has been employed to compute the thermodynamic parameters of micellization in order to obtain better knowledge about the behavior of the surfactant and intermolecular interactions present in such systems.35 The thermodynamic parameters of the CnmimBr/CTAB mixed system at different CTAB concentrations are shown in Fig. 4. The standard free energy change of the micelle formation (ΔGm) revealed a rather slight decrease with the increment of temperature in mixed systems. There is no doubt that the negative values of ΔGm indicated the spontaneous micellization. The more negative value of ΔGm with the increase in the length of the alkyl chain is attributed to the fact that the longer alkyl chains facilitate the spontaneous aggregate formation. Furthermore, the negative ΔHm implies that micellization formation remains mainly an exothermic process, which is owing to greater dissociation of counter-ions from the micellar surface leading to a more exothermic micellization process. However, ΔHm firstly increased and then declined with temperature limits of 298.15–338.15 K with CTAB concentrations of 0.2, 0.4, 0.8 and 1.2 mM, as can be seen from Fig. 4.
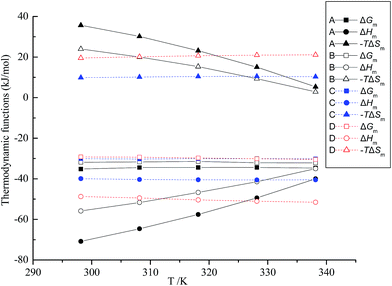 |
| Fig. 4 Thermodynamic functions (kJ mol−1) of C12mimBr/CTAB system against temperature. cCTAB (A–D): 0.2, 0.4, 0.8 and 1.2 mM. | |
Meanwhile, entropy change (−TΔSm) was plotted against temperature to better understand the contribution of entropy to the free energy (Fig. 4). It is noteworthy that the trend in the change of −TΔSm with temperature in the mixed solution is analogous to ΔHm. It can be seen from Fig. 4A–D that −TΔSm (ΔSm) gradually decreases (increases) with temperature at the same low CTAB concentration of 0.2 and 0.4 mM, then substantially keeps flat at 0.8 mM, and finally increases when reaching 1.2 mM. Obviously, this change may be dependent on the presence of the CTAB molecules in Fig. 4A–D with the same IL concentration.17 For CTAB monomer (cCTAB < 0.9 mM) added into IL solution, the disruption of the water structure by the interaction between IL molecules and CTAB monomer makes the arrangement of the mixture system less and less ordered. However, ΔSm decreases with temperature when CTAB micelles (cCTAB > 0.9 mM) are added into IL solution. The essence of this phenomenon is that the higher temperature makes it more difficult to dissolve nonpolar substances in water, leading to enhancement of hydrophobic interactions between micelle molecules. In other words, hydrophobic alkyl chains come closer to each other in the micellar phase. As a result, the molecular arrangement is more ordered so that entropy is reduced. According to the data in Table 1, the CMCs of the two surfactant mixtures at elevated temperatures were lower than each surfactant, which also gives evidence of the strong synergism produced in mixed aggregates.34 In general, Fig. 4 shows that the contribution of enthalpy term ΔHm to ΔGm is superior to (−TΔSm) at all studied temperatures. Therefore, it can also be concluded that the micelle formation of the CTAB/CnmimBr mixture is an enthalpy-driven process. The critical micelle concentration (CMC) determined from conductivity data was also found in the study of the CTAB/DTAB-glycyl dipeptide system,36 which revealed a decrease in the CMC values for both the surfactants with [glycyl dipeptide] and demonstrated the entropy–enthalpy contribution to the micellization process.
3.2 Surface tension measurement
3.2.1 CMC determination. Surface tension measurements were performed at different temperatures. Based on the plots of surface tension versus ILs concentration depicted in Fig. 5–7, several different parameters related to the surface activities are listed in Table 2 wherein the CMC value of the pure ILs are from the previous work.37 It was obvious that the surface tensions of the mixed systems in aqueous media exhibited a steep decrease with an increase of IL concentration up to a certain concentration and subsequently remained constant. The CMC value was obtained by the intersection between the rapidly decreasing line and the flat line of surface tension. As shown in Fig. 5–7, in the case of CTAB at 14 mM or 30 mM in the C10mimBr/CTAB system, a dramatic decrease of the surface tension was observed compared with a moderate decrease at 2 mM or 8 mM CTAB in the C12mimBr/CTAB system, and 0.02 mM or 0.12 mM in the C16mimBr/CTAB system. These trends in the CMC value associated to temperature and CTAB concentration endorse the results drawn from the conductivity method. Chauhan et al. applied surface tension to investigate CM(A)C of a ternary (water + lactose + NaC/NaDC) system.26 The decrease of the CM(A)C values of bile salts in aqueous lactose solution was found to be in excellent agreement with the mixture systems in this work. Moreover, they employed conductivity and UV-vis probe to examine the interactions of the ionic liquid tetrabutylammonium dodecylsulfate (TBADS) in aqueous solutions of glycine with sodium dodecylsulfate (SDS).22
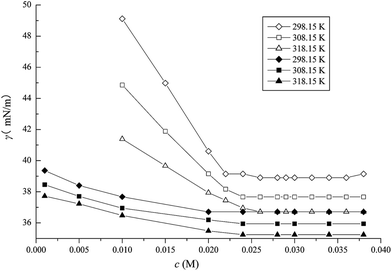 |
| Fig. 5 Surface tension of C10mimBr in the presence of CTAB (◇14 mM, 298.15 K), (□14 mM, 308.15 K), (△14 mM, 318.15 K), (◆30 mM, 298.15 K), (■30 mM, 308.15 K) and (▲30 mM, 318.15 K). | |
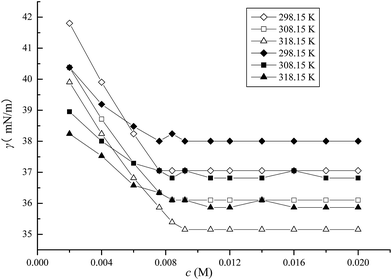 |
| Fig. 6 Surface tension of C12mimBr in the presence of CTAB (◇2 mM, 298.15 K), (□ 2 mM, 308.15 K), (△2 mM, 318.15 K), (◆8 mM, 298.15 K), (■8 mM, 308.15 K) and (▲8 mM, 318.15 K). | |
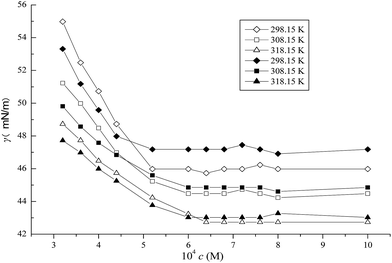 |
| Fig. 7 Surface tension of C16mimBr in the presence of CTAB (◇ 0.02 mM, 298.15 K), (□0.02 mM, 308.15 K), (△0.02 mM, 318.15 K), (◆0.12 mM, 298.15 K), (■0.12 mM, 308.15 K) and (▲ 0.12 mM, 318.15 K). | |
Table 2 Thermodynamic functions of CnmimBr in the absence of CTAB (surface tension method)a
CTAB (mM) |
T K |
CMC (mM) |
ΔGm (kJ mol−1) |
ΔHm (kJ mol−1) |
−TΔSm (kJ mol−1) |
Γmax (μmol m−2) |
Amin (Å2) |
γCMC (mN m−1) |
ΠCMC (mN m−1) |
pC20 |
Standard uncertainties s are s(CMC) = ±0.03 (mM), s(ΔGm) = ±0.03 (kJ mol−1), s(ΔHm) = ±0.02 (kJ mol−1), s(ΔSm) = ±0.02 (J mol−1 K−1). |
C10mimBr |
0 |
298.15 |
27.24 |
−37.44 |
−8.28 |
−29.15 |
1.75 |
94.61 |
48.95 |
23.02 |
1.61 |
308.15 |
30.78 |
−38.42 |
−3.93 |
−34.49 |
1.68 |
98.92 |
45.15 |
26.82 |
1.70 |
318.15 |
31.60 |
−39.53 |
0.15 |
−39.68 |
1.57 |
105.8 |
41.29 |
30.68 |
1.63 |
14 |
298.15 |
22.32 |
−38.77 |
−9.22 |
−29.55 |
105.53 |
38.50 |
33.47 |
38.50 |
105.53 |
308.15 |
24.25 |
−39.65 |
−7.04 |
−32.60 |
113.43 |
37.17 |
33.21 |
38.76 |
113.43 |
318.15 |
25.85 |
−40.59 |
−5.00 |
−35.59 |
121.99 |
36.96 |
31.78 |
40.19 |
121.99 |
30 |
298.15 |
19.89 |
−39.34 |
−18.42 |
−20.92 |
108.31 |
36.02 |
35.95 |
36.02 |
108.31 |
308.15 |
22.55 |
−40.02 |
−11.89 |
−28.13 |
114.96 |
36.00 |
34.38 |
37.59 |
114.96 |
318.15 |
24.38 |
−40.91 |
−5.77 |
−35.13 |
121.99 |
34.68 |
34.06 |
37.91 |
121.99 |
![[thin space (1/6-em)]](https://www.rsc.org/images/entities/char_2009.gif) |
C12mimBr |
0 |
298.15 |
9.19 |
−43.17 |
−35.96 |
−7.21 |
1.80 |
92.49 |
46.71 |
25.26 |
2.35 |
308.15 |
10.23 |
−44.07 |
−31.60 |
−12.46 |
1.78 |
93.49 |
43.52 |
28.45 |
2.29 |
318.15 |
11.06 |
−45.08 |
−27.52 |
−17.56 |
1.70 |
97.59 |
39.36 |
32.61 |
2.26 |
2 |
298.15 |
9.07 |
−43.23 |
−34.71 |
−8.52 |
1.82 |
91.46 |
38.02 |
33.95 |
2.86 |
308.15 |
9.80 |
−44.29 |
−30.36 |
−13.93 |
1.80 |
92.47 |
37.04 |
34.93 |
2.86 |
318.15 |
10.31 |
−45.45 |
−26.28 |
−19.18 |
1.68 |
98.69 |
36.72 |
35.25 |
2.83 |
8 |
298.15 |
8.90 |
−43.33 |
−32.39 |
−10.94 |
1.86 |
89.47 |
37.41 |
34.56 |
2.92 |
308.15 |
9.51 |
−44.44 |
−28.03 |
−16.41 |
1.83 |
90.50 |
34.78 |
37.19 |
2.92 |
318.15 |
9.90 |
−45.67 |
−23.95 |
−21.72 |
1.81 |
91.49 |
34.53 |
37.44 |
2.90 |
![[thin space (1/6-em)]](https://www.rsc.org/images/entities/char_2009.gif) |
C16mimBr |
0 |
298.15 |
0.610 |
−56.61 |
−33.36 |
−23.24 |
2.00 |
83.14 |
44.53 |
27.44 |
3.42 |
308.15 |
0.631 |
−58.34 |
−29.01 |
−29.33 |
1.83 |
90.50 |
42.29 |
29.68 |
3.40 |
318.15 |
0.637 |
−60.18 |
−24.93 |
−35.25 |
1.61 |
103.33 |
41.74 |
30.23 |
3.34 |
0.02 |
298.15 |
0.601 |
−56.69 |
−25.33 |
−31.36 |
2.22 |
74.83 |
42.64 |
29.33 |
3.48 |
308.15 |
0.624 |
−58.40 |
−20.97 |
−37.42 |
2.05 |
81.02 |
41.25 |
30.72 |
3.40 |
318.15 |
0.632 |
−60.22 |
−16.89 |
−43.33 |
1.76 |
94.44 |
40.39 |
31.58 |
3.34 |
0.12 |
298.15 |
0.597 |
−56.72 |
−17.03 |
−39.69 |
2.24 |
91.51 |
40.15 |
31.82 |
3.83 |
308.15 |
0.621 |
−58.42 |
−12.68 |
−45.74 |
2.12 |
96.67 |
38.71 |
33.26 |
3.62 |
318.15 |
0.629 |
−60.25 |
−8.60 |
−51.65 |
1.87 |
102.13 |
35.45 |
36.52 |
3.47 |
3.2.2 Surface properties of IL-surfactant mixtures. The molecules of the IL-surfactant mixture adsorbed at the interface, even at low surfactant concentration, cause the reduction of the surface tension of water. Possible interactions with the surfactant adsorption are hydrophobic effect, electrostatic interaction, van der Waals interaction, hydrogen bonding,12,14,38,39 etc.It is known that the hydrophobicity of surfactants increases with increasing hydrophobic chain length. Thus the surfactant monomers are more likely to escape the solvent into the air–liquid interface, so that the hydrophilic head group in the water is pulled by the hydrophobic hydrocarbon chain away from the solvent. Therefore, the parameter of ΠCMC in Table 2 reflects that the surface tension of the C16mimBr/CTAB mixture is reduced more than that of C12mimBr/CTAB when CTAB monomers get into solution. While in the C10mimBr/CTAB system, the CTAB micelles almost have no effect in reducing the surface tension of water according to the ΠCMC listed in Table 2.
The adsorption efficiency (pC20) of the surfactant molecules at the air–solution interface can be obtained in terms of the surface tension data. For CnmimBr/CTAB mixtures, it can be easily observed that pC20 values increase when increasing the CTAB content, showing superior adsorption efficiency.14 However, pC20 decreasing with the increment of temperature suggests that elevated temperature is not favorable for micellization, corresponding to CMC trends. It is noted that the C10mimBr/CTAB mixtures exhibit increased pC20 values in the case of CTAB micelles, different from the decrease in the C16mimBr/CTAB mixtures with CTAB monomer added into the solution. So the self-assembled behaviors of surfactant molecules in mixture solution are influenced by the CTAB molecule state at different temperatures.
Additionally, the maximum surface excess concentration (Γmax) is close to pC20, and the minimum area of a single surfactant molecule (Amin) is also estimated in Table 1. Like the temperature effect of pC20 mentioned above, it is shown that the higher the temperature, the greater the value of Amin in the presence of CTAB monomer in solution, while Amin gradually decreases with temperature when CTAB micelles are present in solution. By comparing the data for the three ILs with the same counter ion, it was found that Amin per adsorbed ionic liquid at the air/water interface decreases with the length of the alkyl chain and it implies that the longer the alkyl chains, the more compact the monolayers at the air/water interface.40 Certainly, the values of Γmax have the corresponding opposite trend.14 All these parameter changes can be thought to be related to enhanced molecular motion at elevated temperature, thus leading to fewer surfactant molecules being adsorbed at the air–water interface.1 With an excess of CTAB, more CTAB molecules may participate in surface adsorption of mixture solution, besides self-assembly behavior, for example in the C10mimBr/CTAB mixtures.
3.2.3 Thermodynamic functions of micellization. By applying eqn (8)–(10), the standard free energy change (ΔGm), standard enthalpy (ΔHm) and standard entropy change (ΔSm) associated with the micelle formation were also calculated. In the case of ionic surfactants, according to eqn (5)–(8), the standard free energy change as well as other thermodynamic functions of aggregate formation at different temperatures may be calculated using XCMC,4 because the measurement of the degree of dissociation (α) may be omitted. Using eqn (8)–(10), the thermodynamic parameters of the CTAB with CnmimBr mixed system at different CTAB concentrations are given in Table 2. Comparison to the results from the conductivity measurement (Fig. 4) showed that three thermodynamic parameters with temperature and CTAB concentration displayed a similar change, and here an entropy-driven process can also be clearly observed from the data in Table 2, which indicates a CMC value a little smaller than that determined by the conductivity measurement. The difference in the two results is mainly based on applying different eqn (5) and (8). |
ΔGm = 2RT ln XCMC
| (8) |
|
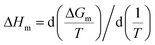 | (9) |
|
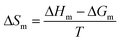 | (10) |
For the conductivity of the mixture solution, the degree of counter-ion dissociation implies that a fraction of the counter-ions on the micelle surface are confined, and the formed micelles can also carry less charge to transport than the free ions.4 Consequently, the α value should change with the number and state of the ionic surfactants in the mixture solution, so it is not a constant “2” in eqn (8), which is an extreme case of α ≈ 0. Theoretically, eqn (5) is more reasonable and accordingly thermodynamic functions shown in Fig. 4 seem to be more convincing.
3.3 FT-IR analysis
According to experimental method mentioned above, FT-IR spectra of C10mimBr, CTAB and their mixture studied in the present work were recorded, as shown in Fig. S3.† The FT-IR spectra of the mixture showed the apparent change with comparison to the pure components. The C–H stretching (C4H, C5H and C2H) of the imidazole ring of IL can be observed at 3050–3200 cm−1,3,41 but becomes weaker after interaction with CTAB in the mixture. It is worth noting that a band located at 1572 cm−1 (C–N stretching) for the IL was weaker in the mixture than in the pure IL. Moreover, the characteristic peak of C–H plane deformation vibration in the imidazole ring significantly moves from 1174 cm−1 to 1168 cm−1. This is evidence of the electrostatic effect between the head groups of IL and CTAB molecules. On the other hand, the band between 2800 and 3050 cm−1 assigned to the asymmetric and symmetric C–H stretching vibration of the methyl groups was seen in C10mimBr, CTAB and their mixture spectra,42 but the slight change corresponds to the hydrophobic interactions between the hydrocarbon chain of the surfactant CTAB and IL cation.
3.4 1H NMR
The 1H NMR spectra were recorded to understand the various intermolecular interactions present among IL and CTAB molecules in aqueous solutions. In this part, the effect of CTAB on the NMR microenvironment of the CnmimBr was investigated and all protons of CnmimBr in the mixed system were analyzed. The 1H NMR spectrum of the IL in Fig. 8 reveals nine characteristic peaks. It can be seen that the characteristic proton signals of Ha–He from CTAB gradually become higher and higher with increasing CTAB. It is well known that the chemical shifts of IL protons move upfield or downfield depend on the shielding and deshielding effects of surrounding groups. The greater the electronic density, the greater the shielding effect. Owing to addition of CTAB, all upfield shifts in the tail chain of the IL proton signals of H7-11 reflect the hydrophobic interactions present among the hydrophobic chain of the surfactant and alkyl chains of the IL cation, which led to shielding of the surrounding protons.12
 |
| Fig. 8 1H NMR spectra of C10mimBr in D2O solution with different wt% of CTAB. | |
In addition, the evident downfield shift of the head protons (H2, 4, 5) of the IL was observed. This may be interpreted that after the addition of CTAB, increased counterion dissociation from CTAB into solution leads to a stronger electrostatic attractive effect between the Br− and the imidazolium cation than electrostatic repulsion, thus the lower electronic density of the imidazolium cation results in the signals of H2, 4, and 5 shifting toward lower field.27 However, an upfield shift was also observed for protons of the imidazole ring (H6), but not significant in comparison with the tail region protons (H8–11) because the magnetic environment of proton H6 located at the micellar surface would not be changed so remarkably after addition of CTAB.
4 Conclusion
The present study presents the surface and thermodynamic properties of the CnmimBr/CTAB surfactants system in aqueous media using surface tension and conductivity methods. For all the systems, the surface activity and CMC values of the CnmimBr/CTAB (n = 10, 12, 16) systems are highly dependent on the CTAB concentration and temperature. The CMC values systematically decreased with the CTAB concentration and an inverse trend was observed when temperature was increased; such dependence is analogous with that of conventional ionic surfactants. It was further perceived that adsorption proficiency decreased at high CTAB monomer concentrations, while the opposite result was also observed in the presence of CTAB micelles. On the other hand, the minimum area per adsorbed molecule decreased sharply with CTAB concentration, indicating that compact mono-layers are formed at the air/water interface and facilitate the formation of well-defined aggregates in the bulk. The value of surface excess concentration (Γmax) implies that the adsorption of IL in the mixture system decreases with increasing CTAB content, while opposite trends were observed in other surfactant systems. For all the mixed systems, the negative ΔGm presented a decrease with temperature and CTAB concentration, suggesting that all micellization processes were spontaneous. The ΔHm and ΔSm values for all the mixed systems were dependent on the CTAB molecule state, as the CTAB monomer and micelle led to an opposite change trend of ΔHm and ΔSm for the mixed system. FT-IR and NMR data provide evidence of the interaction between the IL and CTAB molecules in the mixture system to best understand the cause of the final results. By comparison of the traditional surfactants with CTAB addition into ionic liquid surfactants to form a surfactants mixture, it is important to reveal the higher surface activity. Therefore, similar to classical cationic surfactants, the active surface of such a surfactant mixture forms well defined aggregates at the air/water interface in bulk solution and has potential for the development of novel green surface-active agents. It is clear that the present investigations provide a fundamental understanding of the solution properties of the binary system. Our work herein will also be useful for designing separation technologies and would be further useful in developing new technologies for applying ILs to colloid and interfacial science chemical engineering fields as well.
Conflicts of interest
All authors declare no financial/commercial conflicts of interest.
Acknowledgements
The authors are grateful for the open project of Jiangsu Key Laboratory for Bioresources of Saline Solis (JKLBS2016012), and Jiangsu Province College Students' Innovative Training Project (201610324044x) and a Project Funded by the Excellent Specialties Program Development of Jiangsu Higher Education Institutions, Project (No. PPZY2015B113).
References
- B. Dong, X. Y. Zhao, L. Q. Zheng, J. Zhang, N. Li and T. Inoue, Colloids Surf., A, 2008, 317, 666–672 CrossRef CAS.
- J. Saien and S. Asadabadi, Fluid Phase Equilib., 2015, 386, 134–139 CrossRef CAS.
- K. Li and T. Kobayashi, Ultrason. Sonochem., 2016, 28, 39–46 CrossRef CAS PubMed.
- X. W. Li, Y. A. Gao, J. Liu, L. Q. Zheng, B. Chen, L. Z. Wu and C. H. Tung, J. Colloid Interface Sci., 2010, 343, 94–101 CrossRef CAS PubMed.
- Y. Li, M. Zhang, Q. Liu and H. Su, J. Chem. Thermodyn., 2013, 66, 80–87 CrossRef CAS.
- P. Bharmoria, T. Singh and A. Kumar, J. Colloid Interface Sci., 2013, 407, 361–369 CrossRef CAS PubMed.
- J. Luczak, M. Paszkiewicz, A. Krukowska, A. Malankowska and A. Zaleska-Medynska, Adv. Colloid Interface Sci., 2016, 230, 13–28 CrossRef CAS PubMed.
- S. Livi, J. Duchet-Rumeau, T. N. Pham and J. F. Gerard, J. Colloid Interface Sci., 2010, 349, 424–433 CrossRef CAS PubMed.
- F. Comelles, I. Ribosa, J. J. Gonzalez and M. T. Garcia, Colloids Surf., A, 2015, 484, 136–143 CrossRef CAS.
- O. Nacham, A. Martín-Pérez, D. J. Steyer, M. J. Trujillo-Rodríguez, J. L. Anderson, V. Pino and A. M. Afonso, Colloids Surf., A, 2015, 469, 224–234 CrossRef CAS.
- S. Chabba, S. Kumar, V. K. Aswal, T. S. Kang and R. K. Mahajan, Colloids Surf., A, 2015, 472, 9–20 CrossRef CAS.
- S. Zhang, Y. Gao, B. Dong and L. Zheng, Colloids Surf., A, 2010, 372, 182–189 CrossRef CAS.
- K. Thakkar, B. Bharatiya, D. O. Shah, D. Ray, V. K. Aswal and P. Bahadur, Colloids Surf., A, 2015, 484, 547–557 CrossRef CAS.
- T. Inoue, K. Kawashima and Y. Miyagawa, J. Colloid Interface Sci., 2011, 363, 295–300 CrossRef CAS PubMed.
- J. Liu, L. Zheng, D. Sun and X. Wei, Colloids Surf., A, 2010, 358, 93–100 CrossRef CAS.
- K. D. Danov, P. A. Kralchevsky and K. P. Ananthapadmanabhan, Adv. Colloid Interface Sci., 2014, 206, 17–45 CrossRef CAS PubMed.
- D. Bhatt, K. Maheria and J. Parikh, J. Chem. Thermodyn., 2014, 74, 184–192 CrossRef CAS.
- J. M. Padró and M. Reta, J. Mol. Liq., 2016, 213, 107–114 CrossRef.
- I. J. Warke, K. J. Patil and S. S. Terdale, J. Chem. Thermodyn., 2016, 93, 101–114 CrossRef CAS.
- L. M. Bergstrom and V. M. Garamus, J. Colloid Interface Sci., 2012, 381, 89–99 CrossRef PubMed.
- A. Pal and A. Pillania, J. Mol. Liq., 2015, 212, 818–824 CrossRef CAS.
- S. Chauhan, A. Kumar, M. Kaur and M. S. Chauhan, J. Surfactants Deterg., 2017, 20, 1129–1139 CrossRef CAS.
- J. Łuczak, A. Latowska and J. Hupka, Colloids Surf., A, 2015, 471, 26–37 CrossRef.
- V. G. Rao, S. Mandal, S. Ghosh, C. Banerjee and N. Sarkar, J. Phys. Chem. B, 2012, 116, 13868–13877 CrossRef CAS PubMed.
- X. Wang, J. Liu, L. Yu, J. Jiao, R. Wang and L. Sun, J. Colloid Interface Sci., 2013, 391, 103–110 CrossRef CAS PubMed.
- S. Chauhan, V. Sharma, K. Singh, M. S. Chauhan and K. Singh, J. Mol. Liq., 2016, 222, 67–76 CrossRef CAS.
- A. Pal and A. Pillania, Fluid Phase Equilib., 2015, 389, 67–73 CrossRef CAS.
- A. Pal and A. Pillania, J. Mol. Liq., 2015, 209, 6–13 CrossRef CAS.
- P. Wang, X.-F. Guo, L.-H. Lia, C.-M. Ma and L. Qian, Chin. J. Appl. Chem., 2013, 30, 840–845 CAS.
- A. Pal and S. Chaudhary, J. Mol. Liq., 2015, 207, 67–72 CrossRef CAS.
- A. Pal and A. Pillania, Fluid Phase Equilib., 2016, 412, 115–122 CrossRef CAS.
- S. Chauhan, Atika, K. Singh, K. Singh, M. Kaur and M. S. Chauhan, J. Mol. Liq., 2017, 242, 1066–1074 CrossRef CAS.
- A.-D. M. Sørensen, K. S. Lyneborg, P. Villeneuve and C. Jacobsen, J. Funct. Foods, 2015, 18, 959–967 CrossRef.
- B. Sohrabi, S. Eivazzadeh, A. Sharifi and R. Azadbakht, J. Mol. Liq., 2015, 211, 754–760 CrossRef CAS.
- S. Chauhan and M. Kaur, J. Surfactants Deterg., 2017, 20, 599–607 CrossRef CAS.
- S. Chauhan, S. Kumari and K. Singh, J. Chem. Thermodyn., 2017, 105, 337–344 CrossRef CAS.
- G.-Y. Wang, Y.-Y. Wang and X.-H. Wang, J. Mol. Liq., 2017, 232, 55–61 CrossRef CAS.
- M. N. Garaga, M. Nayeri and A. Martinelli, J. Mol. Liq., 2015, 210, 169–177 CrossRef CAS.
- H. Y. He, H. Chen, Y. Z. Zheng, S. J. Zhang and Z. W. Yu, Chem. Eng. Sci., 2015, 121, 169–179 CrossRef CAS.
- N. V. Sastry, N. M. Vaghela and V. K. Aswal, Fluid Phase Equilib., 2012, 327, 22–29 CrossRef CAS.
- Y. Jiang, L. Wang, L. Zhang, T. Wang, Y. Zhou, C. Ding, R. Yang, X. Wang and L. Yu, Int. J. Biol. Macromol., 2015, 79, 533–541 CrossRef CAS PubMed.
- C. Azémard, C. Vieillescazes and M. Ménager, Microchem. J., 2014, 112, 137–149 CrossRef.
Footnote |
† Electronic supplementary information (ESI) available. See DOI: 10.1039/c7ra08915e |
|
This journal is © The Royal Society of Chemistry 2017 |
Click here to see how this site uses Cookies. View our privacy policy here.