DOI:
10.1039/C7RA08865E
(Paper)
RSC Adv., 2017,
7, 42513-42518
Cucurbit[7]uril complexations of Good's buffers†
Received
10th August 2017
, Accepted 27th August 2017
First published on 1st September 2017
Abstract
The cucurbit[7]uril (CB[7]) host–guest complexations of a series of zwitterionic “Good's” biological pH buffers have been investigated in aqueous solution by means of 1H NMR spectroscopy. The cyclohexylammonium buffers bind very strongly (KCB[7] = 107 to 108 dm3 mol−1), while the morpholinium (102 to 103 dm3 mol−1) and piperazinium (103 to 104 dm3 mol−1) buffers have binding constants several orders of magnitude smaller. The binding constants increase as the distance between the ammonium and sulfonate groups increases. The pKa of 2-(cyclohexylammonio)ethanesulfonate (CHES) increases by 3.1 units upon complexation by CB[7].
Introduction
In 1966, N. E. Good proposed criteria for an optimum biological buffer,1 and his group2,3 and others4 reported the syntheses of several series of zwitterionic compounds (Fig. 1) which are now standard buffers employed in biochemistry. The criteria included easy synthetic preparation (with a resistance to degradation), pKa values between 6 and 8 (with a minimal dependence on temperature, concentration, or ionic strength), water solubility, impermeability to biological membranes, and a lack of binding to other biological cations. The majority of the Good's buffers bear sulfonate groups, which are anionic at physiological pH and enhance the water solubility of the buffer, and tertiary amine groups, whose protonated forms have pKa values near to or above 7.
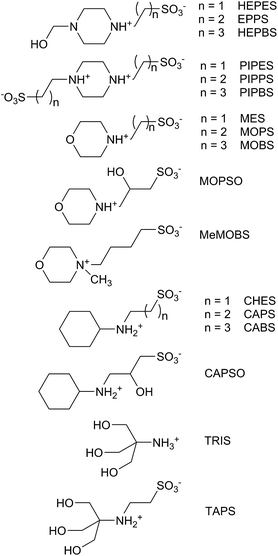 |
| Fig. 1 Good's type biological pH buffers used in this study. | |
As a result of their zwitterionic nature, the Good's buffers have recently been used as the anionic or cationic components of ionic liquids (GBILs), for use as self-buffering and biocompatible media for protein and antibody extractions.5–10 The Good's buffers have also been employed to stabilize platinum and gold nanoparticles, affecting their structures and optical properties.11–16
Despite the proposed criteria, a number of the buffers result in metal ion depletion (notably copper)17–23 and interactions with biological systems, leading to unwanted or beneficial physiological effects.24,25 The former problem has been remedied to some degree by “Better” pH buffers such as PIPES (Fig. 1), which are non-complexing towards metal ions.26,27 There has, however, been little in the way of studies on the interactions of these pH buffers with macrocyclic host molecules,28–30 such as cyclodextrins, calixarenes, and cucurbiturils, which are of increasing interest in biological, biochemical, and medicinal applications.31–34
In this study, the host–guest complexations of a series of Good's (and related) pH buffers (Fig. 1) by the macrocyclic host molecule cucurbit[7]uril (CB[7]) have been studied using 1H NMR spectroscopy. The cucurbit[n]urils (CB[n], n = 5–8, 10 and 13–15) are a family of host molecules comprised of cyclic oligomers of n glycoluril units bridged by 2n methylene groups.34–36 The CB[n] hosts form very stable (KCB[n] up to 1017 dm3 mol−1)37 host–guest complexes with organic cations in aqueous solution, utilizing dipole–dipole and ion-dipole interactions at the polar portals and hydrophobic interaction in the cavity. One feature of the binding of basic organic guests is the preferred complexation of their conjugate acid, resulting in an increase in the pKa value.38–41
Cucurbiturils have recently gained interest in biological, biochemical and medicinal applications, as studies have suggested little evidence of toxicity.42–45 For pH control in these cucurbituril host–guest studies, acetate and phosphate buffers are most commonly used, although TRIS (tris(hydroxymethyl)methylamine) has been used in a few instances,46–49 including early studies of CB[7] with the methyl viologen dication (MV2+).46,47 Ong and Kaifer47 noted that binding constant for MV2+, measured in 30 mmol dm−3 TRIS, was about 10% higher than previously reported in 50 mmol dm−3 TRIS,45 and suggested that the buffer can compete for the host, as found for alkali metal and alkali earth cations.50–52
The cucurbit[7]uril complexations of the four series of Good's buffers, based on cyclohexylammonium, piperazinium, N-2-hydroxyethylpiperazinium, morpholinium, and tris(hydroxymethyl)methylammonium, with alkanesulfonate group substituents, have been investigated using 1H NMR spectroscopy. The host-guest stability constants and the complexation induced guest proton chemical shift values have been determined and related to the nature of cationic portion of the buffer and the length of the alkane chain separating it from the sulfonate group(s). The effect of CB[7] complexation of CHES on its pKa value is also reported.
Experimental
Materials
The cucurbit[7]uril was prepared and characterized by the method of Day et al.53 The majority of the buffers were used as received (Sigma-Aldrich or GFS Chemicals (for PIPPS)). The MOBS, MeMOBS and CABS compounds were prepared by the method of Yi et al.,54 employing 1,4-butane sultone with morpholine, N-methylmorpholine, or cyclohexylamine, respectively. The PIPBS buffer was prepared as described by Jermyn.55
MOBS. 60% yield. 1H NMR (300 MHz, D2O) δ 4.01 (br, 2H), 3.75 (br, 2H), 3.44 (br, 2H), 3.14 (t, 2H, J = 7.3 ppm, overlapping br peak, 2H), 2.88 (t, 2H, 7.3 Hz), 1.90–1.65 (m, 6H) ppm. 13C NMR (75 MHz, D2O) δ 63.85, 56.55, 51.60, 49.95, 21.92, 21.19 ppm.
MeMOBS. 43% yield. 1H NMR (300 MHz, D2O) δ 3.97 (br, 4H), 3.42 (m, 8H), 3.11 (s, 3H), 2.90 (t, 2H, J = 7.5 Hz), 1.88 (m, 2H), 1.75 (qn, 2H, J = 7.1 Hz) ppm. 13C NMR (75 MHz, D2O) δ 64.33, 60.31, 59.59, 49.91, 46.79, 21.04, 19.85 ppm.
CABS. 48% yield. 1H NMR (300 MHz, D2O) δ 3.00 (m, 3H), 2.87 (m, 2H), 1.98 (br, 2H), 1.74 (br, 2H), 1.73 (m, 4H), 1.58 (d, J = 12.3 Hz, 1H), 1.23 (qn, J = 12.3 Hz, 4H), 1.11 (m, 1H) ppm. 13C NMR (75 MHz, D2O) δ 57.09, 50.07, 43.78, 28.86, 24.62, 23.89, 21.33 ppm.
PIPBS. 58% yield. 1H NMR (300 MHz, D2O) δ 3.66 (br, 8H), 3.25 (t, 4H, J = 7.9 Hz), 2.87 (t, 4H, J = 7.3 Hz), 1.86 (qn, 4H, J = 7.9 Hz), 1.75 (qn, 4H, J = 7.3 Hz) ppm. 13C NMR (75 MHz, D2O/DCl) δ 56.07, 49.35, 43.77, 29.33, 21.58 ppm.
Methods
The 1D and 2D 1H and 13C NMR spectra were recorded on Bruker Avance 300 and 400 instruments. The host–guest binding constants were determined from 1H NMR titrations or by 1H NMR competitive binding experiments in D2O at pD = 4.75 (0.050 mol dm−3 NaOAc-d3/0.025 mol dm−3 DCl). The competitor guest used was 3-(trimethylsilyl)propionic-2,2,3,3-d4 acid (TSP),56 with a reported CB[7] binding constant of (1.82 ± 0.22) × 107 dm3 mol−1. The stability constants for weaker CB[7] complexes were determined from either non-linear least squares fitting of 1H NMR chemical shift titrations or from Benesi–Hildebrand plots (1/Δδ vs. 1/CB[7]).57 For the PIPBS buffer, slow exchange behaviour is observed in the NMR titrations, and the value of KCB[7] was calculated by integrating the free and bound methine proton resonances of CB[7]. The limiting chemical shift changes, for the guest protons on weaker binding guests, were determined by extrapolation in fitting the chemical shift titrations.
Results and discussion
The 1H NMR titrations of the Good's buffers with CB[7] (Fig. 2) provide a measurement of the host–guest stability constants (Table 1) and the limiting complexation-induced chemical shift changes for the guest proton resonances (Δδlim = δbound − δfree) give an indication of the average position of the guest within the CB[7] cavity (Fig. 3, S1, S9, S20†). Upfield shifts (Δδlim < 0 ppm) for the proton resonances indicate positioning within the cavity, while downfield shifts are indicative of the protons located outside of the cavity near the polar portal(s) of CB[7]. In the buffers which bind to the CB[7] in this study, the values of Δδlim (Fig. 3, S1, S9, S20†) indicate that the host is binding primarily over the ring (and the hydroxyethyl group for HEPES, EPPS, and HEPBS, Fig. 3 and S20†), rather than the alkylsulfonate chain.
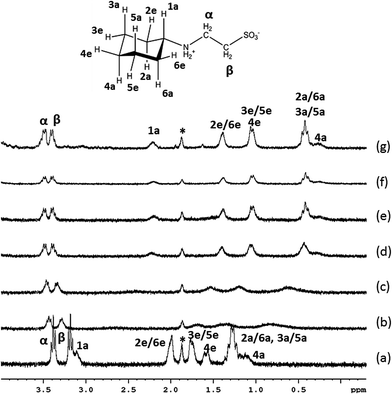 |
| Fig. 2 1H NMR titration of CHES with (a) 0.00, (b) 0.57, (c) 0.80, (d) 0.96, (e) 1.05, (f), 1.73, and (g) 4.20 equivalents of CB[7] in D2O (pD = 4.75, 0.050 mol dm−3 NaOAc-d3(*)/0.025 mol dm−3 DCl). | |
Table 1 Host–guest stability constants for CB[7] with the Good's buffers in D2O (pD = 4.75, 0.050 mol dm−3 NaOAc-d3/0.025 mol dm−3 DCl)
Buffer |
pKa |
Δδlim(Hα), ppm |
KCB[7], dm3 mol−1 |
Ref. 58. Ref. 4. Ref. 27. Ref. 3. Ref. 59. Ref. 60. Ref. 61. |
HEPES |
7.55a |
+0.43 |
(2.3 ± 0.3) × 103 |
EPPS |
8.0b |
+0.27 |
(8.9 ± 1.3) × 103 |
HEPBS |
8.3b |
+0.04 |
(2.1 ± 0.4) × 104 |
PIPES |
2.67, 6.78c |
0 |
0 |
PIPPS |
3.79, 7.97c |
0 |
0 |
PIPBS |
4.29, 8.55c |
+0.15 |
(2.2 ± 0.6) × 103 |
MES |
6.06c |
−0.02 |
210 ± 30 |
MOPSO |
6.95d |
−0.19, −0.32 |
330 ± 50 |
MOPS |
7.09c |
−0.25 |
(2.2 ± 0.3) × 103 |
MOBS |
7.48c |
−0.40 |
(2.4 ± 0.3) × 103 |
MeMOBS |
|
−1.05 |
(4.2 ± 0.6) × 104 |
CHES |
9.27e |
+0.10 |
(3.6 ± 0.5) × 107 |
CAPSO |
9.6f |
+0.02, +0.13 |
(6.0 ± 0.9) × 107 |
CAPS |
10.35e |
−0.02 |
(1.0 ± 0.2) × 108 |
CABS |
10.7b |
+0.03 |
(1.3 ± 0.2) × 108 |
TRIS |
8.06a |
|
290 ± 50 |
TAPS |
8.4g |
−0.01 |
110 ± 20 |
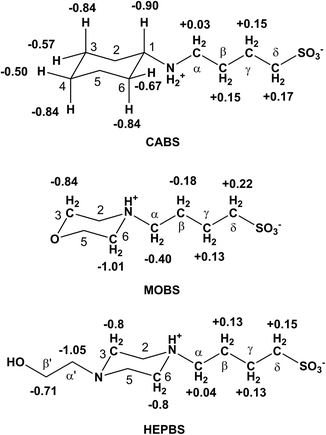 |
| Fig. 3 CB[7] complexation-induced chemical shift changes, Δδlim (ppm), for representative buffers. | |
The strongest binding by CB[7] was observed for the cyclohexylammonium buffers (Fig. 2, S1–S8†), with stability constants in the range of 107 to 108 dm3 mol−1 (Table 1). It is known that the cyclohexylammonium cation binds strongly to cucurbit[7]uril, as a result of the hydrophobic interactions of the cyclohexyl ring with the cavity of CB[7] and the ion-dipole interactions of the ammonium group at the carbonyl portal. Values of KCB[7] = (2.4 ± 0.4) × 107 dm3 mol−1 (0.10 mol dm−3 Na3PO4/DCl, pH = 7.4) and 1.1 × 108 dm3 mol−1 (0.050 mol dm−3 NaOAc, pH 4.0) been reported by Cao and Isaacs,62 and Li and Kaifer,63 respectively. Yu et al. have reported a value of (3.1 ± 0.1) × 106 dm3 mol−1 (30 °C, pH = 4.0) from an ITC experiment.64 Cao and Isaacs have also observed that increasing the size of the ring in cycloalkylammonium cations increases the value of KCB[7],62 while Yu et al. observed a slight increase in the binding constant with the N-methylcyclohexylammonium cation.64 The introduction of a hydroxyl group on the β-carbon in CAPSO results in a slight reduction in the binding constant compared to CAPS.
The values of KCB[7] increase with an increase in the number of methylene groups between the protonated nitrogen and the sulfonate group (Table 1). This would reduce the repulsions between the sulfonate group and the polar carbonyl groups on the CB[7] portal and increase the hydrophobicity of the guest.
The morpholinium buffers (MES, MOPS, MOPSO, and MOBS) exhibit lower binding constants than the corresponding cyclohexylammonium buffers (Table 1), with KCB[7] in the range of 200–2400 dm3 mol−1, increasing with an increase in the alkylsulfonate chain length (Fig. 4, 5 and S10–S17†). This trend in higher KCB[7] values is also accompanied by a deeper inclusion of the morpholine ring within the cavity, as exhibited by the greater upfield chemical shifts of the methylene groups which are attached to the protonated nitrogen (Hα: −0.01, −0.23, and −0.35 ppm for MES, MOPS, and MOBS, respectively (Fig. 3, S9†)). The MOPSO buffer has a slightly lower binding constant than MOPS, similar to that seen for CAPSO compared with CAPS.
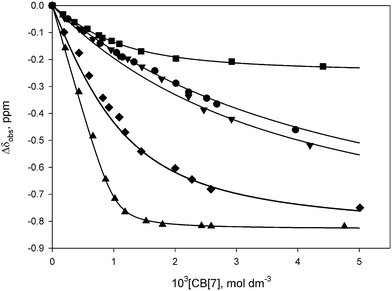 |
| Fig. 4 1H NMR titrations of MES (● H3,5), MOPS (■ H3,5), MOPSO (▼H3,5), MOBS (♦ H3,5), and MeMOBS (▲ HMe) with CB[7] in D2O (pD = 4.75, 0.050 mol dm−3 NaOAc-d3/0.025 mol dm−3 DCl). | |
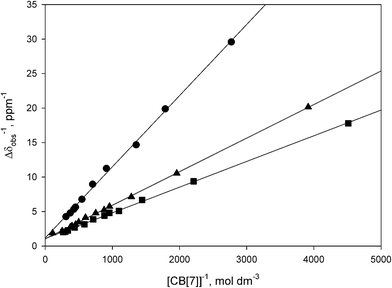 |
| Fig. 5 Benesi–Hildebrand plots for the binding of TRIS (●), TAPS (■), and MES (▲) with CB[7] in D2O (pD = 4.75, 0.050 mol dm−3 NaOAc-d3(*)/0.025 mol dm−3 DCl). | |
Among the other zwitterionic guests investigated previously with CB[7], is carnitine which has also has a β-hydroxypropyl chain separating a trimethylammonium group (resides inside the CB[7] cavity) from a carboxylate group.65 The binding constant of KCB[7] = 8.0 × 104 dm3 mol−1 for carnitine is between the values observed for MOPSO and CAPSO.
The N-methyl derivative of MOBS (MeMOBS) exhibited a higher binding constant of (4.2 ± 0.5) × 104 dm3 mol−1, a factor of 20 compared to that of MOBS, with a very large upfield shift of −1.05 ppm for Hα (Fig. S19 and S20†). We have reported a similar increase in CB[7] binding of the zwitterionic Nε,Nε,Nε-trimethyllysine (6.0 × 104 dm3 mol−1) compared with protonated dimethyllysine (2.1 × 103 dm3 mol−1).66 In both sets of guests, the replacement of a proton by a methyl group makes the ammonium center more hydrophobic and more included within the CB[7] cavity, as reflected in the values of Δδlim.
The host–guest stability constants for this group of buffers are much lower than reported for protonated or alkylated morpholine guest molecules with CB[7], with KCB[7] = (2.3 ± 0.4) × 106 and (5.1 ± 0.8) × 106 dm3 mol−1 for the protonated N-methylmorpholine and N,N-dimethylmorpholinium, respectively.67 The sulfonate group on the alkyl substituent of the morpholinium buffers is likely responsible for their weaker binding to CB[7].
In the piperazinium family of buffers (HEPES, EPPS, and HEPBS), there is hydroxyethyl substituent on one of the N atoms, in addition to the alkylsulfonate substituents found in the cyclohexylammonium and morpholinium buffers. As with the other two sets of buffers, the magnitude of KCB[7] (Table 1, Fig. S20–S26†) increases with alkylsulfonate chain length, with values about an order of magnitude greater than the corresponding morpholinium buffers. Either N atom could be the site of protonation of these buffers in slightly acidic solution, and for HEPES, both protonation tautomers have been observed in the solid state.68–70 Upon complexation by CB[7], the large upfield shifts in the hydroxyethyl proton resonances is suggestive of inclusion of this group and the piperazine ring within the host cavity, with the protonated nitrogen in proximity to one of the portals. The Δδlim values for the Hα′ and Hα resonances for HEPES and EPPS are larger in magnitude than normally seen for fully included guest protons (Fig. S20†). The chemical shift change for Hα′ is likely a combination of the shielding within the cavity plus an upfield shift as the buffer transitions between a tautomer equilibrium to a localized protonation on the N atom bearing the alkylsulfonate group. For the Hα resonance, this would lead to a greater downfield shift than strictly from deshielding effects of the CB[7] portal. The similarities in the resonance positions for the alkylsulfonate methylene protons, for the piperazinium buffers compared with those of the other two sets, suggests that the nitrogen bearing the alkylsulfonate group is the site of protonation on the complexed buffer. The piperazinium ring proton resonances become very broad (and difficult to locate) upon complexation (Fig. S21, S23, S25†), as the ring flipping rate approaches the NMR timescale.67
The replacement of the hydroxyethyl group on the aforementioned piperazinium buffers with another alkylsulfonate group gives rise to the diprotic bases PIPES, PIPPS, and PIPBS. With PIPES and PIPPS, no change in the guest proton resonances are observed upon addition of 5-fold CB[7] at low pH (pH 1–2, both N atoms protonated), pH 5 (one N atom protonated), and high pH (pH 11–12, both N atoms nonprotonated) (Fig. S27 and S28†). The formation of a host–guest complex would require the host to pass over one of the sulfonate groups to bind to the central piperazinium group. This, combined with the presence of repulsive sulfonate groups near each of the two CB[7] polar portals, would not provide for very stable internal host–guest (“pseudorotaxane”) complexes. With PIPBS, the longer alkyl chains would reduce the ion-dipole repulsions, and changes in the guest proton resonances upon addition of CB[7] (Fig. S29†), with slow exchange behaviour, are observed for both the buffer and CB[7] proton resonances. A binding constant of 2.2 × 103 dm3 mol−1 for PIPBS was determined using the integrations of the free and bound methine proton resonances.
The tris(hydroxymethyl)methylammonium buffers TRIS and TAPS bind weakly to CB[7] (Table 1, Fig. 5, S30–S33†), with TAPS exhibiting a lower binding constant as a result of the pendant N-ethylsulfonate group. The binding constants were determined by Benesi–Hildebrand plots using the methylene proton resonance of the hydroxymethyl groups (Fig. 5). The methylene proton resonances for the encapsulated hydroxymethyl arms of TRIS and TAPS shift upfield by −0.92 and −0.85 ppm, respectively, while the ethylsulfonate group of the latter buffer remains outside the cavity of CB[7].
pKa shift of CHES
The complexation of acidic guest molecules by cucurb[7]uril is known to increase the guest pKa value compared to that of the free acid. A pH titration of the CB[7]-complexed CHES buffer (pKa of free CHES is 9.27)58 was carried out in basic solution (Fig. 6). The pKa of the bound CHES was found to be 12.37, representing an increase of 3.1 units, as the deprotonation of the quaternary nitrogen results in weaker binding to the anionic guest species. This may be compared to a shift of 1.3 pKa units for cyclohexylmethylamine upon binding to CB[6].71 The larger shift for CHES with CB[7] would be consistent with a greater reduction in the binding of the conjugate base, because of the sulfonate group. The ΔpKa value of 3.1 for CHES allows for a binding constant estimation (KbCB[7] = KaCB[7](10−ΔpKa))38,39 of KbCB[7] = 3 × 104 dm3 mol−1 for the conjugate base of CHES. The binding constant for this form of CHES with the β-cyclodextrin (β-CD) host has been reported to be 490 ± 20 dm3 mol−1 (pH 10.5, with a similar value for CAPS)28 and 440 ± 30 dm3 mol−1 (pH 11.6).29 The zwitterionic form of CHES binds very much weaker (about 30 dm3 mol−1) with β-CD, which would arise from a decrease in the pKa value upon complexation.29 The strong binding of the cyclohexylammonium buffers to CB[7], along with significant pKa shifts, would make them inappropriate as biological pH buffers with cucurbit[7]uril.
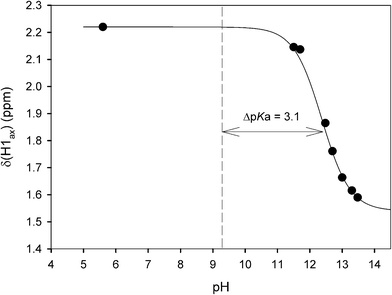 |
| Fig. 6 pH titration of the chemical shift of the CB[7] complex of CHES. The dashed vertical line represents the pKa of free CHES. | |
Conclusions
The macrocyclic host molecule cucurbit[7]uril exhibits a wide range of binding constants with the Good's biological pH buffers in aqueous solution. The CB[7] binding constants are observed to increase as the anionic sulfonate group is placed further from the protonated nitrogen site. The morpholinium (MES, MOPS, MOPSO) and tris(hydroxymethyl)methylammonium (TRIS, TAPS) buffers exhibit binding constants of <103 dm3 mol−1 and could be used in studies with guests which exhibit significantly stronger binding with CB[7]. We are presently looking at reducing the CB[7] binding strength by changing the alkyl spacer between the nitrogen and sulfonate groups to methane.72 The dibasic PIPES and PIPBS buffers would be useful for buffering biological CB[7] solutions over a wide pH range as internal pH indicators for 1H NMR spectroscopy73 with CB[7]. A mixture of PIPES (pKa1 = 2.67 and pKa2 = 6.78) and acetic acid (pKa = 4.75) would cover a significant pH range, and we are currently utilizing them in determining pKa shifts of polyprotic drug molecules upon complexation by CB[7].
Conflicts of interest
There are no conflicts to declare.
Acknowledgements
The financial support of this research by the Natural Sciences and Engineering Research Council of Canada, in the form of a Discovery Grant, is gratefully acknowledged.
Notes and references
- N. E. Good, G. D. Winget, W. Winter, T. N. Connolly, S. Izawa and R. M. M. Singh, Biochemistry, 1966, 5, 497 CrossRef.
- N. E. Good and S. Izawa, Methods Enzymol., 1972, 24, 53 CAS.
- W. J. Ferguson, K. I. Braunschweiger, W. R. Braunschweiger, J. R. Smith, J. J. McCormick, C. C. Wasmann, N. P. Jarvis, D. H. Bell and N. E. Good, Anal. Biochem., 1980, 104, 300 CrossRef CAS PubMed.
- T. Thiel, L. Liczkowski and S. T. Bissen, J. Biochem. Biophys. Methods, 1998, 37, 117 CrossRef CAS PubMed.
- M. Taha, F. A. e. Silva, M. V. Quental, S. P. M. Ventura, M. G. Freire and J. A. P. Coutinho, Green Chem., 2014, 16, 3149 RSC.
- M. Taha, M. R. Almeida, F. A. e. Silva, P. Domingues, S. P. M. Ventura, J. A. P. Coutinho and M. G. Freire, Chem.–Eur. J., 2015, 21, 4781 CrossRef CAS PubMed.
- S. Y. Lee, F. A. Vicente, F. A. e. Silva, T. E. Sintra, M. Taha, I. Khoiroh, J. A. P. Coutinho, P. L. Show and S. P. M. Ventura, ACS Sustainable Chem. Eng., 2015, 3, 3420 CrossRef CAS.
- B. S. Gupta, M. Taha and M.-J. Lee, RSC Adv., 2015, 5, 106764 RSC.
- B. S. Gupta, M. Taha and M.-J. Lee, RSC Adv., 2016, 6, 18567 RSC.
- M. Taha, M. V. Quental, F. A. e Silva, E. V. Capela, M. G. Freire, S. P. M. Ventura and J. A. P. Coutinho, J. Chem. Technol. Biotechnol., 2017, 92, 2287 CrossRef CAS.
- A. Habib, M. Tabata and Y. G. Wu, Bull. Chem. Soc. Jpn., 2005, 78, 262 CrossRef CAS.
- J. Xie, J. Y. Lee and D. I. C. Wang, Chem. Mater., 2007, 19, 2823 CrossRef CAS.
- R. K. Gupta, V. Kumar, A. Srivastava and D. S. Pandey, RSC Adv., 2016, 6, 40911 RSC.
- C. Engelbrekt, M. Wagner, M. U.-B. Christiansen, H. E. M. Christensen, X. Qian, J. Ulstrup, C. Zhao and J. Zhang, ChemElectroChem, 2016, 3, 1212 CrossRef CAS.
- K. Chandra, K. S. B. Culver, S. E. Werner, R. C. Lee and T. W. Odom, Chem. Mater., 2016, 28, 6763 CrossRef CAS.
- E. R. Clark and D. M. Kurtz Jr, Inorg. Chem., 2017, 56, 4584 CrossRef CAS PubMed.
- J. M. Pope, P. R. Stevens, M. T. Angotti and R. Nakon, Anal. Biochem., 1980, 103, 214 CrossRef CAS PubMed.
- R. Nakon and C. R. Krishnamoorthy, Science, 1983, 221, 749 CAS.
- H. A. Azab, F. S. Deghaidy, A. S. Orabi and N. Y. Farid, J. Chem. Eng. Data, 2000, 45, 709 CrossRef CAS.
- M. Sokołowska and W. Bal, J. Inorg. Biochem., 2005, 99, 1653 CrossRef PubMed.
- M. Taha, R. A. Saqr and A. T. Ahmed, J. Chem. Thermodyn., 2007, 39, 304 CrossRef CAS.
- Z. M. Anwar and H. A. Azab, J. Chem. Eng. Data, 2011, 46, 34 CrossRef.
- C. M. H. Ferreira, I. S. S. Pinto, E. V. Soares and H. M. V. M. Soares, RSC Adv., 2015, 5, 30989 RSC.
- S. O. Ugwu and S. P. Apte, Pharm. Technol., 2004, 28, 86 CAS.
- B. S. Gupta, M. Taha and M.-J. Lee, Phys. Chem. Chem. Phys., 2015, 17, 1114 RSC.
- Q. Yu, A. Kandegedara, Y. Xu and D. B. Rorabacher, Anal. Biochem., 1997, 253, 50 CrossRef CAS PubMed.
- A. Kandegedara and D. B. Rorabacher, Anal. Chem., 1999, 71, 3140 CrossRef CAS PubMed.
- M. E. Georgiou, C. A. Georgiou and M. A. Kouppans, Anal. Chem., 1995, 67, 114 CrossRef CAS.
- M. Riesová, J. Svobodová, Z. Tošner, M. Beneš, E. Tesařová and B. Gaš, Anal. Chem., 2013, 85, 8518 CrossRef PubMed.
- M. Beneš, M. Riesová, J. Svobodová, E. Tesařová, P. Dubský and B. Gaš, Anal. Chem., 2013, 85, 8526 CrossRef PubMed.
- M. E. Davis and M. E. Brewster, Nat. Rev. Drug Discovery, 2004, 3, 1023 CrossRef CAS PubMed.
- C. Adeoye and H. Cabral-Marques, Int. J. Pharm., 2017 DOI:10.1016/j.ijpharm.2017.04.050.
- F. Perret and A. W. Coleman, Chem. Commun., 2011, 47, 7303 RSC.
- E. Masson, X. Ling, R. Joseph, L. Kyeremeh-Mensah and X. Lu, RSC Adv., 2012, 2, 1213 RSC.
- S. J. Barrow, S. Kasera, M. J. Rowland, J. del Barrio and O. A. Scherman, Chem. Rev., 2015, 115, 12320 CrossRef CAS PubMed.
- K. I. Assaf and W. M. Nau, Chem. Soc. Rev., 2015, 44, 394 RSC.
- L. Cao, M. Šekutor, P. Y. Zavalij, K. Mlinarić-Majerski, R. Glaser and L. Isaacs, Angew. Chem., Int. Ed., 2014, 53, 988 CrossRef CAS PubMed.
- D. H. Macartney, Isr. J. Chem., 2011, 51, 600 CrossRef CAS.
- I. Ghosh and W. M. Nau, Adv. Drug Delivery, 2012, 64, 764 CrossRef CAS PubMed.
- D. H. Macartney, Future Med. Chem., 2013, 5, 2075 CrossRef CAS PubMed.
- K. I. Kuok, S. Li, I. W. Wyman and R. Wang, Ann. N. Y. Acad. Sci., 2017, 1398, 108 CrossRef CAS PubMed.
- G. Hettiarachchi, D. Nguyen, J. Wu, D. Lucas, L. Isaacs and V. Briken, PLoS One, 2010, 5, e10514 Search PubMed.
- V. D. Uzunova, C. Cullinane, K. Brix and W. M. Nau, Org. Biomol. Chem., 2010, 8, 2037 CAS.
- R. Oun, R. S. Floriano, L. Isaacs, E. G. Rowan and N. J. Wheate, Toxicol. Res., 2014, 3, 447 RSC.
- H. Chen, J. Y. Chan and X. Yang, RSC Adv., 2015, 5, 30067 RSC.
- H.-J. Kim, W. S. Jeon, Y. H. Ko and K. Kim, Proc. Natl. Acad. Sci. U. S. A., 2002, 99, 5007 CrossRef CAS PubMed.
- W. Ong and A. E. Kaifer, J. Org. Chem., 2004, 69, 1383 CrossRef CAS PubMed.
- Y. J. Jeon, S.-Y. Kim, Y. H. Ko, S. Sakamoto, K. Yamaguchi and K. Kim, Org. Biomol. Chem., 2005, 3, 2122 CAS.
- N. Barooah, A. Kunwar, R. Khurana, A.
C. Bhasikuttan and J. Mohanty, Chem.–Asian J., 2017, 12, 122 CrossRef CAS PubMed.
- T. Tian, Y. Song, L. Wei, J. Wang, B. Fu, Z. He, X.-R. Yang, F. Wu, G. Xu, S.-M. Liu, C. Li, S. Wang and X. Zhou, Nucleic Acids Res., 2017, 45, 2283 Search PubMed.
- I. W. Wyman and D. H. Macartney, Org. Biomol. Chem., 2008, 6, 1796 CAS.
- H. Tang, D. Fuentealba, Y. H. Ko, N. Selvapalam, K. Kim and C. Bohne, J. Am. Chem. Soc., 2011, 133, 20623 CrossRef CAS PubMed.
- A. Day, A. P. Arnold, R. J. Blanch and B. Snushall, J. Org. Chem., 2001, 66, 8094 CrossRef CAS PubMed.
- F. Yi, J. Gao, L. Zhang and X. Jiang, Asian J. Chem., 2015, 27, 1260 CrossRef CAS.
- M. A. Jermyn, Aust. J. Chem., 1967, 20, 183 CrossRef CAS.
- S. Liu, C. Ruspic, P. Mukhopadhyay, S. Chakrabarti, P. Y. Zavalij and L. Isaacs, J. Am. Chem. Soc., 2005, 127, 15959 CrossRef CAS PubMed.
- K. A. Connors, Binding Constants, Wiley-Interscience, Toronto, 1987 Search PubMed.
- J. C. Lewis, Anal. Biochem., 1966, 14, 495 CrossRef CAS PubMed.
- Y. Chen, L. Jing, X. Li and Y. Zhu, J. Chromatogr. A, 2006, 1118, 3 CrossRef CAS PubMed.
- Q. Liu, X. Li and S. S. Sommer, Anal. Biochem., 1999, 270, 112 CrossRef CAS PubMed.
- T. L. Esplin, M. L. Cable, H. B. Gray and A. Ponce, Inorg. Chem., 2010, 49, 4643 CrossRef CAS PubMed.
- L. Cao and L. Isaacs, Supramol. Chem., 2014, 26, 251 CrossRef CAS.
- W. Li and A. E. Kaifer, Organometallics, 2013, 32, 6091 CrossRef CAS.
- J.-S. Yu, F.-G. Wu, L.-F. Tao, J.-J. Luo and Z.-W. Yu, Phys. Chem. Chem. Phys., 2011, 13, 3638 RSC.
- I. W. Wyman and D. H. Macartney, Org. Biomol. Chem., 2010, 8, 253 CAS.
- M. A. Gamal-Eldin and D. H. Macartney, Org. Biomol. Chem., 2013, 11, 488 CAS.
- M. A. Gamal-Eldin and D. H. Macartney, Org. Biomol. Chem., 2013, 11, 1234 CAS.
- J. Wouters and D. Stalke, Acta Crystallogr., Sect. C: Cryst. Struct. Commun., 1966, 52, 1684 CrossRef.
- P. Śledź, T. Minor and M. Chruszcz, Acta Crystallogr., Sect. E: Struct. Rep. Online, 2009, 65, o3027 Search PubMed.
- P. Śledź, R. Kamiński, M. Chruszcz, M. D. Zimmerman, W. Minor and K. Woźniak, Acta Crystallogr., Sect. B: Struct. Sci., 2010, 66, 482 Search PubMed.
- C. Marquez and W. M. Nau, Angew. Chem., Int. Ed., 2011, 40, 3155 CrossRef.
- R. D. Long, N. P. Hilliard Jr, S. A. Chhatre, T. V. Timofeeva, A. A. Yakovenko, D. K. Dei and E. A. Mensay, Beilstein J. Org. Chem., 2010, 6, 31 Search PubMed.
- Z. Szakács, G. Hägelea and R. Tyka, Anal. Chim. Acta, 2004, 522, 247 CrossRef.
Footnote |
† Electronic supplementary information (ESI) available: 1H NMR spectra of guest and host–guest complexes, 1H NMR titration plots, and limiting chemical shift values. See DOI: 10.1039/c7ra08865e |
|
This journal is © The Royal Society of Chemistry 2017 |