DOI:
10.1039/C7RA01197K
(Paper)
RSC Adv., 2017,
7, 21036-21044
Metformin as a versatile ligand for recyclable palladium-catalyzed cross-coupling reactions in neat water†
Received
26th January 2017
, Accepted 4th April 2017
First published on 12th April 2017
Abstract
We present the high catalytic activity of an in situ generated palladium(II)/metformin complex in neat water for the Suzuki–Miyaura and Sonogashira cross-coupling reactions. These reactions were performed in pure water without any co-solvent or mass transfer agent. The palladium(II)/metformin complex gave excellent to good yields for the Suzuki–Miyaura coupling reaction and catalyzed with a certain specificity to the aryl iodide coupling in the Sonogashira reaction. The methodology showcases a recyclability up to four catalytic runs for the Suzuki–Miyaura cross-coupling reaction, performed with only 0.5 mol% palladium loading.
Introduction
Nowadays, societies are paying more attention to the environmental impact of our life style. One of the main actual challenges in chemistry is to develop eco-friendly processes to lower their impact to the environment. In 1998, the 12 principles of Green Chemistry were identified to guide chemists through this modern vision of chemistry and they assert the main objectives of this new branch of chemistry.1 Many processes are considered green without applying all the 12 principles at the same time. One of these 12 principles of Green Chemistry encourages chemists to use and develop efficient and preferably recyclable catalytic systems, rather than stoichiometric reactions, in order to minimize the risks in terms of manipulation and toxicity. Metal-catalyzed cross-coupling reactions are a powerful tool for new bond formations (carbon–carbon, nitrogen–carbon, etc.) and are widely used in both academia2 and industry. Unfortunately they come with a heavy price in terms of safety and sustainability, as they are usually performed in toxic, carcinogenic and flammable organic solvents, generally required to solubilize the organic reactants and accelerate the reaction rates.3 To date, water remains the greenest known solvent because of its availability, stability, non-toxicity and non-flammability. Those properties make coupling reactions performed in water greener, because water is not considered as a waste. Therefore, ligands are required to perform metal-catalyzed cross-coupling reactions in neat water to protect the metal center from oxidation and deactivation. The Suzuki–Miyaura cross-coupling reaction has been reviewed many times in the past,4–11 but recently Ward et al.12 showed that the current challenges to be addressed for palladium-catalyzed coupling reactions performed in water are the reactivity of the aryl halide, the possibility to use low catalyst loadings, short reaction times and mild reaction conditions (low temperature).
Since recyclability is also a major concern in green chemistry, a water-soluble ligand may maintain the metal in the aqueous media and therefore may allow a simple recycling procedure, by extracting the organic product at the end of each catalytic cycle. Many water-soluble ligands have been explored to favour the cross-coupling reactions in aqueous media, such as phosphines. However, these ligands are toxic, moisture and/or air sensitive, and produce undesired side-products.13 N-Donor ligands are an interesting alternative for different reasons. First, numerous easy reactions lead to nitrogen-containing compounds with infinite possibilities of design, through the large choice of commercially available starting materials. Also, the nitrogen atom displays different hybridization states (sp, sp2, sp3) and anionic or neutral ligand can be envisaged for different types of interactions with a metal.
Biguanides (Fig. 1), water-soluble compounds, recently emerged as potential ligands in terms of Green Chemistry. They are known in medicine for their antihyperglycaemic,14 antimalarial15 and anticancer properties16 and have been found to form numerous complexes with bivalent transition metals.17 They have been used as polydentate ligands, well-known to stabilize metal nanoparticles and prevent their aggregation and/or deactivation.18,19 Veisi et al. studied the catalytic properties of different large and complex systems containing biguanides. They developed versatile and recyclable functionalized mesoporous silica molecular sieves SBA-15,20–22 biguanides-carbon nanotubes23–25 and biguanide–chitosan26 as catalyst for cross-coupling reactions. However, the cross-coupling reactions were performed in toxic DMF (the use of carbon nanotubes not respecting the Green Chemistry principles either) or a binary water
:
ethanol mixture.
 |
| Fig. 1 Structure of biguanides, metformin hydrochloride and its possible complexation to a transition metal [M]. | |
Green Chemistry is also about maximizing atom economy, i.e. using molecules containing fewer atoms possible. Commercial inexpensive metformin (Fig. 1), one of the smallest biguanides, is highly soluble in water and was proven to be non-toxic, since it was used as a treatment for type 2 diabetes by millions of people since 1957.27 It has previously been shown that metformin can complex palladium(II) and efficiently catalyze Suzuki–Miyaura reactions performed in a water
:
ethanol 1
:
1 mixture with 1 mol% catalyst loading.28 However this study does not cover the recyclability, nor the versatility aspects of the catalytic system and showed some limitations: the Pd loading has not been optimized, two equivalents of metformin were used and the reaction required the use of ethanol as co-solvent.
Herein, we report the catalytic efficiency and recyclability of a 1
:
1 metformin
:
Pd(OAc)2 complex in the Suzuki–Miyaura and the Sonogashira cross-coupling reactions in neat water at a very low catalyst loading. The catalytic species are formed in situ to avoid the money-, time- and energy-consuming synthesis or purification steps. This method is a green procedure, which simply consists in mixing the metal source(s) with the ligand in the presence of a base, for a short time prior to the addition of the substrates.29,30
Results and discussion
Optimization of the conditions for the Suzuki–Miyaura reaction
Our study began with the optimization of the reaction conditions. With a loading of 2 mol% of metformin
:
Pd (1
:
1) complex, complete conversion was obtained after 15 min at 100 °C (Table 1, entry 3). The time of formation of the catalytic species was then lowered to only 15 min at 100 °C (Table 1, entry 5).
Table 1 Formation of the catalytic species and reaction time optimization for the Suzuki–Miyaura cross-coupling reactiona

|
Entry |
Formation time of the catalytic species (min) |
Reaction time (min) |
Yieldb (%) |
Reaction conditions: 0.02 mmol Pd(OAc)2, 0.02 mmol metformin hydrochloride, 1 mmol 4′-bromoacetophenone, 1 mmol phenylboronic acid, 2 mmol K2CO3, 5 mL distilled water, 100 °C in air. Non-isolated yield determined by 1H NMR. |
1 |
120 |
120 |
100 |
2 |
120 |
30 |
94 |
3 |
120 |
15 |
93 |
4 |
120 |
10 |
61 |
5 |
15 |
15 |
99 |
The catalyst loading (Table 2) can be lowered to 0.0025 mol% without losing the efficiency of the system. Compared to recently reported catalytic systems for the Suzuki–Miyaura in neat water, this is a very low catalyst loading, the turnover number (TON) and turnover frequency (TOF) are excellent.30–33
Table 2 Optimization of the catalyst loading for the Suzuki–Miyaura cross-coupling reactiona

|
Entry |
Pd(OAc)2 (mol%) |
Metformin·HCl (mol%) |
Yieldb (%) |
TON |
TOF (s−1) |
Reaction conditions: Pd(OAc)2, metformin hydrochloride, 1 mmol 4′-bromoacetophenone, 1 mmol phenylboronic acid, 2 mmol K2CO3, 5 mL distilled water, 100 °C, 15 min, in air. Non-isolated yield determined by 1H NMR. |
1 |
2 |
2 |
99 |
50 |
0.06 |
2 |
0.0025 |
0.0025 |
95 |
38 000 |
41.8 |
3 |
0.001 |
0.001 |
Traces |
— |
— |
Only one equivalent of base (as K2CO3 is dibasic) was necessary to activate the palladium complex and the boronic acid (Table 3 and Fig. 2).34–36 A slight excess (1.1 eq.) was used to maintain the reaction media under basic conditions and the metformin ligand under its deprotonated form. The temperature needed to be kept at 100 °C to avoid a drastic decrease of the yield (Table 3, entry 5). These optimized conditions were tested under microwave irradiation, but only 79% yield was obtained after 15 min, compared to the 95% obtained with conventional heating. Suzuki–Miyaura couplings usually need higher temperatures under microwave conditions and the optimized conditions with conventional heating are therefore greener.37,38 Finally, the important role of the metformin as a ligand was highlighted through a control reaction. Indeed, at 0.0025 mol% palladium loading in the absence of metformin (Table 4, entry 1), only traces of the coupling product were detected by 1H NMR.37,39,40
Table 3 Optimization of the amount of base and temperature for the Suzuki–Miyaura cross-coupling reactiona

|
Entry |
Base amount (eq.) |
Temperature (°C) |
Yieldb (%) |
Reaction conditions: 0.000025 mmol Pd(OAc)2, 0.000025 mmol metformin hydrochloride, 1 mmol 4′-bromoacetophenone, 1 mmol phenylboronic acid, K2CO3, 5 mL distilled water, 15 min, in air. Non-isolated yield determined by 1H NMR. |
1 |
2 |
100 |
100 |
2 |
1.1 |
100 |
98 |
3 |
0 |
100 |
Traces |
4 |
0.5 |
100 |
60 |
5 |
1.1 |
90 |
14 |
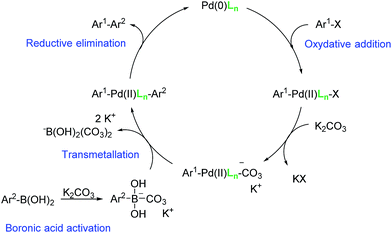 |
| Fig. 2 Catalytic cycle of the Suzuki–Miyaura cross-coupling reaction. | |
Table 4 Control reactions of the Suzuki–Miyaura cross-coupling reactiona

|
Entry |
Pd(OAc)2 (mol%) |
Metformin·HCl (mol%) |
Yieldb (%) |
Reaction conditions: 1 mmol 4′-bromoacetophenone, 1 mmol phenylboronic acid, 1.1 mmol K2CO3, 5 mL distilled water, 100 °C, 15 min, in air. Non-isolated yield determined by 1H NMR. |
1 |
0.0025 |
0 |
Traces |
2 |
0 |
0.0025 |
0 |
Nature of the active species of the Suzuki–Miyaura cross-coupling reaction: the catalyst poisoning test
The nature of the catalytic species was determined by the addition of mercury as the poisoning agent. In the case of a heterogeneous catalyst, the addition of mercury leads to its amalgamation on the surface of the active sites of the catalyst and totally inhibits its catalytic activity. If the catalyst is soluble in the reaction solvent, the addition of mercury has no effect on the catalyst's activity. The nature of our catalytic species was assessed by addition of 2 drops of mercury, prior to the addition of the substrates. The yield dramatically dropped to 3% which indicates that the catalytic species are not water-soluble.
Substrate scope for the Suzuki–Miyaura cross-coupling reaction
With the optimized reaction conditions in hand, the versatility of the catalytic system was studied through a substrate scope (Table 5). It is important to remind here that the optimized catalyst loading was determined for the reaction between 4′-bromoacetophenone and phenylboronic acid and may not be suitable for other substrates. The substrate scope was realized under the optimized reaction conditions for comparison. Aryl halides with electron-withdrawing groups and boronic acids with electron-donating groups, especially in ortho and para positions, are expected to favour the palladium insertion, and therefore increase the rate of the reaction.
Table 5 Substrates scope of aryl halides for the Suzuki–Miyaura cross-coupling reaction catalyzed by the Metformin/Pd complex in neat watera
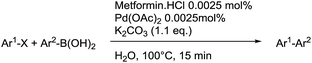
|
Entry |
Ar1-X |
Ar2-B(OH)2 |
Product |
Isolated yield (%) |
Reaction conditions: Pd(OAc)2, metformin hydrochloride, 1 mmol aryl halide, 1 mmol boronic acid, 1.1 mmol K2CO3, 5 mL distilled water, 100 °C, 15 min, in air. Yield after 15 min. Yield after 2 hours. Reaction performed with 0.5 mol% Pd/Met. |
1 |
 |
 |
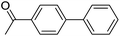 |
39b, 77c |
2 |
 |
 |
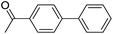 |
95 |
3 |
 |
 |
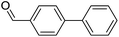 |
81 |
4 |
 |
 |
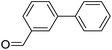 |
67 |
5 |
 |
 |
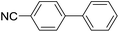 |
90b |
6 |
 |
 |
 |
46b |
7 |
 |
 |
 |
80b,c |
8 |
 |
 |
 |
97b,c |
9 |
 |
 |
 |
24b,c |
10 |
 |
 |
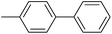 |
42b, 77c,d |
11 |
 |
 |
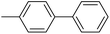 |
42b, 84c,d |
12 |
 |
 |
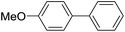 |
45b, 88c,d |
13 |
 |
 |
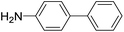 |
15b, 72c,d |
14 |
 |
 |
 |
61b,c |
15 |
 |
 |
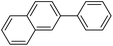 |
70c,d |
16 |
 |
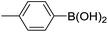 |
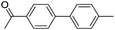 |
99b |
17 |
 |
 |
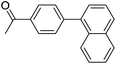 |
81b |
18 |
 |
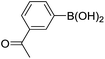 |
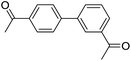 |
74b |
19 |
 |
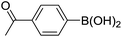 |
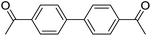 |
0b,c |
20 |
 |
 |
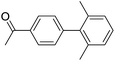 |
0b,c |
Different aryl halides with different functional groups were coupled to phenylboronic acid (Table 5) and a large range of yields were obtained. Electron-deficient aryl halides gave overall excellent yields at both studied catalyst loadings (Table 5, entries 1–6 and 16–18), while electron-rich aryl halides gave only low to moderate yields at the optimized 0.0025 mol% Pd loading after 2 hours (Table 5, entries 10–13). By increasing the catalyst loading and/or the reaction time, good to excellent yields were obtained, showing that less reactive substrates need a longer reaction time, such as the bulky naphtyl halides (Table 5, entries 14 and 15). In the Suzuki–Miyaura cross-coupling, aryl iodides are supposed to give higher yields than aryl the rate-limiting step is bromides.41 As it is not the case here, this result suggests that the oxidative addition in the catalytic cycle, as initially postulated by Suzuki and Miyaura.42 The water solubility of the substrates is also an important factor and aryl iodides are usually less soluble. Water solvation of the aryl halide may play a role on the oxidative addition step, making it faster in the case of aryl bromides. Moreover, at the end of the oxidative addition, KX (X = halide) is generally formed (Fig. 2). According to the HSAB theory,43 the formation of KBr is favoured compared to KI due to the nature of both the acid and the bases.
Different functionalized aryl chlorides (nitro, aldehyde, ketone, trifluoro) were used but no conversion was observed, even when the reaction was performed under nitrogen atmosphere.
When different arylboronic acids were coupled to 4′-bromoacetophenone excellent yields were obtained for those containing electron-rich substituents (Table 5, entries 15–17), as expected. 2,6-Dimethylphenylboronic and 4-acetylphenyl-boronic acid did not react at all even after 2 hours (Table 5, entries 19 and 20), probably due to the steric hindrance of the methyl groups and the presence of a para electron-withdrawing substituent on the respective boronic acids, which may reinforce the carbon–boron bond and disadvantage the transmetallation step.
Recyclability of the Suzuki–Miyaura cross-coupling reaction
The recyclability of the reaction was studied for different palladium loadings (Fig. 3). At 0.005 mol% Pd, we were able, to perform 2 catalytic runs without significant loss of activity, which is not surprising, the 0.0025 mol% being the optimized Pd loading. At 0.5 mol% of Pd, we were able to recycle up to 4 times with no significant loss of efficiency. These results are in accordance with previously reported complex biguanide-based catalytic systems.25,44 However, at higher Pd loadings, we were expecting more recycling cycles. This result suggests that the catalytic species gradually degraded, probably due to air and/or water exposure. This can be the result of a decomplexation of the metformin/Pd complex, which results into non-protected Pd species subject to oxidation in water.
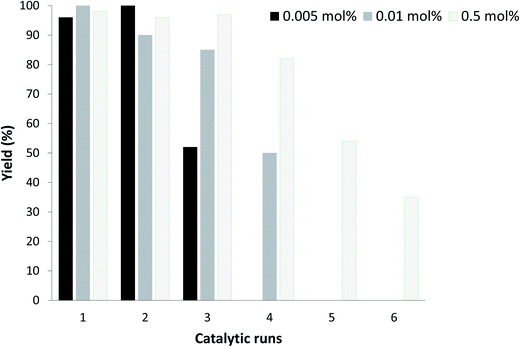 |
| Fig. 3 Recyclability of the Suzuki–Miyaura cross-coupling reaction at different Pd loadings. | |
Greenness of the process
Metrics have been created to measure the greenness of chemical processes. The more relevant here are: the E factor (eqn (1)), which focuses on organic waste only, and the Process Mass Intensity (PMI) (eqn (2)), preferred by the pharmaceutical industry because it includes the use of both organic solvents and water in the whole process (reaction and purification steps).45 |
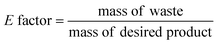 | (1) |
|
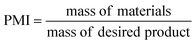 | (2) |
The values of E factor and PMI are sometimes misleading and difficult to compare because scientists do not always consider the purification steps' materials in their calculations. We decided here to consider them to have a better idea of the greenness of the process in its whole and to be able to compare it with others, keeping in mind that the purification steps usually produce the most waste.
Calculations of PMI and E factor have been done for our catalytic system to produce 1 mmol of 4′-phenylacetophenone and estimated for two recent catalytic Suzuki–Miyaura reactions performed in water (Table 6) for the same final product. Bhalla et al. have reported a very interesting photocatalytic system for the Suzuki–Miyaura coupling using a copper complex, but the work-up process involved a huge amount of dichloromethane. Therefore the PMI and E factor values are extremely high. Our catalytic system is very close to the one developed by Khan et al., where a pyridinium modified-β-cyclodextrin ligand was loaded with 3 mol% of Pd and used as catalyst for the same Suzuki–Miyaura coupling.
Table 6 PMI and E factor for recent catalytic systems for the Suzuki–Miyaura coupling in aqueous media
|
Bhalla et al.49 |
Khan et al.50 |
Our work |
PMI |
>2229.2 |
44.99 |
44.81 |
E factor |
>2232.6 |
32.95 |
30.35 |
Optimization of the Sonogashira cross-coupling reaction
To assess the versatility of metformin as a water-soluble ligand for cross-coupling reactions, we also investigated its potential as ligand in the Sonogashira cross-coupling in neat water (Table 7). The Sonogashira reaction was performed under nitrogen atmosphere with degassed water to avoid the undesired Glaser homocoupling product.46–48 Despite all our efforts, the homocoupling product was sometimes observed and therefore 1.5 equivalents of phenylacetylene were used to obtain complete conversion of the aryl halide.
Table 7 Optimization of the Sonogashira cross-coupling reactiona
The reaction time was optimized to 8 h at 100 °C while using a 5 mol% of palladium loading. The Pd loading cannot be lowered without a significant loss of efficiency of the reaction. The control reaction without metformin demonstrates that the ligand has a crucial role, as only 16% of the desired product were obtained. Metformin is therefore necessary to avoid the deactivation of the metals. We began the study with a Pd/Cu 1/2 ratio to overcome the potential oxidation of Cu(I) in water. When this ratio was reduced to 1/1, the resulting catalytic system was less efficient, even when 5 mol% of sodium formate was added as a reducing agent (Table 7, entry 5). As was the case for the Suzuki–Miyaura cross-coupling reaction, the temperature needed to be maintained at 100 °C, since the yield decreased to 66% when the reaction was performed at 90 °C.
Substrate scope for the Sonogashira cross-coupling reaction
With the optimized conditions in hand, the versatility of the catalytic system for the Sonogashira reaction was assessed through a substrate scope (Table 8). Different aryl iodides were coupled with phenylacetylene and again, the substrates containing electron-withdrawing groups gave excellent yields (from 70% to 97%) (Table 8, entries 1, 3 and 5), unlike substrates containing electron-donating groups which did not react (Table 8, entries 8 and 9). Moreover, this catalytic system seems to be specific to aryl iodides since aryl bromides were inactive, even when possessing electron-withdrawing groups (Table 8, entries 2, 4, 6 and 7). Potassium iodide (1 eq.) and iodine (0.2 eq.),51 were used as additives in order to replace the bromide and promote the insertion of Pd, but only traces of the desired product were detected by 1H NMR. In the Sonogashira reaction, the rate-limiting step is the transmetallation, when CuX is formed (Fig. 4). Indeed, during this step, both metals must have an electronic gain and yet, according to the HSAB theory, the formation of CuI is favoured, compared to CuBr.
Table 8 Substrates scope for the Sonogashira cross-coupling reaction catalyzed by the metformin–Pd/Cu in neat watera
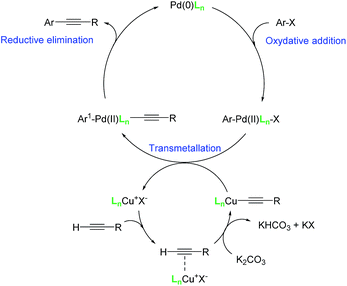 |
| Fig. 4 Catalytic cycle of the Sonogashira cross-coupling reaction. | |
Recyclability of the Sonogashira cross-coupling reaction
Recyclability of the Sonogashira cross-coupling was assessed at 5 and 10 mol% palladium loadings (Fig. 5). The increase of the Pd loading did not increase the number of possible catalytic runs demonstrating the degradation of the catalytic species.
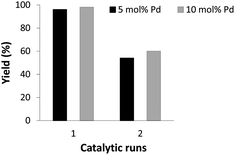 |
| Fig. 5 Recyclability study of the Sonogashira cross-coupling reaction at different Pd loading. | |
Experimental
All organic compounds were obtained commercially and used without further purification. 1H and 13C NMR spectra were recorded on a 400 MHz Bruker spectrometer. All Suzuki–Miyaura reactions were performed in vials in a Chemglass Pie-Block. All Sonogashira reactions were performed under nitrogen atmosphere using distilled water, previously degassed with nitrogen for 15 min.
General procedure for the Suzuki–Miyaura cross-coupling reaction
In a typical experiment, 25 μL of a solution of Pd(OAc)2 in methanol (1 mM), 25 μL of an aqueous solution of metformin hydrochloride (1 mM) and K2CO3 (1.1 eq.) were added to 2.5 mL of distilled water and stirred at 100 °C for 15 min. Arylboronic acid (1 mmol) and aryl halide (1 mmol) were added and the reaction mixture was stirred at 100 °C for 15 min. Afterwards, the reaction medium was cooled to room temperature and the aqueous phase was extracted with 3 × 2 mL of EtOAc. The organic phases were combined, dried over MgSO4, evaporated in vacuo and the obtained white powder was analyzed by 1H NMR to determine the yield. All products are known compounds and their 1H NMR data were in accordance with literature.
General procedure for the recycling process of the Suzuki–Miyaura cross-coupling reaction
After performing a typical Suzuki–Miyaura cross-coupling reaction as described above, the extractions were made in situ in the vial with a 1 mL syringe to collect the organic layers. K2CO3 (0.1382 g, 1 mmol), phenylboronic acid (0.1219 g, 1 mmol) and 4′-bromoacetophenone (0.199 g, 1 mmol) were added to the remaining aqueous phase and stirred at 100 °C for 15 min. The procedure described above was followed and the aqueous phase is once again available for subsequent recycling cycles.
Poisoning experiment of the Suzuki–Miyaura cross-coupling reaction
General procedure mentioned above was followed. Two drops of mercury were added after the formation of the catalyst before adding the substrates.
General procedure for the Sonogashira cross-coupling reaction
In a typical experiment, Pd(OAc)2 (11.22 mg, 0.05 mmol), CuI (19 mg, 0.10 mmol), metformin hydrochloride (24.84 mg, 0.15 mmol) and K2CO3 (0.2764 g, 2 mmol) were added to 5 mL of degassed distilled water and stirred at 100 °C for 30 min. Aryl acetylene (1.5 mmol) and aryl halide (1 mmol) were added and the reaction mixture was stirred at 100 °C for 8 h. Afterwards, the reaction medium was cooled to room temperature and the aqueous phase was extracted with 3 × 5 mL of EtOAc. The organic phases were combined, dried over MgSO4, evaporated in vacuo and the obtained product was analyzed by 1H NMR to determine the yield. All products are known compounds and their 1H NMR data were in accordance with literature.
General procedure for the recycling process of the Sonogashira cross-coupling reaction
After performing a typical Sonogashira cross-coupling reaction as described above, the extractions were made in situ in the flask with a 1 mL syringe to collect the organic layers. K2CO3 (0.2764 g, 2 mmol), phenylacetylene (165 μL, 1.5 mmol) and 4′-iodoacetophenone (0.199 g, 1 mmol) were added to the remaining aqueous phase and stirred at 100 °C for 8 h. The procedure described above was followed.
Conclusions
We have successfully demonstrated the use of metformin as a water-soluble ligand for the Suzuki–Miyaura and the Sonogashira cross-coupling reactions. For the Suzuki–Miyaura, metformin allowed the use of a very low 0.0025 mol% palladium loading. The substrate scope showed the great tolerance of the catalytic system to various functional groups. We were able to recycle up to four catalytic runs without significant loss of activity at only 0.5 mol% Pd. Unfortunately we observed that the catalytic species degraded in water even at high catalyst loadings. The versatility of the ligand was then confirmed by using metformin as ligand in the Sonogashira coupling reaction. The catalytic system was efficient over 8 hours and was specific to aryl iodides. However, no recycling can be performed. We are currently working on a new ligand which should offer a better protection of the metal center and therefore allow a better recycling of the catalytic species.
Acknowledgements
We gratefully acknowledge the Natural Sciences and Engineering Research Council of Canada (NSERC), the Fonds Quebecois de la Recherche sur la Nature et les Technologies (FRQ-NT), the Centre in Green Chemistry and Catalysis (CCVC) and the Université de Montréal for the financial support. We also thank Le Centre Régional de RMN and Le Centre Regional de Spectrométrie de masse of the Université de Montréal.
Notes and references
- P. T. Anastas and J. C. Warner, Green Chemistry: Theory and Practice, 1998 Search PubMed.
- C. C. Johansson Seechurn, M. O. Kitching, T. J. Colacot and V. Snieckus, Angew. Chem., 2012, 51, 5062–5085 CrossRef CAS PubMed.
- D. Prat, A. Wells, J. Hayler, H. Sneddon, C. R. McElroy, S. Abou-Shehada and P. J. Dunn, Green Chem., 2016, 18, 288–296 RSC.
- C. Najera, ChemCatChem, 2016, 8, 1865–1881 CrossRef CAS.
- D. Wang, C. Deraedt, J. Ruiz and D. Astruc, Acc. Chem. Res., 2015, 48, 1871–1880 CrossRef CAS PubMed.
- K. H. Shaughnessy, Molecules, 2015, 20, 9419–9454 CrossRef CAS PubMed.
- A. K. Rathi, M. B. Gawande, R. Zboril and R. S. Varma, Coord. Chem. Rev., 2015, 291, 68–94 CrossRef CAS.
- G. Herve and C. Len, Front. Chem., 2015, 3, 10 Search PubMed.
- C. Deraedt and D. Astruc, Acc. Chem. Res., 2014, 47, 494–503 CrossRef CAS PubMed.
- N. Liu, C. Liu and Z. Jin, Chin. J. Org. Chem., 2012, 32, 860 CrossRef CAS.
- C. A. Fleckenstein and H. Plenio, Chemistry, 2008, 14, 4267–4279 CrossRef CAS PubMed.
- A. Chatterjee and T. R. Ward, Catal. Lett., 2016, 146, 820–840 CrossRef CAS.
- S. Xu and Y. Tang, Lett. Org. Chem., 2014, 11, 524–533 CrossRef CAS.
- D. P. Thorne and T. D. Lockwood, Biochem. J., 1990, 266, 713–718 CrossRef CAS PubMed.
- N. A. Helsby, S. A. Ward, R. E. Howells and A. M. Breckenridge, Br. J. Clin. Pharmacol., 1990, 30, 287–291 CrossRef CAS PubMed.
- Y. Zhao, W. Wang, S. Guo, Y. Wang, L. Miao, Y. Xiong and L. Huang, Nat. Commun., 2016, 7, 11822–11830 CrossRef PubMed.
- P. Ray, Chem. Rev., 1961, 61, 313–359 CrossRef CAS.
- F. Chen, A. Li, G. Xu, Q. Li and Z. Lyu, J. Chin. Chem. Soc., 2016, 63, 770–775 CrossRef CAS.
- C. W. Lu, Y. Wang and Y. Chi, Chemistry, 2016 DOI:10.1002/chem.201601216.
- A. Alizadeh, M. M. Khodaei, D. Kordestania and M. Beygzadeh, J. Mol. Catal. A: Chem., 2013, 372, 167–174 CrossRef CAS.
- H. Veisi, D. Kordestani and A. R. Faraji, J. Porous Mater., 2013, 21, 141–148 CrossRef.
- H. Veisi, D. Kordestani, S. Hemmati, A. R. Faraji and H. Veisi, Tetrahedron Lett., 2014, 55, 5311–5314 CrossRef CAS.
- M. Navidi, N. Rezaei and B. Movassagh, J. Organomet. Chem., 2013, 743, 63–69 CrossRef CAS.
- H. Veisi, A. Khazaei, M. Safaei and D. Kordestani, J. Mol. Catal. A: Chem., 2014, 382, 106–113 CrossRef CAS.
- H. Veisi and N. Morakabati, New J. Chem., 2015, 39, 2901–2907 RSC.
- H. Veisi, M. Ghadermazi and A. Naderi, Appl. Organomet. Chem., 2016, 30, 341–345 CrossRef CAS.
- B. Viollet, B. Guigas, N. Sanz Garcia, J. Leclerc, M. Foretz and F. Andreelli, Clin. Sci., 2012, 122, 253–270 CrossRef CAS PubMed.
- A. Alizadeh, M. M. Khodaei, D. Kordestani and M. Beygzadeh, Tetrahedron Lett., 2013, 54, 291–294 CrossRef CAS.
- A. S. Kashin and V. P. Ananikov, J. Org. Chem., 2013, 78, 11117–11125 CrossRef CAS PubMed.
- V. Kairouz and A. R. Schmitzer, Green Chem., 2014, 16, 3117–3124 RSC.
- M. Charbonneau, G. Addoumieh, P. Oguadinma and A. R. Schmitzer, Organometallics, 2014, 33, 6544–6549 CrossRef CAS.
- Y.-s. Liu, N.-n. Gu, P. Liu, X.-w. Ma, Y. Liu, J.-w. Xie and B. Dai, Tetrahedron, 2015, 71, 7985–7989 CrossRef CAS.
- A. Fihri, D. Luart, C. Len, A. Solhy, C. Chevrin and V. Polshettiwar, Dalton Trans., 2011, 40, 3116–3121 RSC.
- N. Miyaura, K. Yamada and A. Suzuki, Tetrahedron Lett., 1979, 20, 3437–3440 CrossRef.
- L. Liu, S. Zhang, H. Chen, Y. Lv, J. Zhu and Y. Zhao, Chem.–Asian J., 2013, 8, 2592–2595 CrossRef CAS PubMed.
- N. Miyaura, K. Yamada, H. Suginome and A. Suzuki, J. Am. Chem. Soc., 1985, 107, 972–980 CrossRef CAS.
- N. E. Leadbeater and M. Marco, Org. Lett., 2002, 4, 2973–2976 CrossRef CAS PubMed.
- M. Massaro, S. Riela, G. Cavallaro, C. G. Colletti, S. Milioto, R. Noto, F. Parisi and G. Lazzara, J. Mol. Catal. A: Chem., 2015, 408, 12–19 CrossRef CAS.
- N. E. Leadbeater and M. Marco, J. Org. Chem., 2003, 68, 888–892 CrossRef CAS PubMed.
- M. Sarmah, A. Dewan, M. Mondal, A. J. Thakur and U. Bora, RSC Adv., 2016, 6, 28981–28985 RSC.
- T. D. Sheppard, Org. Biomol. Chem., 2009, 7, 1043–1052 CAS.
- N. Miyaura and A. Suzuki, J. Chem. Soc., Chem. Commun., 1979, 866–867 RSC.
- T.-L. Ho, Chem. Rev., 1975, 75, 1–20 CrossRef CAS.
- M. Beygzadeh, A. Alizadeh, M. M. Khodaei and D. Kordestani, Catal. Commun., 2013, 32, 86–91 CrossRef CAS.
- R. A. Sheldon, Green Chem., 2017, 19, 18–43 RSC.
- C. Glaser, Ber. Dtsch. Chem. Ges., 1869, 2, 422–424 CrossRef.
- A. S. Hay, J. Org. Chem., 1962, 27, 3320–3321 CrossRef CAS.
- P. Siemsen, R. C. Livingston and F. Diederich, Angew. Chem., Int. Ed., 2000, 39, 2632–2657 CrossRef CAS PubMed.
- G. Singh, M. Kumar, K. Sharma and V. Bhalla, Green Chem., 2016, 18, 3278–3285 RSC.
- R. I. Khan and K. Pitchumani, Green Chem., 2016, 18, 5518–5528 RSC.
- J. Mao, J. Guo, F. Fang and S.-J. Ji, Tetrahedron, 2008, 64, 3905–3911 CrossRef CAS.
Footnote |
† Electronic supplementary information (ESI) available: Suzuki–Miyaura and Sonogashira substrates scope references. See DOI: 10.1039/c7ra01197k |
|
This journal is © The Royal Society of Chemistry 2017 |
Click here to see how this site uses Cookies. View our privacy policy here.