DOI:
10.1039/C6RA25383K
(Paper)
RSC Adv., 2017,
7, 30262-30273
Upconversion fluorescence imaging of HeLa cells using ROS generating SiO2-coated lanthanide-doped NaYF4 nanoconstructs
Received
17th October 2016
, Accepted 19th May 2017
First published on 12th June 2017
Abstract
Inorganic nanomaterials able to generate reactive oxygen species (ROS) are promising components for modern medical applications. Activated by near-infrared light, up-converting β-NaYF4 doped with Er3+–Yb3+ and Tm3+–Yb3+ pair ions nanoparticles (UCNPs), have a wide range of applications in biological imaging as compared to traditional reagents excited by ultra-violet or visible light. We analysed the green-red and the blue-red luminescence to explain the mechanism of the upconversion depended on the surface condition. The influence of SiO2 coating on the cytotoxicity of the as-produced UCNPs towards HeLa cancer cells was reported. We demonstrated a possibility of a direct UCNPs application to photodynamic therapy, without need to attach additional molecules to their surface. The presence of Tm3+–Yb3+ pair ions, thus ROS generation capability, renders the SiO2 shell coated nanoparticles to become potentially useful theranostic agent.
1 Introduction
Presently, cancer is one of the most prevalent diseases. In Europe, this pathology is responsible for 20% of deaths, with more than 3 million new cases and 1.7 million deaths every year. Actually, after cardiovascular diseases, cancer is the most important cause of deaths and morbidity in Europe (WHO). Fluorescent techniques are known as ultra-sensitive diagnostic tools to identify some pathogenic cells at the molecular level. Optical imaging is extremely useful in biomedical research for early detection of pathogenic cells as well as for therapy. Many types of luminophores are currently applied in bioimaging (e.g. quantum dots, fluorescent proteins, organic dyes, dye doped silica nanoparticles, metallic nanoparticles). However, application of organic probes for luminescence imaging of living organism has some limitations. For instance, the excitation of the traditional bio-labels usually requires UV or VIS light, leading to: (I) low signal-to-noise ratio due to high biological autofluorescence, (II) small light penetration depth, and (III) possible cell photodamages.1,2
Therefore, it is desirable to use fluorescent biolabels that can be excited by near infrared (NIR) light. In particular, NIR light is safe to the human body and can penetrate into tissues up to several centimetres.3,4 Upconversion nanoparticles (UCNPs), which can convert photons of lower energy (e.g. NIR light) to higher energy (e.g. visible light) via a two-photon or multiphoton upconverssion mechanisms,5 represent a new class of fluorescent biolabels. UCNPs have a relatively high quantum yield, as compared to a two-photon excitation of organic dyes, or quantum dots used in two-photon microscopy, narrow emission peak, large anti-Stokes shift, good chemical stability, and relatively low toxicity.6,7 Since UCNPs are NIR light active, the signal-to-noise ratio and sensitivity of the optical detection can be improved due to the absence of autofluorescence.8–10 Therefore, UCNPs are promising alternatives to traditional fluorescent biolabels for cell imaging and possess prominent potential in biological and clinical applications such as photodynamic therapy (PDT). In this therapy as a lethal factor are using reactive oxygen species (ROS).11 In traditional case of PDT, photosensitizer is activate by visible light to generate ROS, as described above this kind of light has limitation at tissues penetration. Materials with upconverting properties could be used to activation of photosensitizer directly in the environment of tumor. UCNPs excited by NIR light transfer absorbed energy to photosensitizer molecules and cause generation of ROS.12,13
The most efficient host material for the 980 nm upconversion is the hexagonal-phase (β-phase) NaYF4. The β-NaYF4 host is frequently doped with rare-earth (RE) ions, as for instance Yb3+–Er3+ or Yb3+–Tm3+ ion pair.14–17 Monodisperse UCNPs are usually synthesized in organic solvents at high-temperatures. However, the most commonly used hydrophobic capping agent, namely oleic acid, limits their biological applications.17–22
The coating of the nanoparticles (NPs) by thin silica (SiO2) layer or metal oxides makes them dispersible in water. The use of SiO2 for external coating of lanthanide-doped UCNPs is an attractive alternative because the surface chemistry of silica spheres is well documented and silica is known to be relatively harmless while using in biological systems.23–25 Moreover, the thickness and the surface properties of SiO2 shell can be easily adjusted to favour secretion of SiO2-coated UCNPs through the kidneys.26
Both types of materials, surface unmodified UCNPs and core–shell with solid oxide, have a potential to attach some molecules.27 This fact enhances their application in selected cancer therapy called photodynamic therapy. In this case UCNPs must be connect with photosensitizer molecules.28,29 Presented nanoparticles, doped with thulium ions, are an alternative system, avoiding unstable (photobleaching) molecules of photosensitizers.30 Wide emission spectrum, dependent on the ions pair (Yb3+–Tm3+), allows to apply the upconverting system for generation of reactive oxygen species without any additional reagents like photosensitizer molecules present in the traditional photodynamic therapy.31 High energy emitted UV light is capable to decompose water molecules to toxic radicals.
Herein, we report on a general method of silica-coating β-NaYF4:20%Yb3+,2%Er3+ and β-NaYF4:20%Yb3+,0.2%Tm3+ NPs. The NaYF4 host matrix has a very low phonon energy and therefore minimizes the quenching of the lanthanide ions excited-state, which results in a high quantum yield for upconversion luminescence (UCL).32–39 Depending on the RE doping, under NIR excitation UCL emission of different colours is obtained, thus making the obtained UCNPs interesting for multicolour bioimaging, basic material in modern photodynamic therapy.
In brief, we report on the synthesis of functionalized SiO2-coated UCNPs with sizes smaller than 20 nm, which form stable aqueous suspensions and therefore can be applied for biological optical imaging. The SiO2-coating improves the overall biocompatibility of the UCNPs and makes them suitable for functionalization with biologically-active molecules.
2 Methods
2.1. Materials
Trifluoroacetic acid (99%, Sigma-Aldrich), sodium trifluoroacetate (>99%, Sigma-Aldrich), yttrium(III) oxide (99.99%, Sigma-Aldrich), ytterbium(III) oxide (99.9%, Sigma-Aldrich), erbium(III) oxide (99.9%, Sigma-Aldrich), thulium(III) oxide (99.9%, Sigma-Aldrich), 1-octadecene (>95%, Sigma-Aldrich), oleic acid (99%, Chempur), cyclohexane (99%, Chempur), ethanol (99.8%, Chempur), polyoxyethylene(5)nonylphenylether IGEPAL CO-520 (Sigma-Aldrich), ammonium hydroxide solution (30%, Sigma-Aldrich) and tetraethyl orthosilicate TEOS (98%, Sigma-Aldrich) were used without further purification.
2.2. Synthesis of β-NaYF4:20%Yb3+,2%Er3+ and β-NaYF4:20%Yb3+,0.2%Tm3+ nanoparticles
The upconverting NPs were synthesized by homogeneous solution coprecipitation method. The nanoparticles were prepared from precursors (trifluoroacetate salts of yttrium, ytterbium, and erbium or thulium). The trifluoroacetate salts were chemically prepared according to method reported previously.40
The main synthesis was carried out at non-oxide waterless reaction environment using solution of oleic acid and octadecene, at 330 °C for 30 minutes.41
2.3. Synthesis of β-NaYF4:20%Yb3+,2%Er3+@SiO2 nanoparticles
The β-NaYF4:20%Yb3+,2%Er3+ NPs were surface coated with silica to improve their functionality. Solution of NPs suspended in cyclohexane was mixed with IGEPAL CO-520 and the mixture was sonicated for 10 min. Next ammonia was added dropwise and stirred for 30 min. Then, TEOS was added and the solution was stirred for 24 h at room temperature. The NPS was cleaned by mixture of ethanol/water solution (1
:
1) five times after the reaction.42
2.4. Characterization of NaYF4:20%Yb3+,2%Er3+, NaYF4:20%Yb3+,0.2%Tm3+, and NaYF4:20%Yb3+,2%Er3+@SiO2 nanoparticles
The size distribution and morphology of both β-NaYF4:20%Yb3+,2%Er3+ and β-NaYF4:20%Yb3+,0.2%Tm3+ NPs were assessed by scanning electron microscopy (SEM) using a Zeiss Auriga Neon 40 microscope at an acceleration voltage of 5 kV. For SEM imaging samples were prepared by dropping the NPs' solution onto the surface of a 0.5 × 0.5 cm2 silicon wafer. The presence of the SiO2 shell was confirmed using high-resolution transmission electron microscopy (HRTEM). Elemental analysis of the NPs was performed using the energy-dispersive spectroscopy (EDX) accessory connected to the TEM system (FEI Tecnai Osiris operating at 80 kV acceleration voltage).
The crystal structure of the NPs was determined using TEM and X-ray diffraction (XRD). XRD measurements were conducted in a Philips X'Pert Pro Alpha1 MPD (Panalytical) diffractometer in the 2θ range of 10–150° (15 h pattern scanning at 1.5406 nm wavelength).
The upconversion luminescence of the NPs was measured in an optical system comprising a 980 nm continuous wave (CW) laser (Lumics model LU0980D300-DNA014) as the optical excitation source and a Jobin Yvon-SPEX 270 M monochromator equipped with a CCD camera. Luminescence spectra were measured in the 500 to 700 nm range. A shortpass filter cut-off 750 nm (ThorLabs FESH0750) was employed while recording the luminescence data.
2.5. Incubation of HeLa cells with β-NaYF4:20%Yb3+,2%Er3+ and β-NaYF4:20%Yb3+,2%Er3+@SiO2 nanoparticles
The standard HeLa cell line derived from cervical cancer was used in this study. The HeLa cells were routinely cultured with DMEM (Dulbecco's modified eagle medium) containing 10% fetal calf serum (FCS). Cell cultures were kept at 37 °C in a humidified atmosphere containing 5% of CO2. Cells were cultured in 6-well plate dishes (6 × 10 cm2) at a density of 100
000 per plate. The cells were incubated with β-NaYF4:20%Yb3+,2%Er3+ NPs without oleic acid (procedure describe in Section 2.4.1) and with β-NaYF4:20%Yb3+,2%Er3+@SiO2 NPs. 291 μl of the sonicated initial aqueous colloidal suspension of β-NaYF4:20%Yb3+,2%Er3+ NPs (3.44 mg ml−1) was dissolved in 709 μl miliR water (the initial aqueous colloidal suspension of β-NaYF4:20%Yb,2%Er@SiO2 NPs contained 3.75 mg ml−1 and 267 μl of this sample was sonicated with 733 μl MiliR water to obtain 1 mg ml−1 concentration). Next, 2 μl of each diluted suspension was added to a 10 cm2 dish with HeLa cells and incubated for 24 h. Then, the medium was changed to DMEM.43
2.6. Procedure of ligand-free β-NaYF4:20%Yb3+,2%Er3+ nanoparticles preparation
Cyclohexane suspension of the NPs was precipitated by ethanol and washed by several cycles of sonication and centrifugation. Removal of oleic acid ligand took place through the using of different solution to dissolve NPs such as: HCl solution (0.1 M), acidic ethanol solution (pH 4), ethanol. Finally, the NPs were re-dispersed in 1 ml distilled water.44,45
2.7. Observation of β-NaYF4:20%Yb3+,2%Er3+ and β-NaYF4:20%Yb3+,2%Er3+@SiO2 nanoparticles inside the HeLa cells
The main technique for imaging both β-NaYF4:20%Yb3+,2%Er3+ and β-NaYF4:20%Yb3+,2%Er3+@SiO2 NPs was confocal microscopy, using a Zeiss 710 NLO system equipped with an infrared femtosecond laser (Coherent, Chameleon). The three channels were observed: the first, with excitation at 980 nm (femtosecond laser), was used for NP imaging in the 500–730 nm range; the second, with excitation at 705 nm (femtosecond laser), was used for imaging the nucleus marked by the Hoechst marker while detecting in the 425–475 nm range; the third, with excitation at 488 nm (continuous laser), was used to image the actine filaments build cell's cytoskeleton marked by antibodies labelled with fluorophore AlexaFluor 488 imaging with detection in the 495–572 nm range. This approach allows for imaging the marked structures and the UCNPs inside the selected cells.
Samples for confocal microscopy imaging were prepared according to the following procedure. Cells were inoculated onto 2 × 2 cm2 slides and placed in the 6-well plate at 100
000 per plate. After 24 h incubation materials were separated by sonication and transfected as previously described (Section 2.4). Then, the medium was removed and the cells were washed twice with 2 ml of phosphate-buffered saline (PBS). Firstly, the cells were fixed by adding 2 ml of 3.7% formaldehyde in PBS and incubated for 20 min. After fixing, the cells were washed twice using 2 ml of PBS. Secondly, the cell's membrane was permeabilizated using 2 ml of 1% solution of TritonX-100 in 5% FBS/PBS (5% solution of Fetal Bovine Serum in PBS) and incubated for 10 min. Next, the cells were washed twice with 2 ml of PBS and permeabilization was blocked by adding 2 ml of 5% FBS/PBS and the samples were incubated for 30 min. Samples were washed with PBS and the cytoskeleton was stained with the primary rabbit actine antibody. 25 μl of the antibody in 5% FBS/PBS solution was dropped onto the slide with cells and covered with a capping slide. Samples were incubated for 1 h and then the capping slide was removed. The samples were washed twice using PBS. Using the same protocol the secondary antibody connected with AlexaFluor 488 was attached to the primary antibody. This step of preparation was conducted in dark to protect the fluorescent dye from photobleaching. The nucleus was marked using the Hoechst 33342 (25 μL) added.
2.8. Cytotoxicity of β-NaYF4:20%Yb3+,2%Er3+ and β-NaYF4:20%Yb3+,2%Er3+@SiO2 nanoparticles
The cytotoxicity of the β-NaYF4:20%Yb3+,2%Er3+ and β-NaYF4:20%Yb3+,2%Er3+@SiO2 NPs was evaluated by the MTT viability assay. The cells (10
000 per well) were incubated in each well of a 96-well plate with the β-NaYF4:20%Yb3+,2%Er3+ and β-NaYF4:20%Yb3+,2%Er3+@SiO2 NPs in the following concentrations: 0.1, 1, 5, 10 and 50 μg ml−1 in medium for 24 and 48 h. Following incubation, the cells were washed three times with the culture medium. Then, the MTT solution (5.0 mg ml−1) was added. After incubation for another 4 h the MTT medium was removed from each well, DMSO was added and the mixture was shaken at room temperature to dissolve the reacted dye. The optical density (OD) was measured at 570 nm with a microplate reader. The cytotoxicity was calculated as follows: cytotoxicity = (B × 100%)/A, where A is the absorbance of the cells incubated with the culture medium (as a control) and B is the absorbance of the cells incubated with or without the NPs.
2.9. Transmission electron microscopy of the β-NaYF4:20%Yb3+,2%Er3+ and β-NaYF4:20%Yb3+,2%Er3+@SiO2 nanoparticles inside the HeLa cells
The presence of the NPs' inside the HeLa cells was confirmed by TEM imaging. Upon incubation with 1 μg ml−1 of β-NaYF4:20%Yb3+,2%Er3+ or β-NaYF4:20%Yb3+,2%Er3+@SiO2 NPs for 24 h HeLa cells pellets were fixed with 2% paraformaldehyde (Sigma Aldrich, Germany) and 2.5% glutaraldehyde (Merck KGaA, Darmstadt, Germany) in 0.1 M cacodylate buffer, pH = 7.4 at 4 °C for 2 h, post-fixed with 1% osmium tetroxide (Sigma, USA) for 1 h, dehydrated in the graded series of ethanol (from 30% to 99.8%) and propylene oxide (ABCR GmbH, Germany), embedded in Agar 100 resin kit R1031 (Agar, USA) and polymerized at 60 °C for 24 h and sectioned (60 nm) using a RMC ultramicrotome (USA). The ultrathin sections were stained with uranyl acetate and lead citrate. Grids were examined with a JEM 1200EX electron microscope.
2.10. Reactive oxygen species generation by β-NaYF4:20%Yb3+,0.2%Tm3+@SiO2 nanoparticles
The potential application of the presented nanoparticles to generate ROS was proved by electron paramagnetic resonance spectroscopy (EPR) after NIR light excitation. Water solution of NPs at 15 mg ml−1 concentration was mixed with spin trap buffer, as a spin trap used 1-hydroxy-3-methoxycarbonyl-2,2,5,5-tetramethylpyrrolidine (CMH), which shows EPR signal after reaction with the radicals.
The experiment was performed using as a near infrared sources: prototype LEDs system with maximum emission by 980 nm developed at University of Brasília, which worked with following parameters: 1 W cm−2 of power density, 30 minutes of irradiation (working cycle: 30 seconds of irradiation, 1 minute break). EPR signal was measured every ten minutes during 20 minutes of irradiation using Bruker EMX plus EPR spectrometer with X-Band using a 4119HS resonator with 5 G modulation, 200 G sweep width, and 20 mW microwave power. The ROS production was performed by spin trapping using by CMH cyclic hydroxylamine and Krebs Hepes buffer, with controlled time to air exposure to avoid false positives results.
3 Results and discussion
The structural properties and the phase purity of the β-NaYF4:20%Yb3+,2%Er3+ and β-NaYF4:20%Yb3+,0.2%Tm3+ UCNPs were examined by XRD measurements. The XRD patterns of the UCNPs are shown in Fig. 1. As can be observed the diffraction peaks of both NPs are well defined and the peak positions and intensities agree well with the values for the hexagonal NaYF4 crystal.7,46
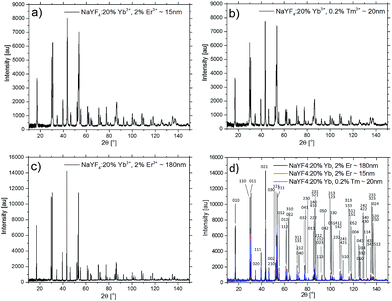 |
| Fig. 1 XRD patterns of: (a) β-NaYF4:20%Yb3+,2%Er3+ (∼15 nm of diameter); (b) β-NaYF4:20%Yb3+,0.2%Tm3+ (∼20 nm); (c) β-NaYF4:20%Yb3+,2%Er3+ (∼180 nm) and (d) all diffractograms grouped together. Note that big crystals (c) were taken as reference. | |
The size distribution of the UCNPs was determined by using XRD results and SEM images (Fig. 2a and b). From the line broadening of the (021) XRD peak of the samples β-NaYF4:20%Yb3+,2%Er3+ and β-NaYF4:20%Yb3+,0.2%Tm3+ and using the Debye–Scherrer formula an average crystallite size of 15.0 and 21.6 nm were calculated, respectively. Typical the UCNPs observed by SEM are well dispersed and very much uniform in size, with an average diameter of about 20.5 (±2.5) nm for the Er3+-doped and 22.3 (±0.9) nm for the Tm3+-doped NPs, as shown in the histograms presented in Fig. 2a and b. The average size values assessed from the SEM micrographs matched the average particle size obtained from the XRD data. Deviation in the average particle size obtained from the SEM and XRD data can be due to inaccuracy of the size estimation while using the XRD technique. The as-produced NPs have a regular spherical shape (Fig. 2), which is beneficial to their application in the cellular imaging.
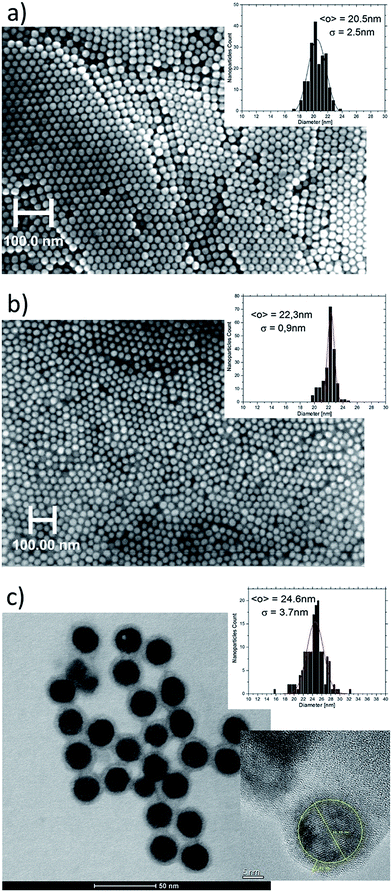 |
| Fig. 2 SEM images of (a) β-NaYF4:20%Yb3+,2%Er3+ NPs; (b) β-NaYF4:20%Yb3+,0.2%Tm3+ NPs (inserts show particle size histograms obtained from 200 counting); (c) TEM image of β-NaYF4:20%Yb3+,2%Er3+@SiO2 NPs (inserts show particle size histogram and HRTEM micrograph showing). | |
The SiO2-shell thickness and morphology of the β-NaYF4:20%Yb3+,2%Er3+@SiO2 UCNPs were assessed by TEM. Typical TEM images of the NPs are shown in Fig. 2c. After coating with SiO2, the average size of the Er3+-doped NPs increased to about 24.6 (±3.7) nm. Nevertheless, after SiO2-coating the shape and size monodispersity remained almost unchanged, as it observed on Fig. 2c, where the SiO2-shell thickness is about 3.0 nm.
The EDX data are shown in Fig. 3. The EDX spectra confirm the presence of all elements (Na, Y, Yb, Er, F) in the β-NaYF4:20%Yb3+,2%Er3+ and (Na, Y, Yb, Tm, F) in the β-NaYF4:20%Yb3+,0.2%Tm3+ NPs (Fig. 3a and b). EDX maps show homogenous distribution of the elements forming the core and the shell of the NPs. Fig. 3c presents core, β-NaYF4:20%Yb3+,2%Er3+ NPs and Fig. 3d shows the SiO2 shell on the NPs.
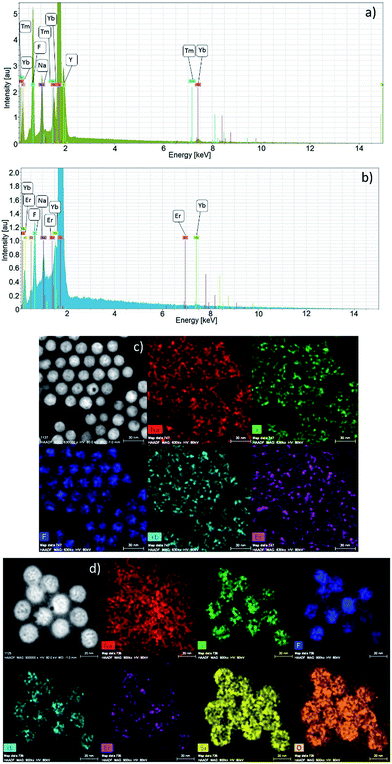 |
| Fig. 3 EDX spectra of the (a) β-NaYF4:20%Yb3+,0.2%Tm3+ and (b) β-NaYF4:20%Yb3+,2%Er3+ NPs. EDX elemental distribution maps of the (c) β-NaYF4:20%Yb3+,2%Er3+ NPs and (d) β-NaYF4:20%Yb3+,2%Er3+@SiO2 NPs. | |
The EDX data in Fig. 3 reviled homogeneous distribution of all elements in both types of the NPs. Likewise, a homogeneous elemental distribution is observed in the β-NaYF4:20%Yb3+,2%Er3+@SiO2 NPs (see Fig. 3d). Silicon and oxygen are in the same location as other elements within the NPs. Indeed, silica forms a shell around the NPs which is visible in the Si EDX map in Fig. 3d. This finding confirms that the NPs were efficiently coated by SiO2.
Before the cytotoxicity measurements of β-NaYF4 NPs, the oleic acid was detached from the surface. Actually, the oleic acid acts as a surfactant capable to cap ligands while introducing the hydrophobic character to the NPs. Therefore, the oleic acid-coated UCNPs are not suitable for biological application, but can be easily removed out from the NP surface by HCl washing while the remaining functional groups associated with oleic acid can be identified by FT-IR spectroscopy (Fig. 4).
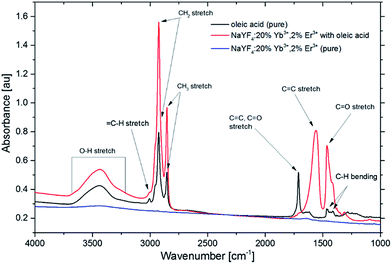 |
| Fig. 4 FT-IR spectra of oleic acid, β-NaYF4:20%Yb3+,2%Er3+ NPs with oleic acid and β-NaYF4:20%Yb3+,2%Er3+ NPs after HCl washing. | |
The oleic acid exhibits an IR band around 3430 cm−1, corresponding to the hydroxyl group's stretching vibration. Two features peaking at 1632 and 1460 cm−1 are associated with the asymmetric and symmetric stretching vibrations of the carboxylic group (COO−), respectively. The two peaks at 2924 and 2854 cm−1 can be assigned to the asymmetric and symmetric stretching vibrations of methylene group, respectively. As shown in Fig. 4, after the HCl purification, the quenching of the features in the FT-IR spectrum in the 4000–1000 cm−1 range indicates that the pure (oleic acid free) NaYF4:20%Yb3+,2%Er3+ NPs were obtained.
The upconversion spectra of 1 mg ml−1 suspensions of β-NaYF4:20%Yb3+,2%Er3+ and β-NaYF4:20%Yb3+,0.2%Tm3+ NPs in cyclohexane under 980 nm laser diode excitation (power density 12.14 W cm−2) are shown in Fig. 5a and d. The insets of Fig. 5a and d show a digital photograph of the total upconversion luminescence of the same solution under the described excitation condition.
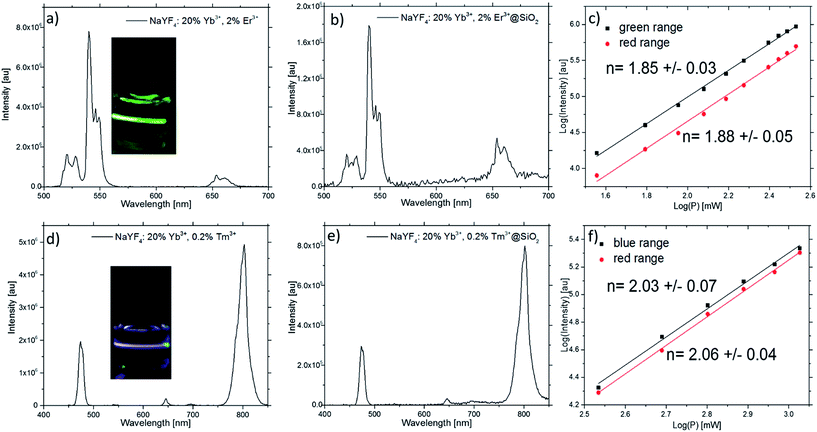 |
| Fig. 5 Luminescence spectra of the NPs suspensions (1 mg ml−1) in cyclohexane excited with a 980 nm laser diode (power density 12.14 W cm−2): (a) β-NaYF4:20%Yb3+,2%Er3+, (d) β-NaYF4:20%Yb3+,0.2%Tm3+ and 1 mg ml−1 in water suspension of (b) β-NaYF4:20%Yb3+,2%Er3+@SiO2 aand (e) β-NaYF4:20%Yb3+,0.2%Tm3+@SiO2 NPs excited with a 980 nm laser diode (power density 12.14 W cm−2). Double log plot of power dependence of the upconverted emissions of 1 mg ml−1 suspensions of the NPs in cyclohexane excited at 980 nm: (c) β-NaYF4:20%Yb3+,2%Er3+ and (f) β-NaYF4:20%Yb3+,0.2%Tm3+. Symbols are experimental data while the straight lines are least-squares fits to the data points. | |
In order to determine the number of photons responsible for the upconversion mechanism the intensity of the upconversion emission (UCL) was recorded as a function of the 980 nm excitation (Fig. 5c and f).
Stable suspension of the NPs in aqueous medium is very important for effective bioimaging applications. Therefore, for the biological applications the silica-coated NPs (β-NaYF4:20%Yb3+,2%Er3+@SiO2) were produce. As a result of surface modification the β-NaYF4:20%Yb3+,2%Er3+@SiO2 NPs are stable in water and emit strong upconverting fluorescence (Fig. 5b and e).
The emission bands of the β-NaYF4:20%Yb3+,2%Er3+ and the β-NaYF4:20%Yb3+,0.2%Tm3+ NPs after NIR light excitation are shown in Fig. 5a and d. They can be assigned to the transitions between the 4f–4f levels of the Er3+ and Tm3+ ions. The spectrum of the β-NaYF4:20%Yb3+,2%Er3+ NPs (see Fig. 5a) exhibits three emission bands. The two strong green emissions at 540 and 550 nm originate from the 2H11/2 to 4I15/2 and from 4S3/2 to 4I15/2 transitions, respectively. The red emission at 654 nm can be assigned to the transition from 4F9/2 to 4I15/2 levels.47,48
Four Tm3+ emission bands were observed in the β-NaYF4:20%Yb3+,0.2%Tm3+ NPs (Fig. 5d) with maximum position of peaks at 474, 645, 697 and 800 nm.
In order to determine the number of photons responsible for the upconversion mechanism, the intensity of the upconversion luminescence (UCL) was recorded as a function of the power of 980 nm excitation (see Fig. 5c and f). The UCL intensity (Iup) in the lanthanide-doped UCNPs depends on the laser power (P) excitation. This dependence can be expressed as: Iup ∼ Pn, where n describes the quantity of absorbed photons in the luminescence process. Fig. 5c shows the green and red Er3+ upconversion emission intensities in quadratic power dependence at low excitation, indicating the two-photon upconversion mechanisms. For the Tm3+-doped NPs (see Fig. 5f) three- and two-photon power dependencies were observed for the 1G4 to 3H6 and 3H4 to 3H6 emissions. Similar observation has been reported previously in the literature.47
The upconversion mechanisms for the Er3+/Yb3+ and Tm3+/Yb3+ ion couples within the β-NaYF4 crystal are well-known49 and schematically represented in Fig. 6. In the case of β-NaYF4:20%Yb3+,2%Er3+ the excitation energy is transferred from the Yb3+ ion in the 2F5/2 state to the Er3+ ion in the 4I11/2 state. The second energy transfer from the Yb3+ ion can populate the 4F7/2 state of the Er3+ ion. The Er3+ ion can relax non-radiative (without emission of photons) to the 2H11/2 and 4S3/2 levels before emitting green light via 2H11/2 → 4I15/2 and 4S3/2 → 4I15/2 transitions. Alternatively, the Er3+ ion can relax and populate the 4F9/2 level, leading to the red 4F9/2 → 4I15/2 emission. The 4F9/2 level may also be populated from the 4I13/2 level of the Er3+ ion by absorption of a 980 nm photon, or by energy transfer from an Yb3+ ion, with the 4I13/2 state being initially populated via the non-radiative 4I11/2 → 4I13/2 relaxation.47
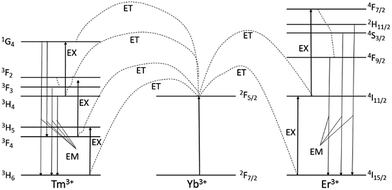 |
| Fig. 6 The energy level diagrams of the Er3+, Tm3+ and Yb3+ dopant ions and the corresponding upconversion mechanisms under 980 nm excitation. The ET interrupted arrows represent energy transfer (non-radiative transition), EX – excitation and EM – emission of visible photons (radiative transition).51–53 | |
Nanoparticles doped by Tm3+ ions are characterized by four main emission bands (see Fig. 5d) with wavelength 474, 645, 697 and 800 nm. After absorption of 980 nm by Yb3+ ions, energy is transferred from 2F5/2 ytterbium state to 3H6, 3H5, 3F2 and 1G4 states, respectively. In consequence of energy transfers and non-radiative transitions between the levels followed the emission from states: 1G4 → 3H6, 1G4 → 3F4, 3F3 → 3H6 and 3H4 → 3H6 respectively. These emission bands are described above (Fig. 6).52,53
As shown in Fig. 5a and e presence of the SiO2 shell, has an impact to the physical aspect of the luminescence. Analysis of the core NaYF4:20%Yb3+,2%Er3+ NPs emission spectrum points to the higher luminescence intensity of the green area compared to the red area. The ratio of the two luminescence areas has different characteristic for the NPs coated by SiO2. In this material the intensity of the green luminescence is lower than the red. This difference is due to the modification of the NPs surface which offsets the surface effects and contribute to the energy of phonons relaxation, which takes part in the upconversion, as we reported previously.54 The silicon oxide shell enhances the non-radiative decay which causes higher quantity of the radiative emission from 4F9/2 level than 4S3/2 level (see Fig. 6).
We have synthesized and characterized β-NaYF4:20%Yb3+,0.2%Tm3+@SiO2 nanoparticles exhibiting several promising properties useful in medical applications: bio imagining of cells and tissues and modern PDT. A high efficiency NIR upconversion and, due to presence of Tm3+–Yb3+ pair ions, ROS generation capability, renders the SiO2 shell coated nanoparticles to become potentially useful theranostic agent.
The experiment, performed under standard conditions, was aimed to test the possibility of β-NaYF4:20%Yb3+,0.2%Tm3+@SiO2 NPs to generate ROS without any additional organic molecules. Comparison of the control sample-without the LED irradiation and the NPs suspension exposed to the light, shows enhanced level of the CMH spin trap signal. Intensity of the EPR signals for the control and LED illuminated samples were summarized (Fig. 7). We observed the 70% increased in the EPR signal after 10 min of irradiation and 70% after 20 min of irradiation. The ROS generation from Tm-doped nanoparticles is potentially promising for the future PDT therapy.
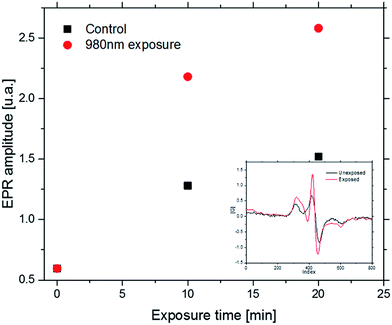 |
| Fig. 7 EPR spectroscopy graph of CMH signal. Spin trap was activated by reactive oxygen species from decomposition of water by NPs emitted high energy light. Control group shows non irradiated solution. Tested group was irradiated during 30 minutes with 980 nm NIR sources at 1 W cm−2 power density, respectively by LEDs system. Insert shows example spectrum (after 20 minute of irradiation) using to make a graph. | |
In order to investigate the cytotoxicity of both β-NaYF4:20%Yb3+,2%Er3+ (without oleic acid) and β-NaYF4:20%Yb3+,2%Er3+@SiO2 NPs a MTT assay, using HeLa cell line, was performed to determine the effect of the UCNPs on the cell proliferation after 24 and 48 h (Fig. 8).
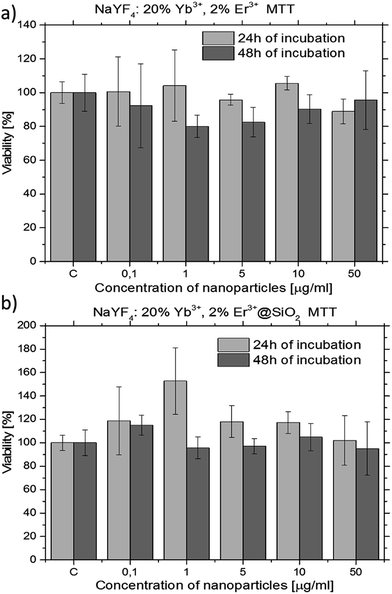 |
| Fig. 8 Cell viability values estimated from the MTT assay versus concentration of the β-NaYF4:20%Yb3+,2%Er3+ NPs without oleic acid (a) and β-NaYF4:20%Yb3+,2%Er3+@SiO2 NPs (b). Cells were incubated with 0–50 μg ml−1 of the NPs at 37 °C, for 24 h (a, c) and 48 h (b, d). Each data point is represented as mean (SD from 4 trials). | |
One of the most important aspect in the design and the synthesis of biomarkers is their toxicity. In order to investigate the cytotoxicity of β-NaYF4:20%Yb3+,2%Er3+ (without oleic acid) and β-NaYF4:20%Yb3+,2%Er3+@SiO2 NPs a MTT assay was used. No significant difference in the cell proliferation was observed in the absence or presence of 0.1–50 μg ml−1 UCNPs (see Fig. 8). The cellular viabilities were estimated to be greater than 90% after 24 h and 80% after 48 h for the β-NaYF4:20%Yb3+,2%Er3+ NPs (Fig. 8a) and almost 100% after 24 and 48 h for the β-NaYF4:20%Yb3+,2%Er3+@SiO2 NPs (Fig. 8b). These data show that the β-NaYF4:20%Yb3+,2%Er3+ NPs have relatively low cytotoxicity after 24 and 48 h of incubation even at relatively high concentration (50 μg ml−1). The β-NaYF4:20%Yb3+,2%Er3+@SiO2 NPs have no apparent cytotoxicity (after 48 h of incubation, the cellular viabilities were 100% even at the NPs concentration of 50 μg ml−1).
Cellular uptake of both β-NaYF4:20%Yb3+,2%Er3+ (without oleic acid) and β-NaYF4:20%Yb3+,2%Er3+@SiO2 NPs by HeLa cells was visualized by multiphoton confocal imaging (Fig. 9a and b). Cells stained by the NPs were exposed to 980 nm irradiation using a femtosecond Ti:sapphire pulsed laser while their typical upconversion luminescence was measured in 500–730 nm channel for the green and red emissions. In addition, the cytoskeleton of the HeLa cells was stained by the antibodies conjugated with the AlexaFluor 488 for a better visualization of the cells. The blue color indicates the nucleus stained by Hoechst 33342 channel (excitation: 705 nm – femtosecond laser, power density: 0.72 W μm−2, detection: 425–475 nm). Green color indicates the actine cytoskeleton filaments marked by the antibody labeled with AlexaFluor 488 (excitation: 488 nm – CW, power density: 0.0375 W μm−2, detection: 495–572 nm). Red color indicates the UCNPs channel (excitation: 980 nm – femtosecond laser, power density: 1.11 W μm−2; detection: 500–730 nm). A spectrum recorded from the cells showed that the luminescence exhibits two emission bands, with maxima at 556 and 653 nm after 980 nm of excitation (Fig. 9c and d). Fig. 9e and f shows the TEM images of (e) SiO2-uncoated and (f) SiO2-coated NPs (without oleic acid) inside the HeLa cells.
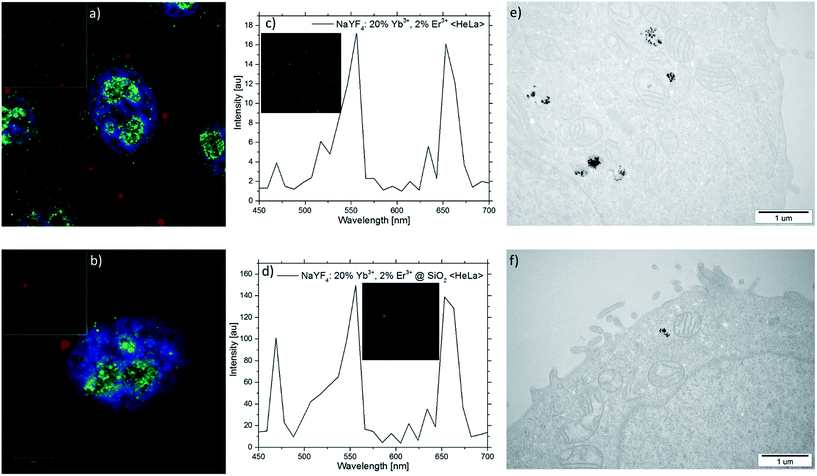 |
| Fig. 9 Confocal images of the HeLa cells incubated with the (a) β-NaYF4:20%Yb3+,2%Er3+ and (b) β-NaYF4:20%Yb3+,2%Er3+@SiO2 NPs after 24 h of incubation with 0.001 mg ml−1 of the NPs concentration. The insets in the panels (a) and (b) show the upconversion channels. Luminescence spectra of the (c) β-NaYF4:20%Yb3+,2%Er3+ and (d) β-NaYF4:20%Yb3+,2%Er3+@SiO2 NPs inside the HeLa cells excited with the 980 nm wavelength light at 1.11 W μm−2 laser power. Insets in (c) and (d) show images with luminescent spots. TEM images showing the (e) β-NaYF4:20%Yb3+,2%Er3+ and (f) β-NaYF4:20%Yb3+,2%Er3+@SiO2 NPs within HeLa cells (incubation with 1 μg ml−1 of NPs at 37 °C, for 24 h). | |
The overlay of the upconversion channel and cytoskeleton channel indicate that the UCL signal distributions are strongly correlated with the cytoskeleton of HeLa cells, meaning that the NPs spontaneously enter into the cells and locate within the cytoplasm. In contrast, the HeLa cells incubated with the β-NaYF4:20%Yb3+,2%Er3+@SiO2 NPs (Fig. 9b) show weaker luminescence in the upconverting green channel under the same conditions, suggesting a lower uptake by the HeLa cells. This may be due to differences in the NP surface charge. The spectrum recorded from the HeLa cells shows that the luminescence exhibits two characteristic emission bands, with maxima at 556 and 653 nm (Fig. 9d).
It should be pointed out that no measurable autofluorescence signal was observed after 980 nm excitation in the upconverting channel of the HeLa cells labelled with both β-NaYF4:20%Yb3+,2%Er3+ and β-NaYF4:20%Yb3+,2%Er3+@SiO2 NPs (insets of Fig. 9a and b). Moreover, in the upconverting channel a high signal-to-noise ratio, with relatively high upconversion intensity, was observed and no background fluorescence was noted. As far as the biological imaging is concerned, the finding is an interesting and useful feature of the UCNPs,50 which has not been obtained in the traditional single-photon or two-photon fluorescent imaging.55–66 While excited with the 980 nm laser light the biological samples presented weak absorption with no luminescence in the visible region. Fig. 9e and f shows that SiO2-coated and SiO2-uncoated NPs (without oleic acid) were trapped within cytosolic vesicular structures after being up-taken by the HeLa cells, suggesting that the NPs' cellular internalization took place by endocytosis in both cases.
4 Conclusions
The present report describes a successful synthesis and characterization of the β-NaYF4:20%Yb3+,2%Er3+ and β-NaYF4:20%Yb3+,0.2%Tm3+ NPs. Under NIR light irradiation the β-NaYF4:20%Yb3+,2%Er3+ NPs exhibited intense green and red luminescence while the β-NaYF4:20%Yb3+,0.2%Tm3+ NPs exhibited intense ultra-violet, blue and red luminescence. UV emission range is capable to generate reactive oxygen species in aqueous environment. Compared to traditionl therapies based on ROS generation, we presented materials which could generate ROS without any other components attached to the nanoparticles. This property allows to solve a common problems with traditional systems containing photosensitizers (photobleaching, dose limitation). The synthesized core NPs were surface coated with silica, having the silica shell thickness about 3 nm. The nanoparticle's core/shell morphology, its structure and surface modification were confirmed by XRD, TEM, SEM, EDX, and FTIR measurements. All synthesized nanomaterials formed stable aqueous colloids exhibiting green or blue luminescence under NIR laser irradiation (λex = 980 nm). The cytotoxicity assays revealed that all the prepared nanomaterials are relatively nontoxic even in relatively high concentration (50 μg ml−1) for 48 h incubation. The β-NaYF4:20%Yb3+,2%Er3+ and β-NaYF4:20%Yb3+,2%Er3+@SiO2 NPs were visualised inside the cytoplasm of HeLa cells. Moreover, no apparent background fluorescence was observed inside the HeLa cells after the NIR light excitation with the strong up-conversion of the nanoparticles. Our report shows several promising applications of the inorganic, lanthanide-doped nanophosphors, in particularly, as potential biomarkers, contrast agents, and drug-delivery systems.
Acknowledgements
This research was partially supported by the EU within the European Regional Development Fund, through the grant Innovative Economy (POIG.01.01.02-00-008/08), the project ‘Development of the cluster center of biomedical engineering’ implemented under the Economy Operational Program (project no. UDA-POIG.05.01.00-00), the EU Research Project FP7-People-2012-IRSES-BRASINOEU (Grant Agreement Number: PIRSES-GA-2012-318916), the grants of PNSC 2013/11/B/NZ1/00089, NN UMO-2013/08/A/ST3/00297, DEC-2012/07/B/ST5/02080, and DEC-2014/15/D/ST5/02604. This work has been done in the NanoFun laboratories co-financed by the European Regional Development Fund within the Innovation Economy Operational Program (Project No. POIG.02.02.00-00-025/09). This research was also co-financed by the Swiss National Science Foundation through the Nano-Tera.ch Focused Project (NTF), ‘NanoUp’. The use of the FEI Tecnai Osiris TEM instrument located at the Facility for Electron Microscopy & Sample Preparation of the University of Rzeszow is acknowledged.
Notes and references
- R. A. Jalil and Y. Zhang, Biocompatibility of Silica Coated NaYF4 Upconversion Fluorescent Nanocrystals, Biomaterials, 2008, 29, 4122–4128 CrossRef PubMed.
- Q. L. DeChermont, C. Chaneac, J. Seguin, F. Pelle, S. Maıtrejean, J. P. Jolivet, D. Gourier, M. Bessodes and D. Scherman, Nanoprobes with Near-Infrared Persistent Luminescence for In Vivo Imaging, Proc. Natl. Acad. Sci. U. S. A., 2007, 104, 9266–9271 CrossRef PubMed.
- J. L. West and N. J. Halas, Engineered Nanomaterials for Biophotonics Applications: Improving Sensing, Imaging and Therapeutics, Annu. Rev. Biomed. Eng., 2003, 5, 285–292 CrossRef CAS PubMed.
- M. S. Gee, R. Upadhyay, H. Bergquist, H. Alencar, F. Reynolds, M. Maricevich, R. Weissleder, L. Josephson and U. Mahmood, Human Breast Cancer Tumor Models: Molecular Imaging of Drug Susceptibility and Dosing During HER2/Neu-Targeted Therapy, Radiology, 2008, 248, 925–935 CrossRef PubMed.
- F. Auzel, Upconversion and Anti-Stokes Processes with f and d Ions in Solids, Chem. Rev., 2004, 104, 139–173 CrossRef CAS PubMed.
- X. Wang and Y. D. Li, Monodisperse Nanocrystals: General Synthesis, Assembly, and Their Applications, Chem. Commun., 2007, 28, 2901–2910 RSC.
- M. Wang, C.-C. Mi, W.-X. Wang, C.-H. Liu, Y.-F. Wu, Z.-R. Xu, C.-B. Mao and S.-K. Xu, Immunolabeling and NIR-Excited Fluorescent Imaging of HeLa Cells by Using NaYF4:Yb, Er Upconversion Nanoparticles, ACS Nano, 2009, 3(6), 1580–1586 CrossRef CAS PubMed.
- J. W. Zhao, Y. J. Sun, X. G. Kong, L. J. Tian, Y. Wang, L. P. Tu, J. L. Zhao and H. Zhang, Controlled Synthesis, Formation Mechanism, and Great Enhancement of Red Upconversion Luminescence of NaYF4:Yb3,Er3 Nanocrystals/Submicroplates at Low Doping Level, J. Phys. Chem. B, 2008, 112, 15666–15672 CrossRef CAS PubMed.
- S. Heer, K. Kompe, H. U. Gudel and M. Haase, Highly Efficient Multicolour Upconversion Emission in Transparent Colloids of Lanthanide-Doped NaYF4 Nanocrystals, Adv. Mater., 2004, 16, 2102–2105 CrossRef CAS.
- O. Ehlert, R. Thomann, M. Darbandi and T. Nann, A Four-Color Colloidal Multiplexing Nanoparticle System, ACS Nano, 2008, 2, 120–124 CrossRef CAS PubMed.
- H. Kolarova, P. Nevrelova, K. Tomankova, P. Kolar, R. Bajgar and J. Mosinger, Production of reactive oxygen species after photodynamic therapy by porphyrin sensitizers, Gen. Physiol. Biophys., 2008, 27, 101–105 CAS.
- X.-F. Qiao, J.-C. Zhou, J.-W. Xiao, Ye-F. Wang, L.-D. Sun and C.-H. Yan, Triple-functional core–shell structured upconversion luminescent nanoparticles covalently grafted with photosensitizer for luminescent, magnetic resonance imaging and photodynamic therapy in vitro, Nanoscale, 2012, 4, 4611–4623 RSC.
- Y. Guo, S. Rogelj and P. Zhang, Rose Bengal-decorated silica nanoparticles as photosensitizers for inactivation of gram-positive bacteria, Nanotechnology, 2010, 21, 065102 CrossRef PubMed.
- G. S. Yi and G. M. Chow, Synthesis of Hexagonal-Phase NaYF4: Yb, Er and NaYF4:Yb, Tm Nanocrystals with Efficient Up- Conversion Fluorescence, Adv. Funct. Mater., 2006, 16, 2324–2329 CrossRef CAS.
- Y. Wei, F. Q. Lu, X. R. Zhang and D. P. Chen, Synthesis of Oil-Dispersible Hexagonal-Phase and Hexagonal-Shaped NaYF4:Yb,Er Nanoplates, Chem. Mater., 2006, 18, 5733–5737 CrossRef CAS.
- L. Y. Wang and Y. D. Li, Controlled Synthesis and Luminescence of Lanthanide Doped NaYF4 Nanocrystals, Chem. Mater., 2007, 19, 727–734 CrossRef CAS.
- H. C. Lu, G. S. Yi, S. Y. Zhao, D. P. Chen, L. H. Guo and J. Cheng, Synthesis and Characterization of Multi-Functional Nanoparticles Possessing Magnetic, Up-Conversion Fluorescence and Bio-Affinity Properties, J. Mater. Chem., 2004, 14, 1336–1341 RSC.
- F. Wang and X. G. Liu, Advances in the chemistry of lanthanide-doped upconversion nanocrystals, Chem. Soc. Rev., 2009, 38, 976–989 RSC.
- G. K. Das, B. C. Heng, S. C. Ng, T. White, J. S. C. Loo, L. D'Silva, P. Padmanabhan, K. K. Bhakoo, S. T. Selvan and T. T. Y. Tan, Gadolinium oxide ultranarrow nanorods as multimodal contrast agents for optical and magnetic resonances imaging, Langmuir, 2010, 26, 8959–8965 CrossRef CAS PubMed.
- D. K. Yi, S. T. Selvan, S. S. Lee, G. G. Papaefthymiou, D. Kundaliya and J. Y. Ying, Silica-coated nanocomposites of magnetic nanoparticles and quantum dots, J. Am. Chem. Soc., 2005, 127, 4990–4991 CrossRef CAS PubMed.
- Z. Y. Liu, G. S. Yi, H. T. Zhang, J. Ding, Y. W. Zhang and X. Min, Monodisperse silica nanoparticles encapsulating upconversion fluorescent and superparamagnetic nanocrystals, Chem. Commun., 2008, 694–696 RSC.
- F. Zhang, G. B. Braun, A. Pallaoro, Y. Zhang, Y. Shi, D. Cui, M. Moskovits, D. Zhao and G. D. Stucky, Mesoporous Multifunctional Upconversion Luminescent and Magnetic “Nanorattle” Materials for Targeted Chemotherapy, Nano Lett., 2012, 12, 61–67 CrossRef CAS PubMed.
- H. Ow, D. R. Larson, M. Srivastava, B. A. Baird, W. W. Webb and U. Wiesner, Bright and stable core-shell fluorescent silica nanoparticles, Nano Lett., 2005, 5, 113–117 CrossRef CAS PubMed.
- L. M. Rossi, L. Shi, F. H. Quina and Z. Rosenzweig, Stober synthesis of monodispersed luminescent silica nanoparticles for bioanalytical assays, Langmuir, 2005, 21, 4277–4280 CrossRef CAS PubMed.
- A. Gnach, T. Lipinski, A. Bednarkiewicz, J. Rybka and J. A. Capobianco, Upconverting nanoparticles: assessing the toxicity, Chem. Soc. Rev., 2015, 44, 1561 RSC.
- S. Sivakumar, P. R. Diamente and F. C. J. M. van Veggel, Silica-Coated Ln3+-Doped LaF3 NanoparticlesasRobus t Down- and Upconverting Biolabels, Chem.–Eur. J., 2006, 12, 5878–5884 CrossRef CAS PubMed.
- F. Wang, X. Yang, L. Ma, B. Huang, N. Na, Y. E, D. He and J. Ouyang, Multifunctional up-converting nanocomposites with multimodal imaging and photosensitization at near-infrared excitation, J. Mater. Chem., 2012, 22, 24597–24604 RSC.
- K. Liu, X. Liu, Q. Zeng, Y. Zhang, L. Tu and T. Liu, et al., Covalently Assembled NIR Nanoplatform for Simultaneous Fluorescence Imaging and Photodynamic Therapy of Cancer Cells, ACS Nano, 2012, 6(5), 4054–4062 CrossRef CAS PubMed.
- C. Wang, L. Cheng and Z. Liu, Upconversion Nanoparticles for Photodynamic Therapy and Other Cancer Therapeutics, Theranostics, 2013, 3(5), 317–330 CrossRef PubMed.
- M. T. Jarvi, M. S. Patterson and B. C. Wilson, Insights into Photodynamic Therapy Dosimetry: Simultaneous Singlet Oxygen Luminescence and Photosensitizer Photobleaching Measurements, Biophys. J., 2012, 102(3), 661–671 CrossRef CAS PubMed.
- S. Tan, P. Yang, N. Niu, S. Gai, J. Wang, X. Jing and J. Lin, Monodisperse and core–shell structured NaYF4:Ln@SiO2 (Ln = Yb/Er, Yb/Tm) microspheres: Synthesis and characterization, J. Alloys Compd., 2010, 490, 684–689 CrossRef CAS.
- J. W. Stouwdam and F. C. J. M. van Veggel, Near-infrared emission of redispersible Er3+, Nd3+ and Ho3+ doped LaF3 nanoparticles, Nano Lett., 2002, 2, 733–737 CrossRef CAS.
- J. W. Stouwdam and F. C. J. M. van Veggel, Improvement in the Luminescence Properties and Processability of LnF3/Ln and LnPO4/Ln Nanoparticles by Surface Modification, Langmuir, 2004, 20, 11763–11771 CrossRef CAS PubMed.
- V. Sudarsan, F. C. J. M. van Veggel, R. A. Herring and M. Raudsepp, Surface Eu3+ ions are different than “bulk” Eu3+ ions in crystalline doped LaF3 nanoparticles, J. Mater. Chem., 2005, 15, 1332–1342 RSC.
- F. Vetrone, J. C. Boyer, J. A. Capobianco, A. Speghini and A. M. Bettinelli, Effect of Yb3+ Codoping on the Upconversion Emission in Nanocrystalline Y2O3:Er3+, J. Phys. Chem. B, 2003, 107, 1107–1112 CrossRef CAS.
- A. Patra, C. S. Friend, R. Kapoor and P. N. Prasad, Effect of crystal nature on upconversion luminescence in Er3+:ZrO2 nanocrystals, Appl. Phys. Lett., 2003, 83, 284–286 CrossRef CAS.
- S. Heer, K. Kompe, H.-U. Gudel and M. Haase, Highly efficient multicolour upconversion emission in transparent colloidal of nanoparticle-doped NaYF4 nanocrystals, Adv. Mater., 2004, 16, 2102–2105 CrossRef CAS.
- M. H. V. Werts, R. T. F. Jukes and J. W. Verhoeven, The emission spectrum and the radiative lifetime of Eu3+ in luminescent lanthanide complexes, Phys. Chem. Chem. Phys., 2002, 4, 1542–1548 RSC.
- K. Binnemans, R. Van Deun, C. Gorller-Walrand and J. L. Adam, Spectroscopic properties of trivalent lanthanide ions in fluorophosphates glasses, J. Non-Cryst. Solids, 1998, 238, 11–29 CrossRef CAS.
- J. E. Roberts, Lanthanum and Neodymium Salts of Trifluoroacetic Acid, J. Am. Chem. Soc., 1961, 83, 1087 CrossRef CAS.
- H.-X. Mai, Ya-W. Zhang, R. Si, Z.-G. Yan, L.-D. Sun, Li-P. You and C.-H. Yan, High-Quality Sodium Rare-Earth Fluoride Nanocrystals: Controlled Synthesis and Optical Properties, J. Am. Chem. Soc., 2006, 128, 6426–6436 CrossRef CAS PubMed.
- X.-F. Qiao, J.-C. Zhou, J.-W. Xiao, Ye-F. Wang, L.-D. Sun and C.-H. Yan, Triple-functional core–shell structured upconversion luminescent nanoparticles covalently grafted with photosensitizer for luminescent, magnetic resonance imaging and photodynamic therapy in vitro, Nanoscale, 2012, 4, 4611 RSC.
- B. Sikora, K. Fronc, I. Kamińska, K. Koper, S. Szewczyk, B. Paterczyk, T. Wojciechowski, K. Sobczak, R. Minikayev, W. Paszkowicz, P. Stępień and D. Elbaum, Transport of NaYF4:Er3C, Yb3C up-converting nanoparticles into HeLa cells, IOP Publishing, Nanotechnology, 2013, 24, 235702 CrossRef PubMed.
- F. Wang, R. Deng, J. Wang, Q. Wang, Y. Han, H. Zhu, X. Chen and X. Liu, Tuning upconversion through energy migration in core–shell nanoparticles, Nat. Mater., 2011, 10, 968–973 CrossRef CAS PubMed.
- N. Bogdan, F. Vetrone, G. A. Ozin and J. A. Capobianco, Synthesis of ligand-free colloidally stable water dispersible brightly luminescent lanthanide-doped upconverting nanoparticles, Nano Lett., 2011, 11(2), 835–840 CrossRef CAS PubMed.
- H. Schafer, P. Ptacek, H. Eickmeier and M. Haase, Synthesis of Hexagonal Yb3R,Er3R-Doped NaYF4 Nanocrystals at Low Temperature, Adv. Funct. Mater., 2009, 19, 3091–3097 CrossRef.
- J. C. Boyer, L. A. Cuccia and J. A. Capobianco, Synthesis of Colloidal Upconverting NaYF4: Er3/Yb3 and Tm3/Yb3 Monodisperse Nanocrystals, Nano Lett., 2007, 7, 847–852 CrossRef CAS PubMed.
- J. C. Boyer, F. Vetrone, L. A. Cuccia and J. A. Capobianco, Synthesis of Colloidal Upconverting NaYF4 Nanocrystals Doped with Er3,Yb3 and Tm3 ,Yb3 via Thermal Decomposition of Lanthanide Trifluoroacetate Precursors, J. Am. Chem. Soc., 2006, 128, 7444–7445 CrossRef CAS PubMed.
- R. H. Page, K. I. Schaffers, P. A. Waide, J. B. Tassano, S. A. Payne, W. F. Krupke and W. K. J. Bischel, Upconversion-pumped luminescence efficiency of rare-earth-doped hosts sensitized with trivalent ytterbium, J. Opt. Soc. Am. B, 1998, 15(3), 996–1008 CrossRef CAS.
- Q. Liu, W. Feng, T. Yang, T. Yi and F. Li, Upconversion luminescence imaging of cells and small animals, Nat. Protoc., 2013, 8, 2033–2044 CrossRef CAS PubMed.
- R. Krishnana and J. Thirumalai, Up/down conversion luminescence properties of (Na0.5Gd0.5)MoO4:Ln3+ (Ln = Eu, Tb, Dy, Yb/Er, Yb/Tm, and Yb/Ho) microstructures: synthesis, morphology, structural and magnetic investigation, New J. Chem., 2014, 38, 3480–3491 RSC.
- X. Chen, J. Vanacken, J. Han, Z. Zhong, L. Li, Y. Han, Y. Liu and V. V. Moshchalkov, Intense infrared upconversion luminescence of NaGdF4:Yb/Tm with controlled intensity, J. Appl. Phys., 2017, 121, 163103 CrossRef.
- A. Yin, Y. Zhang, L. Sun and C. Yan, Colloidal synthesis and blue based multicolor upconversion emissions of size and composition controlled monodisperse hexagonal NaYF4 : Yb,Tm nanocrystals, Nanoscale, 2010, 2, 953–959 RSC.
- I. Kamińska, K. Fronc, B. Sikora, M. Mouawad, A. Siemiarczuk, M. Szewczyk and K. Sobczak, et al., Upconverting/magnetic: Gd2O3:(Er3+,Yb3+,Zn2+) nanoparticles for biological applications: effect of Zn2+ doping, RSC Adv., 2015, 5, 78361–78373 RSC.
- G. Seisenberger, M. U. Ried, T. Endress, H. Buning, M. Hallek and C. Brauchle, Real-time single-molecule imaging of the infection pathway of an adeno-associated virus, Science, 2001, 294(5548), 1929–1932 CrossRef CAS PubMed.
- W. M. Leevy, S. T. Gammon, H. Jiang, J. R. Johnson, D. J. Maxwell and E. N. Jackson, et al., Optical imaging of bacterial infection in living mice using a fluorescent nearinfrared molecular probe, J. Am. Chem. Soc., 2006, 128, 16476–16477 CrossRef CAS PubMed.
- L. Q. Xiong, M. X. Yu, M. J. Cheng, M. Zhang, X. Y. Zhang and C. J. Xu, et al., A photostable fluorescent probe for targeted imaging of tumour cells possessing integrin avb3, Mol. BioSyst., 2009, 5, 241–243 RSC.
- M. Zhang, M. X. Yu, F. Y. Li, M. W. Zhu, M. Y. Li and Y. H. Gao, et al., A highly selective fluorescence turn-on sensor for cysteine/homocysteine and its application in bioimaging, J. Am. Chem. Soc., 2007, 129(34), 10322–10323 CrossRef CAS PubMed.
- M. X. Yu, Q. Zhao, L. X. Shi, F. Y. Li, Z. G. Zhou and H. Yang, et al., Cationic iridium(III) complexes for phosphorescence staining in the cytoplasm of living cells, Chem. Commun., 2008, 2115–2117 RSC.
- M. Bruchez Jr, M. Moronne, P. Gin, S. Weiss and A. P. Alivisatos, Semiconductor nanocrystals as fluorescent biological labels, Science, 1998, 281, 2013–2016 CrossRef CAS.
- W. C. W. Chan and S. Nie, Quantum dot bioconjugates for ultrasensitive nonisotopic detection, Science, 1998, 281(5385), 2016–2018 CrossRef CAS PubMed.
- B. Dubertret, P. Skourides, D. J. Norris, V. Noireaux, A. H. Brivanlou and A. Libchaber, In vivo imaging of quantum dots encapsulated in phospholipid micelles, Science, 2002, 298, 1759–1762 CrossRef CAS PubMed.
- A. M. Smith, H. W. Duan, A. M. Mohs and S. M. Nie, Bioconjugated quantum dots for in vivo molecular and cellular imaging, Adv. Drug Delivery Rev., 2008, 60, 1226–1240 CrossRef CAS PubMed.
- W. B. Cai, D. W. Shin, K. Chen, O. Gheysens, Q. Z. Cao and S. X. Wang, et al., Peptide-labeled near-infrared quantum dots for imaging tumor vasculature in living subjects, Nano Lett., 2006, 6, 669–676 CrossRef CAS PubMed.
- L.-Q. Xiong, Z.-G. Chen, M.-X. Yu, F.-Y. Li, C. Liu and C.-H. Huang, Synthesis, characterization, and in vivo targeted imaging of amine-functionalized rare-earth up-converting nanophosphors, Biomaterials, 2009, 30, 5592–5600 CrossRef CAS PubMed.
- D. K. Chatterjee, A. J. Rufaihah and Y. Zhang, Upconversion fluorescence imaging of cells and small animals using lanthanide doped nanocrystals, Biomaterials, 2008, 29, 937–943 CrossRef CAS PubMed.
|
This journal is © The Royal Society of Chemistry 2017 |
Click here to see how this site uses Cookies. View our privacy policy here.