DOI:
10.1039/C7RA04029F
(Paper)
RSC Adv., 2017,
7, 28556-28563
Coordination matrix/signal amplifier strategy for simultaneous electrochemical determination of cadmium(II), lead(II), copper(II), and mercury(II) ions based on polyfurfural film/multi-walled carbon nanotube modified electrode
Received
9th April 2017
, Accepted 15th May 2017
First published on 30th May 2017
Abstract
In this work, we firstly propose and confirm a novel coordination matrix/signal amplifier strategy to construct a highly sensitive lead(II) electrochemical sensor. Lead(II) ions can be efficiently accumulated and deposited on the electrode surface by strong coordination bonds between the unoccupied d-orbital of lead(II) ions and conjugated π-electron backbones of polyfurfural film (coordination matrix), and then the anodic stripping current can be significantly enhanced by multi-walled carbon nanotubes (MWCNTs, signal amplifier), finally realizing the highly sensitive determination of lead(II). The polyfurfural film/MWCNT modified glassy carbon electrode (GCE) sensor provided a wide linear detection range from 0.05 to 10 μg L−1 and a low detection limit of 0.01 μg L−1 (S/N = 3) for lead(II). Compared with a classical mercury film sensor (a classical and effective method for determining heavy metal ions), our proposed sensor was more sensitive and achieved better results. Moreover, based on the coordination matrix/signal amplifier strategy, the polyfurfural film/MWCNTs/GCE sensor was further successfully utilized for the simultaneous determination of Cd2+, Pb2+, Cu2+, and Hg2+, demonstrating a wide linear detection range for Cd2+ (0.5–15 μg L−1), Pb2+ (0.1–15 μg L−1), Cu2+ (0.1–12 μg L−1), and Hg2+ (1.5–12 μg L−1) and a low detection limit for Cd2+ (0.03 μg L−1, S/N = 3), Pb2+ (0.01 μg L−1, S/N = 3), Cu2+ (0.06 μg L−1, S/N = 3), and Hg2+ (0.1 μg L−1, S/N = 3). Finally, the proposed sensor was successfully applied to simultaneously determine Cd2+, Pb2+, Cu2+, and Hg2+ in real tap water samples. This work provides a novel and effective analytical strategy for constructing novel electrochemical sensors and shows broad application prospects in heavy metal ion determination for the future.
1. Introduction
Heavy metal ions, such as cadmium(II), lead(II), copper(II), and mercury(II), have become a severe threat to human health and environmental safety due to their toxicity.1,2 Heavy metal ions are non-biodegradable and can accumulate in the food chain, causing serious diseases in humans.3–5 Therefore, it is urgently necessary to develop a highly sensitive and rapid method for the determination of heavy metal ions.
In order to monitor the heavy metal ions contained in food and water samples, some typical analytical techniques such as atomic absorption spectrometry,6 inductively coupled plasma mass spectrometry,7 surface enhanced Raman spectrometry,8 and inductively coupled plasma atomic emission spectrometry9 have been applied for the determination of heavy metal ions; however, expensive instrumentation, high costs, and time-consuming determination processes limit their extensive practical application.10 Therefore, the development of a simple and rapid method for the determination of heavy metal ions is of vital importance. Differential pulse anodic stripping voltammetry (DPASV), in which metal ions are deposited on an electrode surface and then oxidized, possesses the advantages of high sensitivity, low cost, easy operation, a low detection limit, and the ability to simultaneously determine several metal ions; this has been widely considered as a powerful tool for the determination of heavy metal ions.11–13 Nowadays, different chemically modified electrodes have been developed for the quantitative determination of heavy metal ions due to their ability to capture metal ions based on their interaction with a functional group at the modified electrode surface.14–17
Polyfurfural film possesses long conjugated π-electron backbones,18 which can provide multiple π-electrons and can act as an excellent coordination matrix to capture heavy metal efficiently via strong coordination bonds between the unoccupied d-orbital of lead(II) ions and the conjugated π-electrons of the film. Multi-walled carbon nanotubes act as an electrochemical signal amplifier19–21 due to their excellent electrical conductivity, high surface area, and high chemical stability, and these have been widely used in electroanalytical chemistry. Taking advantage of the properties of polyfurfural film and MWCNTs, we developed a novel coordination matrix/signal amplifier strategy to construct an electrochemical sensor, and this strategy was also proven to be beneficial in this work. The polyfurfural film/MWCNTs/GCE sensor preparation is simple and all the parameters are fully controlled by electrochemistry; the polyfurfural film can be electropolymerized to the electrode surface via a one-step electropolymerization method.22–25 Moreover, our proposed sensor demonstrated a wide linear detection range and a low detection limit for lead(II), and it exhibited more sensitivity than a classical mercury film sensor (an effective method for determining heavy metal ions). Finally, based on the coordination matrix/signal amplifier strategy, the proposed sensor was successfully applied for the simultaneous determination of Cd2+, Pb2+, Cu2+, and Hg2+, demonstrated a wide linear detection range and a lower detection limit, and exhibited excellent selectivity, stability, and reproducibility. When the proposed sensor was applied to the quantitative determination of Cd2+, Pb2+, Cu2+, and Hg2+ in tap water samples, it gave a satisfactory result. This work may provide a good model for constructing a novel electrochemical sensor for highly sensitive determination of heavy metal ions in the future.
2. Experimental
2.1 Reagents
A 1000 μg mL−1 cadmium(II) standard solution containing 1.0 mol L−1 HNO3, a 1000 μg mL−1 lead(II) standard solution containing 1.0 mol L−1 HNO3, a 1000 μg mL−1 copper(II) standard solution containing 1.0 mol L−1 HNO3, a 1000 μg mL−1 mercury(II) standard solution containing 1.0 mol L−1 HNO3, and multi-walled carbon nanotubes (MWCNTs) were purchased from Aladdin Ltd (Shanghai, China). Furfural (99%, analytical grade) was obtained from J&K Chemical (Beijing, China). All other reagents were of analytical grade and all aqueous solutions were prepared with doubly distilled water. Acetate buffer solution (250 mL of 0.1 mol dm−3) was prepared by mixing solutions of acetic acid and sodium acetate then adjusting to the required pH with NaOH and HAc. The Pb impurities contained in NaAc (10 g, Pb: 10−8%) amount to 0.001 μg, so the concentration of Pb impurities contained in 250 mL of 0.1 M of acetate buffer solution was about 0.0008 ppb, and the sum of Pb impurities contained in HAc and NaOH was about 0.0024 ppb, which was less than the target of 0.05 ppb and could be neglected.
Cd2+, Pb2+, Cu2+, and Hg2+ test solutions: standard solutions of heavy metal ions were diluted to different concentrations by mixing with 0.1 mol dm−3 of acetate buffer solution (pH 4.0). Aliquots of 10 mL of test solution were used and a 3 mm diameter glassy carbon electrode was used in our experiment.
2.2 Fabrication of polyfurfural film/MWCNTs/GCE sensor
The preparation of the electrochemical sensor is shown in Scheme 1. Prior to surface modification, a bare GCE (3 mm diameter) was polished with 0.05 μm alumina powders, washed with absolute alcohol and doubly distilled water under ultrasonication, and finally dried in a nitrogen stream. A MWCNT aqueous solution (5 μL of 0.5 mg mL−1) was deposited on the electrode surface, which was dried under an infrared lamp, thus obtaining MWCNTs/GCE. The preparation of polyfurfural film was performed according to our research group's method.22–25 In this work, the furfural was electropolymerized to the surface of MWCNTs/GCE; the electropolymerization of furfural was performed in acetonitrile containing furfural (0.01 mol dm−3) and sodium perchlorate (0.06 mol dm−3) by cyclic voltammetry at a scan rate of 100 mV s−1 between −0.8 V and +2.8 V for 10 cycles. Finally, the polyfurfural film/MWCNTs/GCE sensor was obtained.
 |
| Scheme 1 Schematic representation of preparation of polyfurfural film/MWCNTs/GCE sensor. | |
2.3 Apparatus and method
Electrochemical impedance spectroscopy (EIS) and DPASV experiments were performed on a CHI 660E electrochemical workstation (Chenhua, Shanghai, China). A three-electrode configuration was used in all electrochemical experiments and consisted of the polyfurfural film/MWCNTs/GCE as working electrode, a platinum wire as the counter electrode, and a saturated calomel electrode (SCE) as reference electrode. Electrochemical impedance spectroscopy was performed with 5 mmol dm−3 of K3[Fe(CN)6]/K4[Fe(CN)6] solution containing 0.1 mol dm−3 of KCl under open circuit potential with a frequency range from 0.1 Hz to 100 kHz and 5 mV amplitude. For DPASV, the amplitude was 0.05 V, the pulse width was 0.05 s, the pulse period was 0.5 s, the sampling width was 0.0167, and the increment was 0.004 V. The electrochemical experimental parameters were the same in all experiments. The surface morphology was characterized using a field emission scanning electron microscope (FE-SEM; Zeiss Ultra 55, Germany).
3. Results and discussion
3.1 Characterization of polyfurfural film/MWCNTs/GCE sensor
Fig. 1 shows the EIS plots of different electrodes; a small resistance was observed at the bare GCE (curve a). When the MWCNTs were bound to the surface of the bare GCE, the resistance of the MWCNTs/GCE (curve b) decreased significantly due to the surface area of the modified electrode being drastically increased and MWCNTs possessing high electrical conductivity. However, when the polyfurfural film was electropolymerized to the surface of the MWCNTs/GCE, the resistance of the polyfurfural film/MWCNTs/GCE (curve c) increased significantly, indicating that the polyfurfural film had poor electrical conductivity.
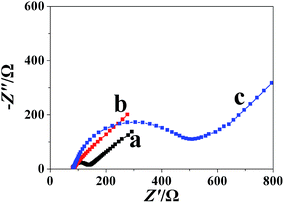 |
| Fig. 1 EIS of (a) bare GCE, (b) MWCNTs/GCE, and (c) polyfurfural film/MWCNTs/GCE in 5.0 mM K3[Fe(CN)6]/K4[Fe(CN)6] solution containing 0.1 M KCl. | |
The SEM image of the bare GCE shows a smooth surface (Fig. 2A). Multi-walled carbon nanotubes are easily observed on the surface of the electrode in Fig. 2B. When the furfural was electropolymerized to the surface of the MWCNTs/GCE, a clear view of the polymer layer with a rough surface was observed in Fig. 2C. It can be clearly observed that polyfurfural film covered the surface of MWCNTs, and the MWCNTs were buried under the polyfurfural film, which proved that Scheme 1 was successful. Therefore, both the EIS plots and SEM images provided confirmation that MWCNTs and polyfurfural film had been successfully bound to the electrode surface.
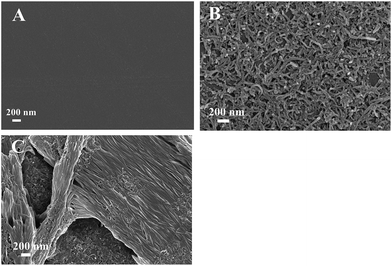 |
| Fig. 2 SEM images of (A) bare GCE, (B) MWCNTs/GCE and (C) polyfurfural film/MWCNTs/GCE. | |
3.2 The coordination matrix/signal amplifier strategy for electrochemical determination of Pb2+
The electrochemical responses to Pb2+ at a bare GCE, a MWCNTs/GCE, a polyfurfural film/GCE, and a polyfurfural film/MWCNTs/GCE were measured by DPASV. As shown in Fig. 3A, both the MWCNTs/GCE and polyfurfural film/GCE showed an enhanced anodic stripping current with Pb2+ compared to the bare GCE. Moreover, when we combined the polyfurfural film and MWCNTs to construct an electrochemical sensor, the anodic stripping current of Pb2+ at the polyfurfural film/MWCNTs/GCE was significantly further enhanced, and the signal was enhanced by about 2.5 times. Therefore, combining the advantages of polyfurfural film and MWCNTs can realize highly sensitive determination of Pb2+ with the best result. Moreover, as shown in Fig. 3A, compared with a classical mercury film sensor, our proposed sensor exhibited more sensitivity with respect to the determination of Pb2+.
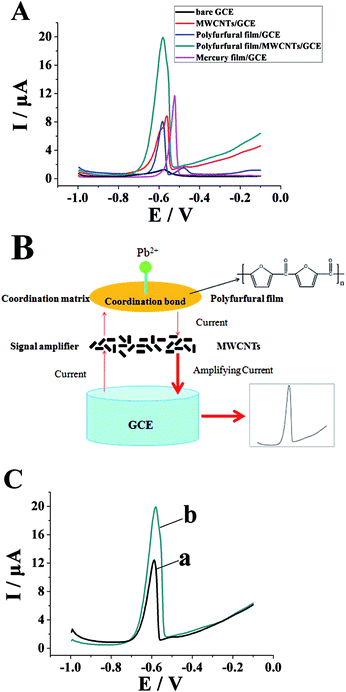 |
| Fig. 3 (A) DPASV curves for bare GCE, MWCNTs/GCE, polyfurfural film/GCE, polyfurfural film/MWCNTs/GCE, and mercury film/GCE in the presence of 10 μg L−1 Pb2+ in 0.1 M acetate buffer solution (pH 4.0). (B) Schematic representation of coordination matrix/signal amplifier strategy. (C) DPASV curves for (a) polyfurfural film/MWCNTs/GCE in the blank 0.1 M acetate buffer solution (pH 4.0) after immersion in 10 μg L−1 Pb2+ in 0.1 M acetate buffer solution (pH 4.0) at open circuit potential for 10 minutes, (b) polyfurfural film/MWCNTs/GCE in 10 μg L−1 Pb2+ in 0.1 M acetate buffer solution (pH 4.0). | |
In order to explain the superiority of the polyfurfural film/MWCNTs/GCE sensor for determination of Pb2+, and explain why the combination of polyfurfural film and MWCNTs can significantly enhance the anodic stripping current of Pb2+ compared with the polyfurfural film/GCE or MWCNTs/GCE alone, we proposed a novel coordination matrix/signal amplifier strategy to explain this interesting phenomenon. As shown in Fig. 3B, firstly, when the polyfurfural film/MWCNTs/GCE was immersed in 10 μg L−1 Pb2+ in 0.1 M acetate buffer solution (pH 4.0), lead(II) ions transferred to the surface of the polyfurfural film and then coordinated to it via strong coordination bonds between the unoccupied d-orbital of lead(II) ions and the conjugated π-electron backbones18,25–28 of polyfurfural film. Secondly, with a deposition potential of −1.2 V and a deposition time of 300 s, Pb2+ ions were reduced to Pb atoms, and polyfurfural film as coordination matrix provided multiple π-electrons, which was beneficial for the adherence of more Pb2+ ions. After the deposition step, Pb atoms were oxidized to Pb2+ ions, and the anodic stripping current of the Pb atoms firstly passed through the polyfurfural film and then through the MWCNTs; at that time, the anodic stripping current was amplified significantly, resulting an amplifying current returning to the electrode, which thus improved the sensitivity and significantly lowered the detection limit for Pb2+.
Subsequently, in order to confirm whether the coordination substance was Pb(II) or Pb(0), a control experiment was performed. Firstly, the polyfurfural film/MWCNTs/GCE was immersed in 10 μg L−1 Pb2+ in 0.1 M acetate buffer solution (pH 4.0) at open circuit potential for 10 minutes. Secondly, the modified electrode was rinsed with 0.1 M acetate buffer solution (pH 4.0) to remove physically adsorbed Pb(II), then the modified electrode was transferred to a Pb(II)-free solution in blank 0.1 M acetate buffer solution (pH 4.0). Finally, anodic stripping voltammetry was performed. As shown in Fig. 3C, a stripping peak for Pb(II) was observed with a peak current value of 11.19 μA (curve a), which means that Pb(II) ions are complexed and are not Pb(0). Moreover, when the anodic stripping voltammetry was performed in 10 μg L−1 Pb2+ in 0.1 M acetate buffer solution, the anodic stripping peak current was 18.47 μA (curve b). These results also demonstrate the strong coordination ability of polyfurfural film for Pb(II) ions.
3.3 The quantitative determination of Pb2+
As shown in Fig. 4A and B, the anodic stripping peak current of Pb2+ at the polyfurfural film/GCE increased linearly within the concentration range 0.5–10 μg L−1, and the linear regression equation was Ip (μA) = 0.86234c (μg L−1) − 0.12419 (R = 0.9973). The detection limit for Pb2+ was determined to be 0.1 μg L−1 (S/N = 3). In Fig. 4C and D, the anodic stripping peak current of Pb2+ at MWCNTs/GCE increased linearly within the concentration range 0.5–10 μg L−1, and the linear regression equation was Ip (μA) = 0.76992c (μg L−1) + 0.36522 (R = 0.9979) with a detection limit of 0.1 μg L−1 (S/N = 3). Benefitting from the superiority of the coordination matrix/signal amplifier strategy, which can realize the highly sensitive determination of lead(II), the polyfurfural film/MWCNTs/GCE sensor was applied to the quantitative determination of Pb2+ by DPASV. As shown in Fig. 4E and F, the anodic stripping peak current of Pb2+ at polyfurfural film/MWCNTs/GCE increased linearly within the concentration range 0.05–10 μg L−1, and the linear regression equation was Ip (μA) = 1.85169c (μg L−1) + 0.10536 (R = 0.9983). The detection limit for Pb2+ was determined to be 0.01 μg L−1 (S/N = 3). Moreover, as shown in Table 1, our proposed sensor showed a lower detection limit compared with other reported electrochemical sensors29–33 for the determination of Pb2+, and our proposed sensor preparation is simple and all parameters are fully controlled by electrochemistry, where polyfurfural film can electropolymerize to the electrode surface via a one-step electropolymerization method. These results indicated that the coordination matrix/signal amplifier strategy is an effective analytical strategy for highly sensitive determination of Pb2+.
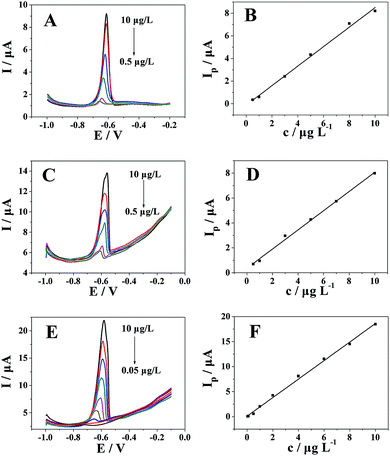 |
| Fig. 4 DPASV curves of Pb2+ at different concentrations at the (A) polyfurfural film/GCE, (C) MWCNTs/GCE, and (E) polyfurfural film/MWCNTs/GCE in 0.1 M acetate buffer solution (pH 4.0). Linear relationship between anodic stripping peak current and concentration at (B) polyfurfural film/GCE, (D) MWCNTs/GCE, and (F) polyfurfural film/MWCNTs/GCE. | |
Table 1 Comparison of the performance of the polyfurfural film/MWCNTs/GCE with those of other modified electrodes
Electrode |
Linear range (μg L−1) |
Limit of detection (μg L−1) |
Reference |
L-Cys-rGO/GCE |
83.0–250 |
0.416 |
29 |
SBA-15/CPE |
62.0–1450 |
8.3 |
30 |
PPh3/MWCNTs/IL/CPE |
0.02–31.0 |
0.012 |
31 |
N-Doped graphene/GCE |
2.0–1865 |
1.0 |
32 |
Mercury film/N/IL/Electrode |
1.0–16.0 |
0.12 |
33 |
Polyfurfural film/MWCNTs/GCE |
0.05–10 |
0.01 |
This work |
3.4 Simultaneous electrochemical determination of Cd2+, Pb2+, Cu2+, and Hg2+ with the polyfurfural film/MWCNTs/GCE sensor
The polyfurfural film/MWCNTs/GCE sensor was further utilized for simultaneous determination of Cd2+, Pb2+, Cu2+, and Hg2+, as shown in Fig. 5; compared with bare GCE, both the polyfurfural film/GCE and MWCNTs/GCE showed obviously increased anodic stripping currents with Cd2+, Pb2+, Cu2+, and Hg2+. However, when we combined the polyfurfural film and MWCNTs, the anodic stripping current at polyfurfural film/MWCNTs/GCE was significantly further enhanced. Therefore, based on the coordination matrix/signal amplifier strategy, the polyfurfural film/MWCNTs/GCE sensor can realize the simultaneous determination of Cd2+, Pb2+, Cu2+, and Hg2+ with better results.
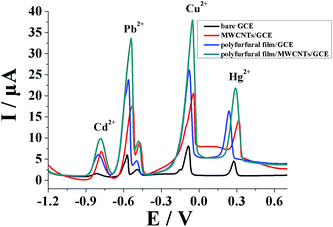 |
| Fig. 5 DPASV curves for bare GCE, MWCNTs/GCE, polyfurfural film/GCE, and polyfurfural film/MWCNTs/GCE in coexistent 10 μg L−1 Cd2+, 10 μg L−1 Pb2+, 10 μg L−1 Cu2+, and 10 μg L−1 Hg2+ in 0.1 M acetate buffer solution (pH 4.0). Electropolymerization cycles of furfural: 10 cycles, pH: 4.0, deposition potential: −1.2 V, deposition time: 300 s. | |
3.5 Optimization of number of electropolymerization cycles of furfural and pH
The effects of the number of electropolymerization cycles of furfural and pH on the electrochemical response to heavy metal ions in acetate buffer solution have been investigated by DPASV. As shown in Fig. 6A, in 0.1 M acetate buffer solution (pH 4.0), all the anodic stripping peak currents increased with the number of electropolymerization cycles of furfural from 3 to 10 cycles, but above 10 cycles, all the stripping peak currents decreased as the number of electropolymerization cycles of furfural increased. Therefore, 10 cycles have been chosen as the optimized condition in subsequent experiments.
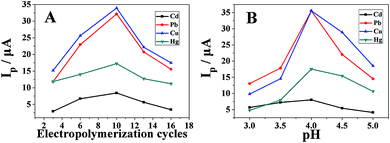 |
| Fig. 6 DPASV carried out on the polyfurfural film/MWCNTs/GCE with coexistent 10 μg L−1 Cd2+, 10 μg L−1 Pb2+, 10 μg L−1 Cu2+, and 10 μg L−1 Hg2+ in 0.1 M acetate buffer solution with different numbers of electropolymerization cycles of furfural (A) and different pH values (B) (deposition potential: −1.2 V, deposition time: 300 s). | |
In Fig. 6B, all the anodic stripping peak currents of heavy metal ions increased with pH and reached a maximum value at pH 4.0. However, above pH 4.0, all the anodic stripping peak currents gradually decreased. Therefore, a pH of 4.0 was chosen in later experiments.
3.6 Optimization of deposition potential and deposition time
The effects of deposition potential and deposition time on the anodic stripping peak currents of heavy metal ions in acetate buffer solution (pH 4.0) have also been investigated by DPASV. As shown in Fig. 7A, at a deposition time of 300 s, all the anodic stripping peak currents reached a maximum value at a deposition potential of −1.2 V. Above −1.2 V, all the anodic stripping peak currents decreased as the deposition potential increased. Therefore, a deposition potential of −1.2 V was chosen as the best condition for the simultaneous determination of metal ions in subsequent experiments.
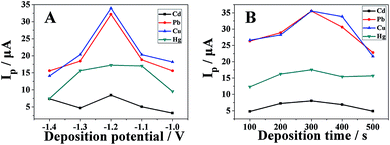 |
| Fig. 7 DPASV carried out on the polyfurfural film/MWCNTs/GCE in coexistent 10 μg L−1 Cd2+, 10 μg L−1 Pb2+, 10 μg L−1 Cu2+, and 10 μg L−1 Hg2+ in 0.1 M acetate buffer solution with different deposition potentials (A) and different deposition times (B) (electropolymerization cycles of furfural: 10 cycles, pH: 4.0). | |
As shown in Fig. 7B, at a deposition potential of −1.2 V, all the anodic stripping peak currents gradually increased with the deposition time and reached a maximum value at a deposition time of 300 s. Above 300 s, all the anodic stripping peak currents gradually decreased. Therefore, a deposition time of 300 s was chosen for simultaneous determination of metal ions in subsequent experiments.
3.7 Simultaneous quantitative determination of Cd2+, Pb2+, Cu2+, and Hg2+ with the polyfurfural film/MWCNTs/GCE sensor
The simultaneous quantitative determination of Cd2+, Pb2+, Cu2+, and Hg2+ with the polyfurfural film/MWCNTs/GCE sensor was measured by DPASV. Fig. 8A shows DPASV observed at different concentrations of Cd2+ in 0.1 mol L−1 acetate buffer solution (pH 4.0) in the presence of 0.5 μg L−1 Pb2+, 0.5 μg L−1 Cu2+, and 1 μg L−1 Hg2+. The result showed that the anodic stripping peak current of Cd2+ exhibits linearity with the concentration of Cd2+, while the anodic stripping peak currents of Pb2+, Cu2+, and Hg2+ remained almost constant, indicating that the anodic stripping of four metal ions at the polyfurfural film/MWCNTs/GCE took place independently. The linear ranges of Cd2+ were 0.5–5.5 μg L−1 and 5.5–15 μg L−1, and the linear regression equations were Ip (μA) = 3.88619c (μg L−1) − 1.21987 (R = 0.9985) and Ip (μA) = 1.14175c (μg L−1) + 13.57854 (R = 0.9988), respectively, with a detection limit of 0.03 μg L−1 (S/N = 3). Fig. 8B shows DPASV observed at different concentrations of Pb2+ in the presence of 1 μg L−1 Cd2+, 1 μg L−1 Cu2+, and 1 μg L−1 Hg2+. The anodic stripping peak current of Pb2+ increased linearly with concentration ranges of 0.1–5 μg L−1 and 5–15 μg L−1, and the linear regression equations were Ip (μA) = 4.07191c (μg L−1) − 0.57489 (R = 0.9988) and Ip (μA) = 1.70164c (μg L−1) + 11.50792 (R = 0.9992), respectively. The detection limit for Pb2+ was 0.01 μg L−1 (S/N = 3). Fig. 8C shows DPASV obtained from different concentrations of Cu2+ in the presence of 1 μg L−1 Cd2+, 1 μg L−1 Pb2+, and 1 μg L−1 Hg2+. The linear ranges of Cu2+ were 0.1–3 μg L−1 and 3–12 μg L−1, and the linear regression equations were Ip (μA) = 4.90678c (μg L−1) − 0.32969 (R = 0.9980) and Ip (μA) = 1.59947c (μg L−1) + 9.33 (R = 0.9979), respectively, with a detection limit of 0.06 μg L−1 (S/N = 3). Fig. 8D shows DPASV observed at different concentrations of Hg2+ in the presence of 1 μg L−1 Cd2+, 0.5 μg L−1 Pb2+, and 0.5 μg L−1 Cu2+. The anodic stripping peak current of Hg2+ increased linearly with concentration ranges of 1.5–6 μg L−1 and 6–12 μg L−1, and the linear regression equations can be expressed as Ip (μA) = 1.56593c (μg L−1) − 1.57058 (R = 0.9990) and Ip (μA) = 0.3714c (μg L−1) + 5.4804 (R = 0.9999), respectively. The detection limit for Hg2+ was 0.1 μg L−1 (S/N = 3). Moreover, the detection limit of our proposed sensor was comparable and even better than those of other previously reported electrochemical sensors29–33 for simultaneous determination of heavy metal ions, as shown in Table 2, indicating that the polyfurfural film/MWCNTs/GCE sensor is an appropriate platform for the simultaneous determination of these heavy metal ions.
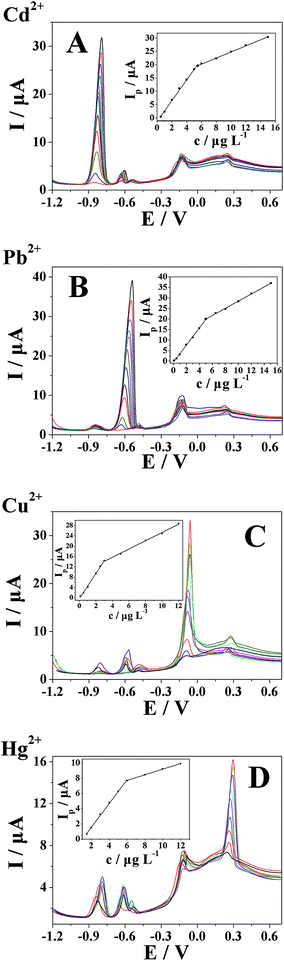 |
| Fig. 8 (A) DPASV of Cd2+ at different concentrations in 0.1 mol L−1 acetate buffer solution (pH 4.0) in the presence of 0.5 μg L−1 Pb2+, 0.5 μg L−1 Cu2+, and 1 μg L−1 Hg2+ at the polyfurfural film/MWCNTs/GCE. (B) DPASV of Pb2+ at different concentrations in the presence of 1 μg L−1 Cd2+, 1 μg L−1 Cu2+, and 1 μg L−1 Hg2+ at the polyfurfural film/MWCNTs/GCE. (C) DPASV of Cu2+ at different concentrations in the presence of 1 μg L−1 Cd2+, 1 μg L−1 Pb2+, and 1 μg L−1 Hg2+ at the polyfurfural film/MWCNTs/GCE. (D) DPASV of Hg2+ at different concentrations in the presence of 1 μg L−1 Cd2+, 0.5 μg L−1 Pb2+, and 0.5 μg L−1 Cu2+ at the polyfurfural film/MWCNTs/GCE. Insets: linear relationships between anodic stripping peak current and concentration. | |
Table 2 Comparison of the performance of the polyfurfural film/MWCNTs/GCE with those of other modified electrodes
Electrode |
Method |
Linear range (μg L−1) |
Limit of detection (μg L−1) |
Reference |
L-Cys-rGO/GCE |
DPASV |
Cd2+, 45.0–225 |
0.366 |
29 |
Pb2+, 83.0–249 |
0.416 |
Cu2+, 25.0–127 |
0.261 |
Hg2+, 80.0–401 |
1.113 |
SBA-15/CPE |
DPASV |
Pb2+, 62–1450 |
8.3 |
30 |
Cu2+, 51–635.5 |
12.7 |
Hg2+, 401–2006 |
80.2 |
PPh3/MWCNTs/IL/CPE |
OSWSV |
Cd2+, 0.01–17 |
0.008 |
31 |
Pb2+, 0.02–31 |
0.012 |
Hg2+, 0.02–30 |
0.018 |
N-Doped graphene/GCE |
DPASV |
Cd2+, 5.6–1012 |
5.6 |
32 |
Pb2+, 2.0–1865 |
1.0 |
Cu2+, 0.64–318 |
0.32 |
Hg2+, 40–1805 |
10.0 |
Mercury film/N/IL/Electrode |
AdSV |
Cd2+, 0.1–16.0 |
0.13 |
33 |
Pb2+, 1.0–16.0 |
0.12 |
Polyfurfural film/MWCNTs/GCE |
DPASV |
Cd2+, 0.5–15 |
0.03 |
This work |
Pb2+, 0.1–15 |
0.01 |
Cu2+, 0.1–12 |
0.06 |
Hg2+, 1.5–12 |
0.1 |
3.8 Selectivity, reproducibility, and stability of the polyfurfural film/MWCNTs/GCE sensor
As shown in Table 3, the selectivity of the proposed sensor was estimated by adding 10-fold excesses of various possible interfering metal ions such as Na+, Ca2+, K+, Mg2+, Al3+, Ni2+, Co2+, and Mn2+ into standard solutions containing 10 μg L−1 Cd2+, 10 μg L−1 Pb2+, 10 μg L−1 Cu2+, and 10 μg L−1 Hg2+; the results showed that the effects of various interfering metal ions on the simultaneous determination of Cd2+, Pb2+, Cu2+, and Hg2+ were negligible (signal change below 5%), which demonstrated the excellent selectivity of the proposed sensor for simultaneous determination of Cd2+, Pb2+, Cu2+, and Hg2+.
Table 3 Effect of interfering metal ions on the anodic stripping peak currents
Interference |
Concentration (μg L−1) |
Signal changes (%) |
Cd2+ |
Pb2+ |
Cu2+ |
Hg2+ |
Na+ |
100 |
−2.54 |
0.50 |
1.51 |
1.85 |
Ca2+ |
100 |
1.81 |
−1.10 |
−3.21 |
2.03 |
K+ |
100 |
1.57 |
0.22 |
0.55 |
3.02 |
Mg2+ |
100 |
3.36 |
1.50 |
1.87 |
2.19 |
Al3+ |
100 |
2.87 |
−3.25 |
−4.56 |
3.61 |
Ni2+ |
100 |
4.43 |
4.05 |
3.57 |
−2.87 |
Co2+ |
100 |
4.59 |
2.20 |
3.82 |
4.12 |
Mn2+ |
100 |
−3.21 |
−3.80 |
4.55 |
3.97 |
The reproducibility of the proposed sensor was evaluated with six modified electrodes that were fabricated under the same conditions. The values of the relative standard deviation (RSD) in the determination were 2.71% for Cd2+, 2.60% for Pb2+, 1.62% for Cu2+, and 2.53% for Hg2+, suggesting that the proposed sensor possesses high reproducibility. The stability of the proposed sensor was measured after 1 month, and the anodic stripping peak currents of the four metal ions retained 98.97% for Cd2+, 92.80% for Pb2+, 92.20% for Cu2+, and 98.29% for Hg2+ of their initial values, which suggested that the proposed sensor possesses excellent stability.
3.9 Analytical application for real tap water samples
In order to evaluate the analytical application potential of the polyfurfural film/MWCNTs/GCE sensor for simultaneous determination of Cd2+, Pb2+, Cu2+, and Hg2+ in real samples, tap water samples were used for quantitative analysis using a standard-addition technique, and three parallel experiments were performed for all measurements; Cd2+, Pb2+, Cu2+, and Hg2+ standard solutions were added to tap water. The initial contents of Cd2+, Pb2+, Cu2+, and Hg2+ in the tap water samples were determined with an inductively coupled plasma emission spectrometer (ICP). As shown in Table 4, the average recoveries range from 96.50% to 101.43% for Cd2+, 97.51% to 103.98% for Pb2+, 98.01% to 100.40% for Cu2+, and 100.00% to 101.60% for Hg2+, suggesting that the proposed sensor can be efficiently applied to the simultaneous determination of Cd2+, Pb2+, Cu2+, and Hg2+ in real samples.
Table 4 Determination of four heavy metal ions in tap water samples (n = 3)
Analyte |
Initial content (μg L−1) |
Added (μg L−1) |
Founda (μg L−1) |
Recovery (%) |
RSD (%) |
Sample responses are expressed as a confidence interval with 95% probability. |
Cd2+ |
— |
10 |
9.96 ± 0.21 |
99.60 |
0.85 |
— |
7 |
7.10 ± 0.62 |
101.43 |
3.52 |
— |
4 |
4.05 ± 0.17 |
101.25 |
1.69 |
— |
2 |
1.93 ± 0.10 |
96.50 |
2.09 |
Pb2+ |
0.01 |
10 |
10.01 ± 1.34 |
100.00 |
5.39 |
0.01 |
7 |
6.94 ± 0.47 |
99.00 |
2.73 |
0.01 |
4 |
3.91 ± 0.19 |
97.51 |
1.96 |
0.01 |
2 |
2.09 ± 0.06 |
103.98 |
1.16 |
Cu2+ |
0.01 |
10 |
10.05 ± 0.50 |
100.40 |
2.00 |
0.01 |
7 |
7.00 ± 0.39 |
99.86 |
2.24 |
0.01 |
4 |
4.00 ± 0.58 |
99.75 |
5.84 |
0.01 |
2 |
1.97 ± 0.09 |
98.01 |
1.84 |
Hg2+ |
— |
10 |
10.16 ± 0.42 |
101.60 |
1.67 |
— |
7 |
7.06 ± 0.16 |
100.86 |
0.91 |
— |
4 |
4.02 ± 0.28 |
100.50 |
2.81 |
— |
2 |
2.00 ± 0.07 |
100.00 |
1.41 |
4. Conclusions
In summary, we proposed and confirmed a novel coordination matrix/signal amplifier strategy to construct a highly sensitive lead(II) electrochemical sensor. The polyfurfural film/MWCNTs/GCE sensor had a wider linear range (0.05–10 μg L−1) and a lower detection limit (0.01 μg L−1, S/N = 3) for lead(II). Compared with a classical mercury film sensor, our proposed sensor exhibited greater sensitivity and achieved better results. Benefitting from the coordination matrix/signal amplifier strategy, the proposed sensor was successfully applied to the simultaneous quantitative determination of cadmium(II), lead(II), copper(II), and mercury(II) ions in real tap water samples. Moreover, the proposed sensor exhibited better selectivity, stability, and reproducibility and also had wide linear ranges for Cd2+ (0.5–15 μg L−1), Pb2+ (0.1–15 μg L−1), Cu2+ (0.1–12 μg L−1), and Hg2+ (1.5–12 μg L−1) with low detection limits for Cd2+ (0.03 μg L−1, S/N = 3), Pb2+ (0.01 μg L−1, S/N = 3), Cu2+ (0.06 μg L−1, S/N = 3), and Hg2+ (0.1 μg L−1, S/N = 3). This work provides a novel and effective analytical strategy for highly sensitive determination of heavy metal ions in the future.
Acknowledgements
Financial support from the National Natural Science Foundation of China (Grant No. 21475046, 21427809) and the Fundamental Research Funds for the Central Universities (No. 2015ZP028) is gratefully acknowledged.
References
- M. J. Melgar, B. Miguez, M. Perez, M. A. Garcia, M. I. Fernandez and M. Vidal, J. Environ. Sci. Health, Part A: Environ. Sci. Eng. Toxic Hazard. Subst. Control, 1997, 32, 687–697 CrossRef.
- N. R. Ekere, P. O. Ukoha, U. V. Udeogu, J. N. Ihedioha and V. E. Agbazue, Hum. Ecol. Risk Assess., 2016, 22, 393–400 CrossRef CAS.
- R. K. Sharma and M. Agrawal, J. Environ. Biol., 2005, 26, 301–313 CAS.
- L. C. Acosta-Saavedra, M. E. Moreno, T. Rodriguez-Kessler, A. Luna, D. Arias-Salvatierra, R. Gomez and E. S. Calderon-Aranda, Toxicol. Mech. Methods, 2011, 21, 656–666 CrossRef CAS PubMed.
- C. H. Jagoe, C. E. Dallas, R. K. Chesser, M. H. Smith, S. K. Lingenfelser, J. T. Lingenfelser, K. Holloman and M. Lomakin, Ecotoxicology, 1998, 7, 201–209 CrossRef CAS.
- E. L. Silva and P. D. Roldan, J. Hazard. Mater., 2009, 161, 142–147 CrossRef CAS PubMed.
- A. A. Ammann, Anal. Bioanal. Chem., 2002, 372, 448–452 CrossRef CAS PubMed.
- Y. M. Ma, H. L. Liu, K. Qian, L. B. Yang and J. H. Liu, J. Colloid Interface Sci., 2012, 386, 451–455 CrossRef CAS PubMed.
- K. S. Rao, T. Balaji, T. P. Rao, Y. Babu and G. R. Naidu, Spectrochim. Acta, Part B, 2002, 57, 1333–1338 CrossRef.
- K. M. Zeinu, H. Hou, B. Liu, X. Yuan, L. Huang, X. Zhu, J. Hu, J. Yang, S. Liang and X. Wu, J. Mater. Chem. A, 2016, 4, 13967–13979 CAS.
- H. Lin, M. X. Li and D. Mihailovic, Electrochim. Acta, 2015, 154, 184–189 CrossRef CAS.
- O. El Tall, N. Jaffrezic-Renault, M. Sigaud and O. Vittori, Electroanalysis, 2007, 19, 1152–1159 CrossRef CAS.
- G. M. S. Alves, J. Magalhaes, P. Salaun, C. M. G. Berg and H. Soares, Anal. Chim. Acta, 2011, 703, 1–7 CrossRef CAS PubMed.
- I. Cesarino, E. T. G. Cavalheiro and C. M. A. Brett, Electroanalysis, 2010, 22, 61–68 CrossRef CAS.
- V. Jovanovski, N. I. Hrastnik and S. B. Hočevar, Electrochem. Commun., 2015, 57, 1–4 CrossRef CAS.
- S. Chaiyo, E. Mehmeti, K. Zagar, W. Siangproh, O. Chailapakul and K. Kalcher, Anal. Chim. Acta, 2016, 918, 26–34 CrossRef CAS PubMed.
- D. Omanović, C. Garnier, K. Gibbon–Walsh and I. Pižeta, Electrochem. Commun., 2015, 61, 78–83 CrossRef.
- A. M. S. Lucho, J. L. J. Hallal and R. S. Goncalves, J. Macromol. Sci., Part A: Pure Appl.Chem., 2003, 40, 933–946 CrossRef.
- Y. S. Fang, H. Y. Wang, L. S. Wang and J. F. Wang, Biosens. Bioelectron., 2014, 51, 310–316 CrossRef CAS PubMed.
- Y. S. Fang, X. J. Huang, L. S. Wang and J. F. Wang, Biosens. Bioelectron., 2015, 64, 324–332 CrossRef CAS PubMed.
- R. Akter, M. A. Rahman and C. K. Rhee, Anal. Chem., 2012, 84, 6407–6415 CrossRef CAS PubMed.
- Y. Fu, Y. Lin, T. Chen and L. Wang, J. Electroanal. Chem., 2012, 687, 25–29 CrossRef CAS.
- T. Wei, X. Huang, Q. Zeng and L. Wang, J. Electroanal. Chem., 2015, 743, 105–111 CrossRef CAS.
- Q. Zeng, T. Wei, M. Wang, X. Huang, Y. Fang and L. Wang, Electrochim. Acta, 2015, 186, 465–470 CrossRef CAS.
- J. Huang, X. Shen, Q. Hu, Y. Ma, S. Bai, G. Yue, X. Yu, Q. Zeng and L. Wang, RSC Adv., 2016, 6, 95435–95441 RSC.
- A. C. Ferrari and J. Robertson, Phys. Rev. B: Condens. Matter Mater. Phys., 2000, 61, 14095–14107 CrossRef CAS.
- C. E. Banks and R. G. Compton, Analyst, 2005, 130, 1232–1239 RSC.
- C. E. Banks, R. R. Moore, T. J. Davies and R. G. Compton, Chem. Commun., 2004, 1, 1804–1805 RSC.
- S. Muralikrishna, K. Sureshkumar, T. S. Varley, D. H. Nagaraju and T. Ramakrishnappa, Anal. Methods, 2014, 6, 8698–8705 RSC.
- I. Cesarino, G. Marino, J. D. Matos and E. T. G. Cavalheiro, Talanta, 2008, 75, 15–21 CrossRef CAS PubMed.
- H. Bagheri, A. Afkhami, H. Khoshsafar, M. Rezaei and A. Shirzadmehr, Sens. Actuators, B, 2013, 186, 451–460 CrossRef CAS.
- H. K. Xing, J. K. Xu, X. F. Zhu, X. M. Duan, L. M. Lu, W. M. Wang, Y. S. Zhang and T. T. Yang, J. Electroanal. Chem., 2016, 760, 52–58 CrossRef CAS.
- E. Nagles, V. Arancibia, R. Rios and C. Rojas, Int. J. Electrochem. Sci., 2012, 7, 5521–5533 CAS.
|
This journal is © The Royal Society of Chemistry 2017 |