DOI:
10.1039/C7RA01060E
(Paper)
RSC Adv., 2017,
7, 22706-22714
Damage of EPS and cell structures and improvement of high-solid anaerobic digestion of sewage sludge by combined (Ca(OH)2 + multiple-transducer ultrasonic) pretreatment
Received
24th January 2017
, Accepted 17th April 2017
First published on 26th April 2017
Abstract
The effects of individual and combined full-scale Ca(OH)2 and ultrasonic pretreatment on high-solid sludge (total solids content = 8%) were examined in terms of soluble organics increase, damage of extracellular polymeric substance (EPS) and cell structures, and subsequent anaerobic digestion. The maximum solubilization difference was obtained when combined pretreatment of 0.04 g g−1-TS lime and 225 kJ kg−1-TS ultrasound was used. The individual 0.04 g g−1 TS lime and low power ultrasonic pretreatment could only disintegrate the EPS partly, while the combined pretreatment damage not only EPS but also cells. Compared with the individual pretreatment, the combined pretreatment could result in a better performance of anaerobic digestion. When 0.04 g g−1-TS lime and 225 kJ kg−1-TS ultrasonic pretreatment was applied, the cumulative methane yield increased by 36.7% compared with that of individual lime pretreatment. These results proved that the multiple-transducer ultrasonic process could disintegrate high-solid sludge effectively when combined with Ca(OH)2, as well as enhance the methane yield.
1. Introduction
Anaerobic digestion is widely used for sewage sludge stabilization, resulting in sludge reduction and biogas production.1 Conventional anaerobic digestion (CAD) commonly treats sludge with a total solids (TS) content of 2 to 6%.2 Due to the high water content of sludge, traditional digesters commonly require a large volume, consequently, there are some shortcomings of CAD such as high heating cost, big floor space and complex operation.3 High-solid anaerobic digestion (HSAD) of sewage sludge may be a feasible alternative to CAD. The TS content in HSAD reactors is higher than 8%,2,4 and thus, HSAD requires smaller digesters and less energy for heating compared with CAD.5 However, inefficient hydrolysis is also the key shortcoming of HSAD, which was more deteriorated compared to CAD for its much poor substrates conversion efficiency under higher solids content.6
Sludge pretreatment has been studied to overcome this shortcoming in the last 30 years, such as mechanical,7 chemical8 and thermal6 methods. Sonication, a mechanical disintegration method for sludge, is an energy intensive process, which limits its full scale application.9 According to Asgharzadehahmadi et al.10 and Gogate et al.,11 the key to minimize the required ultrasonic energy consumption is to use a number of transducers in reactor with as large irradiation surface as possible, and using of multiple transducers is an alternative to single transducer with the same power. However, few literatures have reported full-scale application of multiple-transducer ultrasonic process.
Recently, ultrasonic pretreatment has often been combined with other disintegration methods such as alkaline,12 microwave13 and ozone14 pretreatments to achieve a higher disintegration degree. Especially, when ultrasonic pretreatment was combined with alkaline pretreatment, the disintegration efficiency was maximal, compared with that of thermo-acid, thermo-alkaline, and ultrasonic-acid combinations.15
The preferred alkali, in most cases, has been NaOH, which has higher solubilization efficiency than calcium hydroxide [Ca(OH)2].16 However, the sodium ions can reduce the soil permeability and increase the soil salinity and sodicity,17,18 and lead to soil deterioration. NaOH pretreatment limits long-term biosolids use in agriculture, and also involves higher costs. Ca(OH)2 relies on the same working principle but is 8–10 times cheaper than NaOH.19 Besides that, although it was confirmed that NaOH has higher solubilization efficiency than calcium hydroxide [Ca(OH)2],16,20 it lacked comparison study of anaerobic digestion performance of sludge after NaOH and Ca(OH)2 pretreatment in these literatures. According to Zhang and Jahng,21 there were inhibitory effects on sludge anaerobic digestion such as low methane yield and high level VFAs in the effluent when NaOH or KOH was used, and this inhibition was attributed to the cation toxicity of sodium and potassium ions, as proved by batch anaerobic assay in the presence of NaCl, KCl and CaCl2. When lime was used, it was found that methane yields (262.3 mL CH4 per g of CODadded) were significantly higher than that of NaOH (165.7 mL CH4 per g of CODadded) and KOH (155.3 mL CH4 per g of CODadded) under the same pH 9.5.22 Thus, Ca(OH)2 was used in this study.
Combined alkaline and ultrasonic pretreatment have been proved to enhance sludge digestion over either pretreatment alone.23 The high energy requirement in ultrasonic pretreatment process can be reduced by combining it with alkaline pretreatment.24 Moreover, combined alkaline and ultrasonic pretreatment has been proved to be an effective method by which to increase biogas production.25
So far, there has been a lack of study on the effect of combined full scale Ca(OH)2 and multiple-transducer ultrasonic pretreatment on high-solid sludge solubilization and subsequent HSAD. In current work, diluted dewatered sludge with TS 8% was subjected to the combined Ca(OH)2 and ultrasonic pretreatment. The objectives of this study were: (1) to investigate the effects of individual and combined Ca(OH)2 and ultrasonic pretreatment on high-solid sewage sludge (TS = 8%) solubilization; (2) to evaluate the damages of EPS and microbial cell structures of sludge before and after pretreatment; (3) to study the performance of HSAD before and after individual and combined pretreatment.
2. Materials and methods
2.1 Sludge samples and inoculums
Dewatered sludge was collected from a wastewater treatment plant with a capacity of 300
000 m3 day−1 in Tianjin, China; Anaerobic–Anoxic–Oxic (AAO) process was used in the plant to treat wastewater in which more than 70% was industrial wastewater. The discharged sludge, which was composed of 30% primary sludge and 70% waste activated sludge, was conditioned with polyacrylamide and dewatered to water content of 80% using a belt filter press. The pump was easy to be clogged when the TS content of sludge was more than 10% due to the high viscosity, and the dewatered sludge was diluted to TS 8% by tap water or Ca(OH)2 solution.
The inoculum used in this study was taken from a lab-scale anaerobic digester with a total volume of 5 L, and it had been digesting for 6 months at mesophilic temperature (37 ± 1 °C). Dewatered sludge, which was taken from the same wastewater treatment plant as that in this study, was diluted to TS 10% before feed into the lab-scale digester as substrate. The inoculum was incubated at 37 ± 1 °C for 2 weeks to ensure degradation of residual organic matter and to remove the dissolved methane before BMP-test.26
Characteristics of the diluted dewatered sludge (DDS) and inoculums were listed in Table 1.
Table 1 Characteristics of diluted dewatered sludge (DDS) and inoculums
Parameters |
DDS |
Inoculums |
pH |
6.9 |
7.3 |
Total solid (TS, g L−1) |
79.82 |
83.62 |
Volatile solid (VS, g L−1) |
47.61 |
34.48 |
Total chemical oxygen demand (TCOD, mg L−1) |
69 200 |
44 260 |
Soluble chemical oxygen demand (SCOD, mg L−1) |
462 |
1204 |
2.2 Full-scale ultrasonic reactor
As shown in Fig. 1, a double-layer ultrasonic reactor was developed with a working volume of 250 L. 128 piezoceramic flat transducers, were fixed evenly on both sidewalls with a total irradiating surface area of 2 m2. Ultrasonic frequency was fixed at 20 kHz, the power density can vary within the range of 0–0.04 kW L−1 by controlling the total power from 0–10 kW.
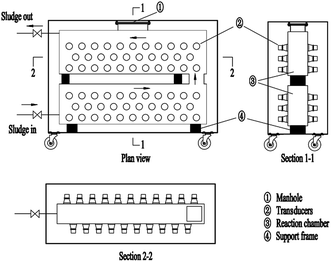 |
| Fig. 1 Diagram of full-scale ultrasonic bath reactor. | |
2.3 High-solid sewage sludge pretreatment
For individual Ca(OH)2 pretreatment, different amount of lime (CaO, Technical grade, Rual Co., Ltd, Tianjin, China) was first dissolved using tap water with doses of 0.02, 0.04, 0.06, 0.08, 0.1 g g−1 TS of sludge. Then the solution was used to dilute dewatered sludge to TS 8%. The alkaline pretreatment was implemented in a 1 m3 tank. The samples were stirred at 80 rpm with a paddle for 1 hour at ambient temperature.
Ultrasonic pretreatment was performed in the full-scale ultrasonic reactor. The input power was fixed at 5 kW. By controlling the sludge flow rate, the sludge was exposed to ultrasonic waves for 15, 30 and 45 minutes. The specific energy input can be calculated using eqn (1)
27 as follows:
|
 | (1) |
where
Espec is specific energy input in kW s kg
−1 of total solids (kJ kg
−1 of total solids),
P is ultrasonic power input (kW),
T is duration of exposure to ultrasonic waves (s),
V is volume of treated sludge (L), and TS is total solids concentration of the sludge (kg L
−1).
For combined pretreatment, 120 kg dewatered sludge were first diluted with 180 L Ca(OH)2 solution with different lime doses of 0.02, 0.04, 0.06, 0.08 and 0.1 g g−1 TS, and then the samples were mixed at 80 rpm with a paddle for 1 h at ambient temperature. After that, the sludge was pumped into the ultrasonic reactor, where it was disintegrated for 15, 30 and 45 minutes at 5 kW.
By diluting dewatered sludge with tap water, a control group was obtained.
2.4 Batch high-solid anaerobic digestion
After pretreatment, automatic methane potential test system II (AMPTSII, Bioprocess Control, Lund, Sweden) was used for batch anaerobic digestion experiments. 294 g inoculum and 106 g disintegrated sludge with a 2
:
1 VS ratio were added into bottles (650 mL), and the TS of the mixture was 8%. 294 g inoculum and 106 g deionized water were added to bottles and served as the blank. After sludge samples were added, each bottle was purged with nitrogen gas for 5 min to remove oxygen. Digestion temperature was set at 37.5 °C. CO2 was fixed in 100 mL bottles containing 80 mL of 3 M sodium hydroxide (NaOH) and thymolphthalein pH indicator solution. Biogas was real-time measured. The batch anaerobic digester ran for 30 days.
2.5 Extracellular polymeric substances (EPS) extraction
A heating extraction method was modified to extract the EPS from the sludge.28 10 mL of sludge samples were firstly centrifuged at 5000g for 10 min, the supernatants were collected as soluble EPS (S-EPS). The sludge sediments were re-suspended to their original volume using deionized water, then treated by ultrasound for 5 min (150 W, 20 kHz) and centrifuged at 5000g for 15 min, the supernatants were collected as loosely bound EPS (LB-EPS). The residuals left in the centrifuge tubes were re-suspended to their initial volume again with phosphate buffer (pH 7.5), sonicated for 5 min (150 W, 20 kHz), then heated at 60 °C for 30 min, finally centrifuged at 8000g for 15 min and the supernatants were tightly bound EPS (TB-EPS). In the present study, the sum of carbohydrate and protein represented the total EPS.29
2.6 Flow cytometry (FCM)
To study the damage to sludge microbial cell membranes during the disintegration process, sludge samples were fluorescently stained and measured by flow cytometry (FCM). Sludge samples were first diluted for 100 times with deionized water and then filtered through a 200-mesh sieve in order to avoid clogging of the cytometer nozzle. Ultrasonication (150 W, 3 min) was applied to disperse sludge flocs. After that, samples were harvested by centrifugation (5000g, 15 min). The sludge samples were stained by propidium iodide (PI) and FITC Annexin V (FITC Annexin V Apoptosis Detection Kit II, BD Pharmingen, Hei-delberg, Germany). FCM analysis was performed using a flow cytometer (FACSCalibur, BD, USA). The analysis were finished within 1 h after staining. Approximately 10
000 events were acquired for each sample in the flow cytometric measurement.
2.7 Protein and carbohydrate determination
The Lowry method was applied for protein determination.30 Bovine serum albumin (CAS no. 9048-46-8; Sigma) was used as standard. Reagents: Reagent 1: 2 percent Na2CO3 in 0.10 N NaOH. Reagent 2: 0.5% CuSO4·5H2O in 1% potassium sodium tartrate. Reagent 3: Alkaline copper solution. Mix 50 mL of Reagent A with 1 mL of Reagent B. Discard after 1 day. Reagent 4: titrate Folin–Ciocalteu phenol reagent (BR, Yuanye Co., Ltd, Shanghai, China) with NaOH to a phenolphthalein end-point. On the basis of this titration dilute the Folin reagent to make it 1 N in acid. Procedure: to 0.2 mL sample in a 5 mL test-tube, 1 mL of Reagent 3 is added. Mix well and allow to stand for 10 minutes or longer at room temperature. 0.10 mL of Reagent 4 is added very rapidly and mixed within a second. After 30 minutes at room temperature, the absorbance at 550 nm was read.
Anthrone method was used to determine carbohydrate with glucose (AR, Yuanli Co., Ltd, Tianjin, China) as standard.31 0.2 g anthrone (AR, Yuanye Co., Ltd, Shanghai, China) was dissolved in 100 mL 80% H2SO4. The reagent was allowed to stand for 30–40 min with occasional shaking until it was perfectly clear. The reagent was freshly prepared each day and used within 12 h. The anthrone reagent (5 mL) was pipetted into 10 mL tube and chilled in ice water. The sample (1 mL) was added, cooled for a further 5 min and then thoroughly mixed while still immersed in ice water. The tubes were loosely fitted with tube covers, heated as required in a vigorously boiling, constant level water bath and then cooled in ice water for 5 min. Absorbance was measured at 620 nm with a spectrophotometer.
2.8 Analytical methods
TS, VS, COD and total ammonia nitrogen (TAN) were analyzed according to the standard methods.32 The sludge sample was centrifuged for 10 min at 12
000g and immediately filtered through 0.45 μm membrane for analyzing the soluble COD (SCOD), protein, carbohydrate and TAN.
N-Acetylglucosamine content was determined by the Reissig colorimetric method with N-acetylglucosamine as a standard.33
Analysis were carried out in duplicate and average values were determined for each set.
2.9 Kinetic model
First-order kinetic model has been widely used to study the process of anaerobic digestion.34–37 Assuming first-order kinetic model for the hydrolysis of particulate organic matter, the cumulative methane production can be described by eqn (2). |
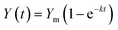 | (2) |
where Y(t) = the cumulative methane yield at a digestion time t days (mL g−1 VS); Ym = the maximum potential methane yield of substrate (mL g−1 VS); k = the methane production rate constant (first order disintegration rate constant) (1 per day); t = time (days); e is the exp (1) = 2.7183. A nonlinear curve fitting was performed by Origin 8.6 to determine Ym, k and the predicted methane yield.
2.10 Calculation
The COD solubilization permits to evaluate the maximum level of sludge solubilization. The COD solubilization was calculated using the eqn (3).25 |
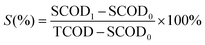 | (3) |
where S was COD solubilization (%), SCOD1 was SCOD concentration of the sludge after disintegration (mg L−1), SCOD0 was SCOD concentration of the sludge before disintegration (mg L−1), TCOD was TCOD concentration of the sludge before disintegration (mg L−1).
3. Results and discussion
3.1 Sludge solubilization after Ca(OH)2 and ultrasonic pretreatment
3.1.1 Individual Ca(OH)2 pretreatment. For individual Ca(OH)2 pretreatment, COD solubilization and concentrations of carbohydrate and protein in the supernatant were shown in Fig. 2. After 0.04 g g−1-TS lime pretreatment, COD solubilization increased to 2.4%. Higher lime dose was favourable to COD solubilization, the COD solubilization after 0.08 g g−1-TS pretreatment increased to 6.2%. The solubilization rate became slow when the lime dose exceeded 0.08 g g−1-TS.
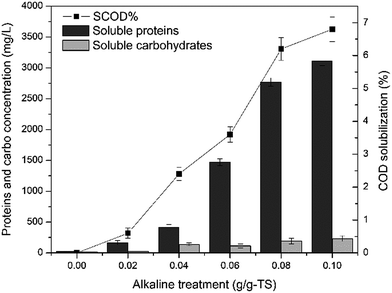 |
| Fig. 2 COD solubilization and concentrations of carbohydrate and protein in the supernatant after Ca(OH)2 pretreatment. | |
Compared with the 10–30% COD solubilization in previous Ca(OH)2 pretreatment studies,16,20,25 the solubilization results in this study were much lower. The reason may be due to that the technical grade lime with a lower purity was used in this study, and the sludge used in this study was high-solid dewatered sludge with more compact structure35 and higher alkaline buffer capacity.38
Higher lime dose was also favourable to the carbohydrate and protein solubilization, the protein was released much more than carbohydrate at the same lime dose. After 0.1 g g−1-TS lime pretreatment, the concentrations of carbohydrate and protein were the highest, which were 11.59 and 247.46 times higher than that of the raw sludge, respectively.
3.1.2 Individual ultrasonic pretreatment. Effect of ultrasonic pretreatment on COD solubilization was presented in Fig. 3. The COD solubilization was almost unchanged after ultrasonic pretreatment. The concentration of soluble carbohydrate and protein increased slightly after ultrasonic pretreatment. When the specific energy input was 675 kJ kg−1-TS, the concentration of soluble carbohydrate and protein were the highest, which were 1.87 and 20.05 times, higher than that of the raw sludge, respectively.
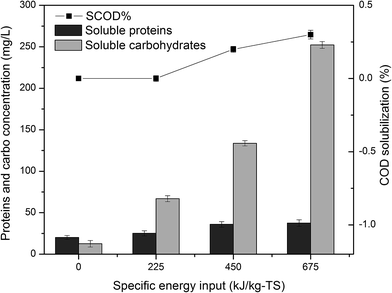 |
| Fig. 3 COD solubilization and concentrations of carbohydrate and protein in the supernatant after ultrasonic pretreatment. | |
There have been many studies reporting the solubilization of organic matters by ultrasonic pretreatment,39–41 and most of the studies showed higher solubilization rates than that in this study. The reason was that “weak” ultrasonic with low power density 0.02 W mL−1 was used in this study, and low energy input meant weak solubilization.
3.1.3 Combined alkaline and ultrasonic pretreatment. Table 2 revealed that after combined 0.04 g g−1-TS lime and 225 kJ kg−1-TS ultrasonic pretreatment, the SCOD increase was 2350 mg L−1 compared with the control, and the SCOD concentration increased with the increase of lime dose and ultrasonic energy input. D was defined as the SCOD increase of combined pretreated sludge compared with the raw sludge, and C was defined as the sum of SCOD increase after individual alkaline and ultrasonic pretreatment, E was the difference between D and C. Higher E-value meant stronger combined effect. As depicted in Table 2, the E-value were 630, 583 and 517 mg L−1 for 0.04 g g−1-TS lime, when combined with 225, 450 and 675 kJ kg−1-TS ultrasonic pretreatment, respectively. The E-value increased with the lime dose increased from 0.02 to 0.04 g g−1-TS. The highest E of 630 mg L−1 occurred when combined 0.04 g g−1-TS lime and 225 kJ kg−1-TS ultrasonic pretreatment was applied. The results demonstrated that there existed an optimal condition for combined lime and ultrasonic pretreatment, which agreed with the results of previous study by Kim et al.25 A possible reason of such combined disintegration was that lime made the sludge structure more susceptible to the hydromechanical shear forces caused by ultrasonic waves.42,43 However, the E-value was negative when the lime dose was 0.06 and 0.08 g g−1-TS, it was maybe because that the calcium ions could create a tighter bound network of biopolymers and flocs that was more resistant to shear.44 Consequently more lime used, the sludge was more resistant for ultrasonic disintegration.
Table 2 SCOD increase after combined alkaline and ultrasonic pretreatment
Pretreatment |
SCOD increase (mg L−1) |
Lime (g g−1 TS) |
Ultrasound (kJ kg−1 TS) |
Individual alkaline pretreatment (A) |
Individual ultrasonic pretreatment (B) |
Sum (C = A + B) |
Combined pretreatment (D) |
Difference (E = D − C) |
0.02 |
225 |
448 |
46 |
494 |
652 |
158 |
450 |
125 |
573 |
870 |
297 |
675 |
259 |
707 |
994 |
287 |
0.04 |
225 |
1674 |
46 |
1720 |
2350 |
630 |
450 |
125 |
1799 |
2382 |
583 |
675 |
259 |
1933 |
2450 |
517 |
0.06 |
225 |
2418 |
46 |
2464 |
2496 |
32 |
450 |
125 |
2543 |
2550 |
7 |
675 |
259 |
2677 |
2542 |
−135 |
0.08 |
225 |
4318 |
46 |
4364 |
4349 |
−15 |
450 |
125 |
4443 |
4340 |
−103 |
675 |
259 |
4577 |
4404 |
−173 |
3.2 Damages of EPS, cell walls and cell membranes
The sludge flocs are composed of EPS, microbial cells and some inorganic particles. EPS is found to have double layers including the loosely bound EPS (LB-EPS) which is diffused from the tightly bound EPS (TB-EPS) that surrounds the cells.28 The microbial cells are packed by cell walls and cell membranes.45 In order to gain an insight into the disintegration mechanism of combined lime and ultrasonic pretreatment, damages of EPS and cell structures were evaluated.
As demonstrated above, when the lime dose was 0.04 g g−1-TS, the highest E value was obtained. Therefore, combined pretreatment of 0.04 g g−1-TS lime and ultrasound was further investigated.
As depicted in Fig. 4(a), after individual ultrasonic pretreatment, the S-EPS concentrations increased little with slight decrease of LB-EPS, while TB-EPS was almost unchanged, suggesting negligible impacts of low power ultrasonic pretreatment on EPS, the result was consistent with the weak SCOD solubilization as mentioned above. The individual 0.04 g g−1-TS lime pretreatment caused an S-EPS increase (from 32 ± 3 to 865 ± 46 mg L−1), a TB-EPS decrease (from 2249 ± 47 to 1788 ± 43 mg L−1) and a slight LB-EPS increase. After combined 0.04 g g−1-TS lime and ultrasonic pretreatment with specific energy input of 225, 450 and 675 kJ kg−1-TS, the S-EPS concentration increased from 32 ± 3 to 1126 ± 34, 1288 ± 39 and 1355 ± 41 mg L−1, respectively, while the TB-EPS decreased from 2249 ± 47 to 1604 ± 48, 1529 ± 56 and 1308 ± 69 mg L−1, respectively. LB-EPS changed little. The results demonstrated that individual 0.04 g g−1-TS lime pretreatment and combined pretreatment had disintegrated the TB-EPS, the organic compounds in TB-EPS were released into LB-EPS and S-EPS.
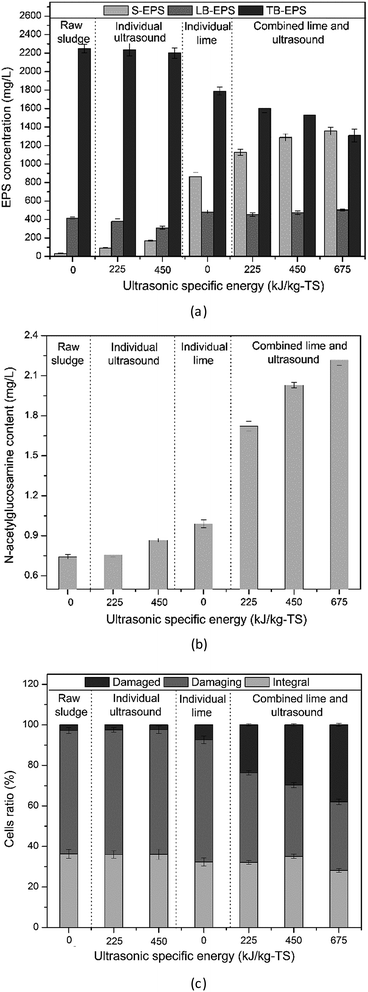 |
| Fig. 4 Damage to EPS (a), cell walls (b) and cell membranes (c) of combined 0.04 g g−1-TS lime with different ultrasound specific energy input. | |
When the microbial cell walls were damaged, the peptidoglycan, a polymer constituent of cell walls, was hydrolyzed and released N-acetylglucosamine.46 The content of soluble N-acetylglucosamine was measured to evaluate the damage of cell walls (Fig. 4(b)). The individual alkaline and ultrasonic pretreatment caused a small increase of the N-acetylglucosamine content, illustrating a slight increase of cell walls damaged ratio. After combined 0.04 g g−1-TS lime and 225, 450 and 675 kJ kg−1-TS ultrasonic pretreatment, the N-acetylglucosamine concentrations were 2.34, 2.74 and 2.97 times higher than that of raw sludge, respectively, which suggested an obvious damage to cell walls.
FCM assays were used to evaluate the damages of cell membrane of sewage sludge.47 Damaged cells were stained by PI, whereas cells at early period of apoptosis were stained by FITC Annexin V. The terms “integral”, “damaging” and “damaged” were used to refer to microbial cell with intact, partially damaged and completely damaged, respectively.48 As illustrated in Fig. 4(c), after individual ultrasonic pretreatment, the damaged and integral cell ratios were almost unchanged. The lime pretreatment with dose of 0.04 g g−1-TS showed an increase of the damaged cells ratio from 2.8% to 7.4% and slight decreases of damaging cells ratio from 60.9% to 60.3% and integral cells ratio from 36.3% to 32.3%. After combined 0.04 g g−1-TS lime and 225, 450 and 675 kJ kg−1-TS ultrasonic pretreatment, the ratio of damaged cells increased significantly from 2.8% to 23.7%, 29.7 and 38%, respectively, the damaging cells ratio decreased from 60.3% to 44.2%, 35.2% and 33.8%, respectively, while the ratio of integral cells was almost unchanged. It suggested that the partially damaged cells were disintegrated during the combined pretreatment, while the intact cells were tough enough to avoid the disintegration.
Individual ultrasound disintegrated LB-EPS slightly and hardly disintegrated cell walls and membranes, indicating that most ultrasonic energy was consumed to disintegrate the LB-EPS and no extra energy was used to disintegrate the cells. Hence, SCOD increase was small, and most of the organic matters were released from LB-EPS. This finding was consistent with the study of Bougrier et al.49 Individual 0.04 g g−1-TS lime pretreatment (pH ≈ 9) disintegrated cell structures slightly and damaged TB-EPS partly, illustrating that most organic matters were released from TB-EPS and few were from intracellular polymers. It was consistent with Xiao et al.,45 who concluded that cell walls and cell membranes began to be disintegrated when pH was 11.00 and under this pH, only EPS was partly damaged.
After combined 0.04 g g−1-TS lime and ultrasonic pretreatment, obvious cell wall and membrane damages were observed, which demonstrated that the solubilization of organic matters were not only from TB-EPS but also intracellular polymers. Based on different disintegration mechanisms of combined alkaline and ultrasonic pretreatment, the reason of the combined effect may be as following: (1) after lime pretreatment, the EPS in flocs was partly disintegrated, some cells lost the protection of EPS and thus exposed directly to the ultrasound, partially damaged cells was easier to be disintegrated by hydraulic shear force generated by ultrasound; the alkaline disintegration process was mainly happened in the first 30 min,50 the SCOD concentration was almost unchanged after 1 h for 0.04 g g−1-TS lime disintegration process in this study. When combining lime with ultrasonic pretreatment, the ultrasonic waves could weak the EPS structure, and then pH 9 in the ultrasonic reactor could induce further release of organic matters from EPS and cellular compounds of partially damaged cells.
3.3 Improvement of HSAD after pretreatment
3.3.1 Cumulative methane yield. The cumulative methane yield (CMY) of 30 days anaerobic digestion after individual and combined pretreatment was shown in Fig. 5. After Individual ultrasonic pretreatment, the CMY were almost unchanged after 30 day. When individual 0.04 g g−1-TS lime pretreatment was applied, the CMY increased from 93 ± 2 to 118 ± 4 mL CH4 per g VS. After the combined 0.04 g g−1-TS lime and 225, 450 and 675 kJ kg−1-TS ultrasonic pretreatment, the CMY increased from 93 ± 2 to 149 ± 9, 141 ± 8 and 161 ± 2 mL CH4 per g VS, respectively. The highest CMY of 161 ± 2 mL CH4 per g VS was found at 0.04 g g−1-TS lime and 675 kJ kg−1-TS ultrasonic pretreatment. Compared with the individual lime pretreatment, the combined 0.04 g g−1-TS lime and 675 kJ kg−1-TS ultrasonic pretreatment led to a highest CMY increase ratio of 36.7%.
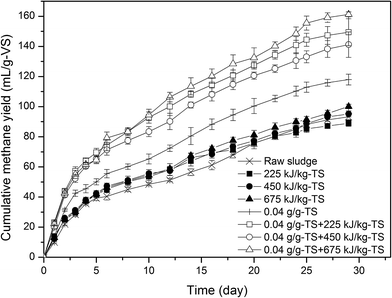 |
| Fig. 5 Cumulative methane yield during HSAD after individual and combined pretreatment. | |
When LB-EPS was slightly damaged after individual ultrasonic pretreatment, the CMY was almost unchanged. When TB-EPS was partly damaged and cells were slightly destroyed during individual 0.04 g g−1-TS lime pretreatment, the CMY increased by 26.6%, compared with the control group. When both TB-EPS and cell structures were disintegrated during combined pretreatment, a better performance of HSAD could be obtained, the CMY increased by 60.4%, 51.5% and 73.1% for combined 0.04 g g−1-TS lime and 225, 450 and 675 kJ kg−1-TS ultrasonic pretreatment, compared with the control group, respectively.
The organic matters in sludge were adsorbed or packed by EPS and cell structures.51 After combined pretreatment, TB-EPS, cell walls and cell membranes were damaged as mentioned above. The protection to these organic matters of the EPS, cell walls and cell membranes were weakened or damaged. Thus, the organic matters in EPS and intracellular polymers were easier to be utilized by the fermentation bacteria and methanogens during the anaerobic digestion process, which contributed to the CMY increase.
The dewatered sludge used in this study was from the same waste water treatment plant as the previous study of Zhang et al.,35 who observed that the CMY values of high-solid dewatered sludge (22% TS) increased from 74.3 mL CH4 per g VS to 100.3 and 127.03 mL CH4 per g VS after 20 mg NaOH and 120 °C pretreatment, respectively. Higher CMY values were obtained in this study, which demonstrated that high-solid anaerobic digestion results in a decrease of methane yield per g volatile solid (VS).
3.3.2 Organic components degradation. As summarized in Table 3, compared with the control group, the consumption rates of VS, total COD, total protein, and total carbohydrate were higher after anaerobic digestion for individual lime and combined pretreatment, while those were almost unchanged for individual ultrasonic pretreatment. The results suggested that more organic matters in sludge were degraded after individual lime and combined pretreatment than that in control group during HSAD. These results were consistent with the methane yield results as demonstrated above, both soluble and insoluble organic matters in the disintegrated sludge were easier to be transformed into methane.
Table 3 Organic components degradation during HSAD after individual and combined pretreatment
parameters |
Raw |
Individual ultrasound (kJ kg−1-TS) |
Individual lime (0.04 g g−1-TS) |
Combined lime (0.04 g g−1-TS) + ultrasound (kJ kg−1-TS) |
225 |
450 |
675 |
225 |
450 |
675 |
VS consumed (%) |
27.83 |
28.12 |
27.45 |
29.6 |
38.67 |
41.61 |
40.97 |
41.68 |
TCOD consumed (%) |
26.27 |
25.41 |
26.28 |
27.13 |
35.82 |
38.24 |
38.11 |
39.44 |
T-protein consumed (%) |
31.78 |
33.71 |
32.52 |
34.12 |
46.44 |
49.31 |
49.62 |
48.18 |
T-carbohydrate consumed (%) |
21.22 |
23.33 |
23.66 |
24.71 |
27.22 |
29.31 |
29.55 |
28.54 |
TANBA (mg L−1) |
266 |
268 |
266 |
269 |
292 |
320 |
314 |
336 |
TANAA (mg L−1) |
1362 |
1378 |
1372 |
1388 |
1499 |
1606 |
1574 |
1660 |
The TAN concentrations in the disintegrated sludge groups were higher than that of the control, and the TAN concentrations were safe for HSAD.
3.4 Kinetic model study
Table 4 summarized the study results of the first-order kinetic model. The difference between the predicted and measured methane yields was in the range of 4.1–7.4%, and the correlation coefficients R2 were higher than 0.95, which suggested that this first-order kinetic model was well fit to the methane production curve in the HSAD system. When the individual ultrasonic pretreatment was applied, Ym didn't increase compared with the control, which was consistent with the weak solubilization as mentioned above. The Ym increased from 100.55 to 121.75 when individual lime pretreatment was used. After combined 0.04 g g−1-TS lime and 225, 450 and 675 kJ kg−1-TS ultrasonic pretreatment, Ym increased from 100.55 to 146.42, 137.72, 162.99 mL CH4 per g VS, respectively. Ym of combined 0.04 g g−1-TS lime and 675 kJ kg−1-TS ultrasonic pretreatment group was the highest, which was due to the highest damage ratio of TB-EPS and cells.
Table 4 Kinetics result of the HSAD after individual and combined pretreatment from first-order kinetic model
Ultrasonic (kJ kg−1-TS) |
Lime (g g−1-TS) |
Ym |
K (1 per day) |
R2 |
Predicted methane yield (mL CH4 per g-VS) |
Measured methane yield (mL CH4 per g-VS) |
Difference (%) |
0 |
0 |
100.55 |
0.0733 |
0.9545 |
88.57 |
93.2 |
4.90 |
225 |
0 |
87.65 |
0.1106 |
0.9723 |
84.10 |
89 |
5.50 |
450 |
0 |
92.91 |
0.1025 |
0.9633 |
88.16 |
95.2 |
7.40 |
675 |
0 |
100.56 |
0.0902 |
0.9707 |
93.21 |
100.1 |
6.89 |
0 |
0.04 |
121.75 |
0.0913 |
0.9677 |
113.12 |
118 |
4.10 |
225 |
0.04 |
146.42 |
0.1149 |
0.9664 |
141.19 |
149.5 |
5.50 |
450 |
0.04 |
137.72 |
0.1121 |
0.9648 |
132.39 |
141.2 |
6.20 |
675 |
0.04 |
162.99 |
0.0969 |
0.974 |
153.18 |
161.3 |
5.00 |
The methane potential of the sludge (Ym value) was lower in this study compared with that in some previous studies,36,37,52 the reason might be attributed to that high-solid sludge was used in this study. The main disadvantages of HSAD were that the low mixing efficiency due to high viscosity of the solids, and restricted digestion due to blocked mass and heat transfer, which resulted in the decrease of methane potential.6,35
4. Conclusions
(1) The combined pretreatment suggested a combined effect on solubilization, and the maximum difference of SCOD increase was 630 mg L−1, and it occurred when 0.04 g g−1-TS lime and 225 kJ kg−1-TS ultrasonic pretreatment was applied.
(2) The individual 0.04 g g−1 TS lime and low power ultrasonic pretreatment could only disintegrate the TB-EPS, while the combined pretreatment damage not only TB-EPS but also cells.
(3) After combined pretreatment, both TB-EPS and cell structures were disintegrated, the CMY increased by 36.7%, compared with that after individual lime pretreatment.
Acknowledgements
This research was funded by the Special Fund for Agro-scientific Research in the Public Interest (201303101).
Notes and references
- V. N. Gunaseelan, Biomass Bioenergy, 1997, 13, 83–114 CrossRef CAS.
- X. Liao and H. Li, Appl. Energy, 2015, 148, 252–259 CrossRef CAS.
- N. Duan, B. Dong, B. Wu and X. Dai, Bioresour. Technol., 2012, 104, 150–156 CrossRef CAS PubMed.
- C. Liu, H. Li, Y. Zhang, D. Si and Q. Chen, Bioresour. Technol., 2016, 216, 87–94 CrossRef CAS PubMed.
- J. Guendouz, P. Buffière, J. Cacho, M. Carrère and J. P. Delgenes, Water Sci. Technol., 2008, 58, 1757 CrossRef CAS PubMed.
- X. Liao, H. Li, Y. Zhang, C. Liu and Q. Chen, Int. Biodeterior. Biodegrad., 2016, 106, 141–149 CrossRef CAS.
- P. Kampas, S. A. Parsons, P. Pearce, S. Ledoux, P. Vale, J. Churchley and E. Cartmell, Water Res., 2007, 41, 1734–1742 CrossRef CAS PubMed.
- S. Khatri, S. Wu, S. Kizito, W. Zhang, J. Li and R. Dong, Appl. Energy, 2015, 158, 55–64 CrossRef CAS.
- S. Kavitha, J. Rajesh Banu, C. D. IvinShaju, S. Kaliappan and I. T. Yeom, Bioresource Technol, 2016, 221, 1–8 CrossRef CAS PubMed.
- S. Asgharzadehahmadi, A. A. Abdul Raman, R. Parthasarathy and B. Sajjadi, Renewable Sustainable Energy Rev., 2016, 63, 302–314 CrossRef CAS.
- P. R. Gogate, V. S. Sutkar and A. B. Pandit, Chem. Eng. J., 2011, 166, 1066–1082 CrossRef CAS.
- Y. Yan, L. Feng, C. Zhang, C. Wisniewski and Q. Zhou, Water Res., 2010, 44, 3329–3336 CrossRef CAS PubMed.
- A. M. Yeneneh, A. Kayaalp, T. K. Sen and H. M. Ang, J. Environ. Chem. Eng., 2015, 3, 2514–2521 CrossRef CAS.
- S. Yang, W. Guo, G. Cao, H. Zheng and N. Ren, Bioresour. Technol., 2012, 124, 347–354 CrossRef CAS PubMed.
- X. Liu, H. Liu, J. Chen, G. Du and J. Chen, Waste Manage., 2008, 28, 2614–2622 CrossRef CAS PubMed.
- M. López Torres and M. D. C. Espinosa Lloréns, Waste Manage., 2008, 28, 2229–2234 CrossRef PubMed.
- T. A. Gemtos, N. Chouliaras and S. Marakis, J. Agric. Eng. Res., 1999, 3, 283–296 CrossRef.
- P. Rengasamy and K. A. Olsson, Soil Res., 1991, 6, 935–952 CrossRef.
- W. C. Khor, K. Rabaey and H. Vervaeren, Bioresour. Technol., 2015, 176, 181–188 CrossRef CAS PubMed.
- J. Kim, C. Park, T. H. Kim, M. Lee and S. Kim, J. Biosci. Bioeng., 2003, 95, 217–275 Search PubMed.
- L. Zhang and D. Jahng, J. Hazard. Mater., 2010, 182, 536–543 CrossRef CAS PubMed.
- L. Zhang and D. Jahng, J. Hazard. Mater., 2010, 182, 536–543 CrossRef CAS PubMed.
- N. D. Park, S. S. Helle and R. W. Thring, Biomass Bioenergy, 2012, 46, 750–756 CrossRef CAS.
- I. Zawieja, L. Wolny and P. Wolski, Desalination, 2008, 222, 374–381 CrossRef CAS.
- D. Kim, E. Jeong, S. Oh and H. Shin, Water Res., 2010, 44, 3093–3100 CrossRef CAS PubMed.
- R. Chandra, H. Takeuchi, T. Hasegawa and R. Kumar, Energy, 2012, 43, 273–282 CrossRef CAS.
- X. Feng, H. Lei, J. Deng, Q. Yu and H. Li, Chemical Engineering and Processing: Process Intensification, 2009, 48, 187–194 CrossRef CAS.
- Z. Chen, W. Zhang, D. Wang, T. Ma, R. Bai and D. Yu, Water Res., 2016, 103, 170–181 CrossRef CAS PubMed.
- H. Liu and H. H. P. Fang, J. Biotechnol., 2002, 95, 249–256 CrossRef CAS PubMed.
- O. H. Lowery, N. J. Rosebrough, A. L. Farr and R. J. Randall, J. Biol. Chem., 1951, 193, 265–275 Search PubMed.
- A. F. Gaudy, Ind. Water Wastes, 1962, 1, 17–27 Search PubMed.
- S. APHA, Methods for the Examination of Water and Wastewater, American Public Health Association, Washington DC, USA, 21st edn, 2005 Search PubMed.
- J. L. Reissig, J. L. Strominger and L. F. Leloir, J. Biol. Chem., 1955, 2, 959–966 Search PubMed.
- G. K. Kafle, S. H. Kim and K. I. Sung, Bioresour. Technol., 2013, 127, 326–336 CrossRef CAS PubMed.
- S. Zhang, H. Guo, L. Du, J. Liang, X. Lu, N. Li and K. Zhang, Bioresour. Technol., 2015, 185, 171–177 CrossRef CAS PubMed.
- W. Zhang, Q. Lang, S. Wu, W. Li, H. Bah and R. Dong, Bioresour. Technol., 2014, 156, 63–69 CrossRef CAS PubMed.
- G. K. Kafle and S. H. Kim, Appl. Energy, 2013, 103, 61–72 CrossRef CAS.
- W. Fang, P. Zhang, G. Zhang, S. Jin, D. Li, M. Zhang and X. Xu, Bioresour. Technol., 2014, 168, 167–172 CrossRef CAS PubMed.
- S. Pilli, S. Yan, R. D. Tyagi and R. Y. Surampalli, J. Environ. Manage., 2016, 166, 374–386 CrossRef CAS PubMed.
- N. T. Le, C. Julcour-Lebigue and H. Delmas, Ultrason. Sonochem., 2013, 20, 1203–1210 CrossRef CAS PubMed.
- V. K. Tyagi, S. Lo, L. Appels and R. Dewil, Crit. Rev. Environ. Sci. Technol., 2014, 44, 1220–1288 CrossRef.
- I. Doğan and F. D. Sanin, Water Res., 2009, 43, 2139–2148 CrossRef PubMed.
- S. Şahinkaya and M. F. Sevimli, J. Ind. Eng. Chem., 2013, 19, 197–206 CrossRef.
- E. Neyens, J. Baeyens and C. Creemers, J. Hazard. Mater., 2003, 97, 295–314 CrossRef CAS PubMed.
- B. Xiao, C. Liu, J. Liu and X. Guo, Bioresour. Technol., 2015, 196, 109–115 CrossRef CAS PubMed.
- X. Guo, J. Liu and B. Xiao, J. Biotechnol., 2014, 188, 130–135 CrossRef CAS PubMed.
- X. Han, Z. Wang, X. Wang, X. Zheng, J. Ma and Z. Wu, Water Res., 2016, 88, 293–300 CrossRef CAS PubMed.
- T. Abzazou, H. Salvadó, C. Bruguera-Casamada, P. Simón, C. Lardín and R. M. Araujo, Environ. Sci. Pollut. Res., 2015, 22, 11446–11455 CrossRef CAS PubMed.
- C. Bougrier, H. Carrère and J. P. Delgenès, Chem. Eng. J., 2005, 106, 163–169 CrossRef CAS.
- H. Li, C. Li, W. Liu and S. Zou, Bioresour. Technol., 2012, 123, 189–194 CrossRef CAS PubMed.
- W. Zhang, B. Cao, D. Wang, T. Ma, H. Xia and D. Yu, Water Res., 2016, 88, 728–739 CrossRef CAS PubMed.
- S. P. S. M. S. Rao, Bioresour. Technol., 2004, 95, 173–185 CrossRef PubMed.
|
This journal is © The Royal Society of Chemistry 2017 |