DOI:
10.1039/C7RA02876H
(Paper)
RSC Adv., 2017,
7, 23530-23534
Phase change materials of fatty amine-modified silicate clays of nano layered structures
Received
9th March 2017
, Accepted 19th April 2017
First published on 28th April 2017
Abstract
Fatty-amine modified silicate clays of layered structure were prepared by conventional intercalation of n-octadecyl amine (n-C18H37NH2, ODA) into sodium montmorillonite clay (Na+-MMT). Organically modified clays are considered as a new type of phase change materials (PCM). At one equivalent ratio of ODA-HCl over Na+-MMT by an ionic exchange reaction, the interlayer galleries of the silicates' layered structure were expanded from 12 Å to 28 Å as measured by X-ray diffraction (XRD) analysis. As a result, the organoclay (ODA/MMT) became hydrophobic and spacing was accessible for incorporating a second organic compound, n-octadecane. Physical absorption of n-octadecane in ODA/MMT allowed interlayer spacing and further expansion from 28 Å to 30 Å. Absorption efficiency, defined as the weight of n-octadecane over ODA/MMT, ranged from 5.3 to 9.1 fold depending on the amount of ODA intercalation. Since n-octadecane is a kind of paraffin wax, a phase change temperature of 25–29 °C was observed. The n-octadecane was characterized by thermal gravimetric analysis (TGA) to study absorption efficiency of ODA/MMT. During the heating process, the ODA/MMT/n-octadecane powder remained in solid state without any liquid leakage at 80 °C (far beyond the phase change temperature). Thus, the organoclay (ODA/MMT) can be used as good thermal storage and phase change material.
Introduction
Storage of thermal energy has attracted a great deal of interest due to its many applications.1 Thermal energy can be confined and stored in different forms of internal energy as sensible heat, latent heat, thermochemical bonds, etc. Among them, latent heat storage is most attractive due to its high storage density and temperature sensitive variation between storage and retrieval. Requirements for suitable latent heat storage are its melting points, which must be close to the practical operation temperature, and stabilities in physical and chemical properties. Among various materials used for latent heat storage, phase change materials (PCM) have potential for practical applications for utilizing solar energy, recovering industrial waste heat, and shifting electrical power loads.
PCM includes classes of organic and inorganic substances,2–4 and both have general chemical and thermal stability against phase segregation and physical deterioration. Organic substances such as paraffin consist of a mixture of n-alkanes which are generally crystalline and can absorb energy. Besides paraffins, non-paraffin materials such as esters,5 fatty-acids,6 alcohols, and glycols7 have high heats of fusion but low thermal conductivity, inflammability, toxicity, and instability at high temperatures. Inorganic PCMs4,8 include salt hydrates,9 salts, metals, and alloys. They usually have low cost, high density energy storage, and high thermal conductivity. However, paraffins are popularly used for wide range of latent heat fusion from 170 kJ kg−1 to 270 kJ kg−1 between a range of 5 °C to 80 °C which is suitable for building insulation and solar applications.4
For solid–liquid phase change materials, encapsulation of paraffin is the important issue since it relates to stability of the composites during application. Common materials for encapsulating or supporting paraffins are polymers such as polymethylmethacrylate,10 polyethylene oxide,11 and high density polyethylene.12,13 However, uses of supporting polymers could generate problems of flammability and low thermal stability. Other examples, such as silica nanoparticles (nano-SiO2), were demonstrated as additives to lauric acid/PET to form shape-stable PCM.14 The role of nano-SiO2 was as a modifier for improving material properties such as large surface area, high strength, good heat stability, and good chemical resistance.
Naturally occurring clays with a layered structure generally existed in an aggregated form with 2D layered stacks having an average of 8–10 sheets with dimensions of 100 × 100 × 1 nm3 for each sheet. In our previous works,15,16 the 2D primary structure rendered silicates having a high specific surface area, high strength, good heat stability, and good chemical resistance. In addition, consider the uniqueness of its exchangeable sodium ions at a value of 1.20 mequiv. g−1 for cationic exchange capacity (CEC); for example, sodium montmorillonite (Na+-MMT) is noticeable for its encapsulating ability. It is allowable for the clay to be organically modified by an ionic exchanging reaction to adjust interlayer galleries in its nanostructure. Intercalating amine-salts could enlarge basal spacing of the clay and make it accessible for absorbing other hydrophobic organics17,18 That hydrophobic modification made the modified MMT capable of absorbing crude oils19,20 for an application of remedying oil pollution in water.
In continuing our previous works with clay intercalation and attempts for new applications of layered silicates, here we report the approach of utilizing the organoclay, n-octadecyl amine (ODA) intercalated MMT, to absorb n-octadecane as the phase change material for energy storage. Paraffin encapsulated clays are proposed for PCM uses with advantages of non-flammability, low toxicity, and thermal stability. The clay spacing expansion, paraffin absorption efficiency, and thermal properties were characterized by X-ray diffraction, thermal gravimetric analysis, and differential scanning calorimetry.
Experimental section
Materials
Sodium montmorillonite (Na+-MMT) (Nanocor Co., USA) is one of the phyllosilicates from natural minerals which have the generic structure of tetrahedral and octahedral aluminosilicate sheets with a 2
:
1 ratio. Each primary stack has an average number of 8–10 sheets which are plate-like shapes in stacks with a dimension of approximately 100 × 100 × 1 nm3. This clay of layered structure was estimated to have an ionic character with a cationic exchange capacity (CEC) of 1.20 mequiv. g−1, indicating a capacity for an organic salt exchange reaction to replace the counter metal ions of Na+ in the clay. The n-octadecylamine (ODA) and n-octadecane were both purchased from Alfa Aesar (USA).
Preparation of organoclays from n-octadecylamine intercalation with MMT
The organoclay (ODA/MMT) was prepared from the intercalation of Na+-MMT and n-octadecylamine (ODA)/HCl salt via an ionic exchange reaction. In typical procedures, Na+-MMT (1.0 g, 1.2 mequiv. g−1) was swelled in deionized water (100 mL) at 80 °C for three hours. ODA (0.28 g, 1.2 mmol) was dissolved in ethanol and treated with one equivalent of hydrochloric acid (35%, 0.13 g, 1.2 mmol) to generate –NH3+Cl− salts. The salts were added into the MMT slurry at an amount of one equivalent to the clay's CEC, while the mixture was vigorously mixed by mechanical stirring at 80 °C for 0.5 h. The resultant precipitates were filtered, then washed with deionized water several times, and dried at 50 °C for 24 h.
Preparation of the second-step interaction for phase change materials
A three-necked round-bottomed flask (100 mL) equipped with a mechanical stirrer, a reflux condenser, and a thermometer, was charged with ODA/MMT (0.5 g) in water (50 mL) at 80 °C. After stirring well and dispersing ODA/MMT for 0.5 h, the n-dodecane was added into the organoclay mixture with different amounts until reaching the maximum paraffin absorption efficiency (i.e., no further absorption). The mixture was stirred for 10 minutes, then washed with water using repetitive filtration and re-dispersion in the water.
Characterization
Measurements for basal spacings (d spacing) of the clays were performed by X-ray powder diffraction (XRD, Schimadzu, Japan) using a SD-D1 diffractometer with Cu target (k = 1.5408 Å) at a generator voltage of 45 kV and current of 40 mA. The d spacing was calculated according to the Bragg's equation (nλ = 2d
sin
θ), where the value for n = 1 was assigned by calculating the observed peak pattern for fitting n = 2, 3, or 4. The organic fraction was analyzed with thermal gravimetric analysis (TGA, PerkinElmer, USA) using a Pyris 1 model and observing the weight loss from the programming temperature increase from 100 °C to 800 °C at a rate of 10 °C min−1. Enthalpy of the materials was measured by differential scanning calorimetry (DSC, TA Instruments Q-20, USA), under temperature scanning between 0 °C and 60 °C with a temperature programming rate of 1 °C per minute.
Results and discussion
Preparation of organoclays by intercalation of n-octadecylamine
Naturally abundant minerals, layered silicate clays, were used to prepare the phase change materials (PCM), owing to their unique structure with a primary stack unit of multiple aluminosilicate sheets. The original stack unit consisted of an average of 8–10 sheets with d spacing of 12 Å (by X-ray analysis), while the thickness of each fundamental sheet was 1.0 nm having lateral dimension of 100 × 100 nm2. The silicate clays were estimated to have the value of cationic exchange capacity (CEC) of 1.20 mequiv. g−1, indicating the possible exchange of counter metal ions (sodium ions) with organic amine-salts. Specifically, the ionic exchange capacity of Na+ was allowed the equivalent amount of organic salts for clay intercalation. In this work, the organoclays were prepared by the intercalation of n-octadecylamine salts (C18H37NH3+Cl− or ODA salts) to convert the original inorganic clays into those with an organophilic nature. The organic modification afforded organoclays (ODA/MMT) with amphiphilic characteristics and made them useful for the second stage which incorporates hydrophobic organics into the clay's layered structures.
As shown in Table 1, the ODA salts were prepared from HCl treatment or acidification of ODA separately and then used to make the ionic exchange reactions with Na+-MMT at different CEC ratios. As a result, the d spacing of clay increased from 12 Å to 28 Å, 28 Å, 30 Å, and 34 Å, depending on the applied amount equivalence of amine-salts of 0.5 CEC, 1.0 CEC, 1.5 CEC, and 2.0 CEC relative to the clay capacity. Enlargement of clay interlayer spacing corresponded well with incorporation of organic amounts into the layered silicate clay. In Fig. 1a–e, the organoclays with different amounts of hydrophobic ODA-salt are shown with their dispersion abilities in water. Control of the pristine clay, Na+-MMT, was hydrophilic and well dispersed in water. With an increasing amounts of ODA additions from 1b–e, the organoclays become more hydrophobic and less dispersible in water; they tended to form aggregated lumps showing clear separation from the water phase and demonstrating a hydrophobic effect from the presence of ODA. However, due to its amphiphilic property, the organoclay could be homogeneously dispersed in water after agitation.
Table 1 X-ray analyses of Na+-MMT intercalated by the ODA-salts at different equivalent ratios
MMT/C18H37NH3+Cl− |
d spacing (Å) |
Equivalent ratio |
Weight ratioa (w/w) |
Determined by TGA. |
0 |
100/0 |
12 |
0.5 |
84/16 |
28 |
1.0 |
72/28 |
28 |
1.5 |
63/37 |
30 |
2.0 |
58/42 |
34 |
 |
| Fig. 1 Appearance of Na+-MMT clay intercalated with different equivalent ratios of ODA-salts at (a) 0 CEC or without ODA, (b) 0.5 CEC, (c) 1.0 CEC, (d) 1.5 CEC, and (e) 2.0 CEC. | |
Hydrocarbon absorbing properties of the organoclays
The ODA salts-modified organoclays were enabled to absorb hydrophobic organics such as hydrocarbons and wax, hence the practical uses for phase change materials (PCM) were explored. One of the paraffins, n-octadecane, was chosen to perform the hydrophobic absorption experiments with the organoclay. The phase change temperature of n-octadecane is between 25–29 °C which is suitable for practical uses around the ambient temperature such as the building materials.
With XRD analyses, the d spacing was measured before and after absorption of paraffin (Table 2). This process, consisting of two stages of intercalation,17 was reported previously for the hydrophobic effect in the second stage involving incorporating the hydrocarbon. The first intercalation involved the ionic exchange reaction with the ODA-salt through an exchange of the counter ions in Na+-MMT leading to a incorporated hydrophobic force that attracted the second stage of hydrocarbon accessibility to become PCM. Subsequently, with more paraffin absorption, basal spacing of the layered structure of clay was further expanded. The phenomenon of d spacing expansion for the two-stage intercalation was reported earlier.17,21 A conceptual illustration of paraffin incorporation in clay layered structure of ODA/MMT is shown in Scheme 1.
Table 2 Paraffin absorption efficacy and d spacing variation of organoclay with 1.0 CEC ODA
MMT/ODA/C18H38 (w/w/w)a(1.0 CEC) |
Efficiencyb (η) |
d spacing (XRD) (Å) |
Determined by TGA. Efficiency (η) was calculated according to eqn (1). |
71/29/0 |
Negligible |
28 |
61/24/15 |
0.20 |
29 |
51/21/28 |
0.40 |
30 |
43/17/40 |
0.70 |
30 |
21/8/71 |
2.5 |
30 |
13/5/82 |
4.5 |
30 |
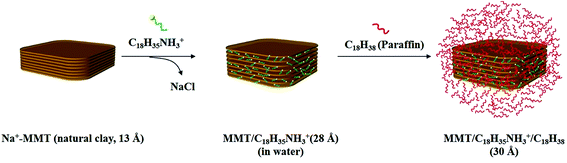 |
| Scheme 1 Conceptual illustration of an ODA salt-intercalated organoclay ready for further paraffin absorption. | |
As summarized in Table 2, the organoclay of ODA/MMT at 1.0 CEC had a 28% weight increase after absorbing paraffin, while the spacing slightly increased from 28 Å to 30 Å. With more paraffin addition and absorption, the clay spacings were maintained at 30 Å without further expansion, indicating the absorption is irrelevant to the interlayer of the clay structure and is only a peripheral phenomenon. After paraffin absorption, the resultant organoclays became less dispersible in water and more easily formed aggregates and precipitates from water. Apparently, the paraffin tended to be associated with the surface of the clay units and rendered the property to be amphiphilic or more hydrophobic. It was noticed that a further addition of more paraffin to the ODA/MMT, finally converted it into wax-like materials that floated on top of the water. Up to 4.5 fold of paraffin to organoclay by weight was determined to be the maximal absorption ability of ODA/MMT.
By comparison, if the paraffin was directly treated with Na+-MMT without the first stage of organic intercalation, then the layered structure of basal spacing was not expanded but showed only surface or peripheral absorption. Thus, organic modification of the clay through the ionic exchange reaction of Na+-MMT to expand the layered structure and incorporate some hydrophobic organics was necessary for effective second-stage paraffin absorption. The suitable PCM of clays possessed the fundamental units of layered structure from the two-stage intercalation via fatty-amine-salt ionic exchange reaction and hydrophobic paraffin incorporation.
The absorption efficiency of the second-stage incorporation of paraffin
The efficiency of paraffin absorption with the first-stage ODA-salt intercalation is demonstrated in Fig. 2. The efficiency (η) was estimated according to eqn (1), where mparaffin absorbed, moctadecylamine (mODA), and mMMT are the mass of absorbed paraffin, ODA, and MMT, respectively. |
η = (mparaffin absorbed)/(mODA) + (mMMT)
| (1) |
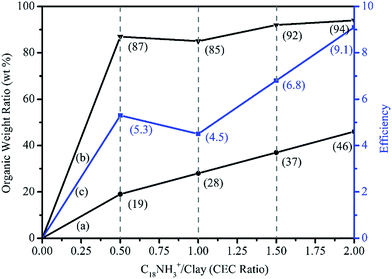 |
| Fig. 2 Organic fraction and paraffin absorption efficiency of ODA/MMT with different CEC intercalations (from 0.5 to 2.0); curve (a) organic fraction before paraffin absorption, curve (b) after paraffin absorption, and curve (c) the calculated paraffin-absorption efficiency corresponding with (b). | |
The absorption amount of paraffin increased depending on the amount of ODA intercalation in the first stage; then the incorporated hydrophobic force was attributed to the second stage absorption of paraffin. At 1.0 CEC intercalation of ODA salts, the paraffin absorption efficiency was 4.5 fold on the basis of the organoclay's weight, and increased to 6.8 and 9.1 fold in the cases of ODA intercalation increasing up to 1.5 CEC and 2.0 CEC (Fig. 2). However, the hydrocarbon absorption efficiency of the 0.5 CEC organoclay was 5.3 fold which is higher than 1.0 CEC organoclay (4.5 fold). But the 0.5 CEC organoclay would not form aggregated lumps in water when absorbing paraffin, implying a potential problem of purification in the preparation process. Without hydrophobic aggregation behavior, it seems that 0.5 CEC organoclay has a higher surface area for paraffin absorption efficiency than 1.0 CEC.
Thermal properties of paraffin in ODA/MMT
The paraffin, n-octadecane, is considered to be a phase change material with a suitable transition temperature and melting point at 28 °C. As shown in Fig. 3a, paraffin melts at 28 °C. When paraffin was absorbed in an organoclay with a clay layered structure, the powder form of the organoclays in aggregates remained a solid even when heated to 80 °C (Fig. 3b–e), which is quite different from pristine paraffin above 28 °C. As more paraffin was absorbed, the appearance of the ODA/MMT/paraffin complex changed from fine powders into large aggregated lumps. Their appearance showed that none of liquid leached out from the clay aggregates while heating up to 80 °C in an oven. The paraffin was stably incorporated in two fashions: incorporated in a layered structure and also as a peripheral unit surface in a tertiary phase aggregation. This stable confinement of paraffin in the clay aggregates is shown for suitable uses of PCM.
 |
| Fig. 3 Appearance of paraffin and ODA/MMT absorbed paraffin with different absorption efficiencies: (a) paraffin, the organoclays absorbed paraffin at (b) ηC18 = 0.26, (c) ηC18 = 1.2, (d) ηC18 = 2.5, and (e) ηC18 = 4.5. | |
Furthermore, the enthalpy of ODA/MMT/paraffin was measured by differential scanning calorimetry (DSC), as shown in Table 3 and Fig. 4. The measured enthalpy of n-octadecane was 243 J g−1, similar to its theoretical value, while the phase change temperature was between 25–29 °C. When the paraffin was absorbed by 1.0 CEC ODA/MMT, its enthalpy was measured to be 243 J g−1, and the thermal energy conversion efficiency reached 100%.
Table 3 Enthalpy of phase change materials
Entry |
Composition |
Weight ratioa (w/w/w) |
d spacing (Å) |
Efficiencyb (ηC18) |
ΔHfc (J g−1) |
ΔHcc (J g−1) |
MMT/ODA/C18H38 |
C18H38 |
MMT/ODA/C18H38 |
C18H38 |
Determined by TGA. Efficiency (η) was calculated by eqn (1). Measured by DSC. |
1 |
MMT/ODA/C18H38 |
13/5/82 |
30.4 |
4.5 |
199.7 |
243.4 |
199.2 |
242.9 |
2 |
(1.0 CEC) |
0/0/100 |
— |
— |
243.3 |
243.3 |
243.2 |
243.2 |
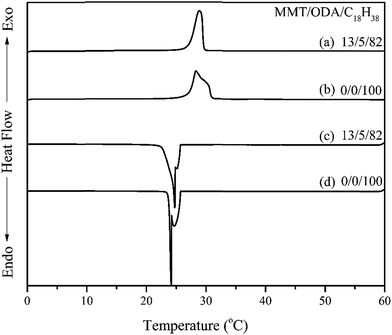 |
| Fig. 4 DSC curves of MMT/ODA/C18H38 at 13/5/82 weight ratio vs. C18H38 under temperature scanning between 0 °C and 60 °C. | |
Conclusion
The PCM of organoclays were prepared by a two-stage intercalation; the first was an ionic exchange intercalation of Na+-MMT layered silicate clays with n-octadecylamine (ODA) salts and then a secondary hydrophobic force attraction for paraffin occurred. The d spacing of the clay changed accordingly, expanding in organoclays after ODA intercalation and then further to a higher interlayer spacing after absorbing n-octadecane (paraffin) in the clay galleries. The absorption efficacy of paraffin was 4.5 times based on the 1.0 CEC intercalated ODA/MMT. The ODA/MMT/paraffin composition was suitable for a phase change material which remained in a solid state without any liquid leaking, even heating to a temperature above paraffin's melting point. The ODA/MMT/paraffin from two-step preparation is considered as a new energy storage material or PCM for absorbing and releasing heat according to the environmental temperature change.
References
- A. Kumar and S. K. Shukla, Energy Procedia, 2015, 74, 462–469 CrossRef.
- M. Akgün, O. Aydın and K. Kaygusuz, Appl. Therm. Eng., 2008, 28, 405–413 CrossRef.
- C. Alkan, Thermochim. Acta, 2006, 451, 126–130 CrossRef CAS.
- B. Zalba, J. M. Marín, L. F. Cabeza and H. Mehling, Appl. Therm. Eng., 2003, 23, 251–283 CrossRef CAS.
- Y. B. Cai, H. Z. Ke, J. Dong, Q. F. Wei, J. L. Lin, Y. Zhao, L. Song, Y. A. Hu, F. L. Huang, W. D. Gao and H. Fong, Appl. Energy, 2011, 88, 2106–2112 CrossRef CAS.
- A. A. Aydın and A. Aydın, Sol. Energy Mater. Sol. Cells, 2012, 96, 93–100 CrossRef.
- S. B. Şentürk, D. Kahraman, C. Alkan and İ. Gökçe, Carbohydr. Polym., 2011, 84, 141–144 CrossRef.
- D. Fernandes, F. Pitié, G. Cáceres and J. Baeyens, Energy, 2012, 39, 246–257 CrossRef CAS.
- B. Sandnes and J. Rekstad, Sol. Energy, 2006, 80, 616–625 CrossRef CAS.
- L. Wang and D. Meng, Appl. Energy, 2010, 87, 2660–2665 CrossRef CAS.
- K. Pielichowska, S. Głowinkowski, J. Lekki, D. Biniaś, K. Pielichowski and J. Jenczyk, Eur. Polym. J., 2008, 44, 3344–3360 CrossRef CAS.
- H. Inaba and P. Tu, Heat Mass Transfer, 1997, 32, 307–312 CrossRef CAS.
- C. H. Lee and H. K. Choi, Polym. Compos., 1998, 19, 704–708 CrossRef CAS.
- Y. Cai, H. Ke, J. Dong, Q. Wei, J. Lin, Y. Zhao, L. Song, Y. Hu, F. Huang, W. Gao and H. Fong, Appl. Energy, 2011, 88, 2106–2112 CrossRef CAS.
- J.-J. Lin, Y.-N. Chan and W.-H. Chang, in Advanced Nanomaterials, Wiley-VCH Verlag GmbH & Co. KGaA, 2010, pp. 459–480, DOI:10.1002/9783527628940.ch14.
- J.-J. Lin, Y.-N. Chan and Y.-F. Lan, Materials, 2010, 3, 2588–2605 CrossRef CAS.
- J.-Y. Chiou, R.-S. Hsu, C.-W. Chiu and J.-J. Lin, RSC Adv., 2013, 3, 12847–12854 RSC.
- C.-W. Chiu, T.-K. Huang, Y.-C. Wang, B. G. Alamani and J.-J. Lin, Prog. Polym. Sci., 2014, 39, 443–485 CrossRef CAS.
- C. Y. Liao, J. Y. Chiou and J. J. Lin, RSC Adv., 2015, 5, 100702–100708 RSC.
- R.-S. Hsu, W.-H. Chang and J.-J. Lin, ACS Appl. Mater. Interfaces, 2010, 2, 1349–1354 CAS.
- C.-C. Chou, F.-S. Shieu and J.-J. Lin, Macromolecules, 2003, 36, 2187–2189 CrossRef CAS.
|
This journal is © The Royal Society of Chemistry 2017 |