DOI:
10.1039/C6RA16486B
(Paper)
RSC Adv., 2016,
6, 74765-74768
Magnetic properties and giant reversible magnetocaloric effect in GdCoC2
Received
26th June 2016
, Accepted 3rd August 2016
First published on 3rd August 2016
Abstract
The crystal structure, magnetic properties and magnetocaloric effect (MCE) of GdCoC2 have been studied. The compound crystallizes in an orthorhombic CeNiC2-type structure which belongs to the Amm2 space group. A giant reversible MCE is observed in GdCoC2 accompanied by a second-order paramagnetic to ferromagnetic (PM–FM) phase transition around the Curie temperature ∼15 K. For the magnetic field change of 0–5 T, the maximum values of the magnetic entropy change (−ΔSmaxM), relative cooling power (RCP), and refrigerant capacity (RC) are 28.4 J kg−1 K−1, 566 J kg−1 and 369 J kg−1, respectively. The present results indicate that GdCoC2 is a promising candidate for low temperature magnetic refrigeration.
1. Introduction
Materials with large/giant magnetocaloric effect (MCE) have gained more and more interest due to their applications for magnetic refrigeration. Compared with traditional gas refrigeration, magnetic refrigeration has significant advantages in conversion efficiency, low noise and environmental protection.1–5 The MCE manifests as ΔSM (isothermal magnetic entropy change) and ΔTad (adiabatic temperature change). Finding special materials with a large MCE value is considered to be the most important work of magnetic refrigeration, since the MCE is an inherent characteristic of magnetic materials. In recent years, rare-earth based compounds have been widely investigated with respect to their MCE properties.6–19 And some of them exhibit large/giant MCE at 10–25 K, which is around the boiling point of hydrogen.6–15 Considering the problems in application and storage of liquid hydrogen at room temperature, these materials are eligible to be applied for magnetic refrigeration for hydrogen liquefaction.
In the present study, we have investigated the MCE in GdCoC2 which belongs to an existing ternary system RETC2 (RE stands for heavy rare earth element and T stands for transition metal Co and Ni). Schafer et al. have reported that all the RECoC2 compounds order ferromagnetically, whereas, compounds of RENiC2 are antiferromagnetic.20–26 Up to the present, only the MCE in TbCoC2 belonging to this series compounds has been reported and the maximum magnetic entropy change (−ΔSmaxM) is 15.3 J kg−1 K−1 for the magnetic field change of 0–5 T.15 For the presently studied GdCoC2, the values of −ΔSmaxM are 16.0 J kg−1 K−1, 28.4 J kg−1 K−1, and 32.9 J kg−1 K−1 for magnetic field changes of 0–2 T, 0–5 T and 0–7 T, respectively.
2. Experimental details
The polycrystalline GdCoC2 sample was synthesized by the method of arc-melting. First, stoichiometric amounts of high-purity components were weight and 3% extra carbon was added to compensate the loss during arc-melting. The sample was turned over and melted for four times under an argon atmosphere to ensure good homogeneity. Then the sample was annealed at 900 °C for six days in evacuated quartz tubes and rapidly quenched in ice water. The sample was characterized by X-ray powder diffraction (XRD) measurement by Rigaku D/MAX 2550 using Cu Kα radiation. The magnetization measurements were done with a commercial superconducting quantum interference device (SQUID) based vibrating sample magnetometer (VSM) (Quantum Design Company, USA).
3. Results and discussion
The XRD pattern for GdCoC2 together with the Rietveld refinement profiles which were analyzed by MAUD software are shown in Fig. 1. The inset of Fig. 1 shows the crystal structure diagram obtained by using the CRYSTALMAKER software package. The factors of Rietveld refinement which were calculated by MAUD software are Rwp (%) = 8.99, RB (%) = 6.69, Rexp (%) = 12.0. The results reveal that the sample is crystallized in single phase with orthorhombic CeNiC2-type structure (Amm2 space group) and no impurity phases can be detected. According to the Rietveld refinement profiles, the refined lattice parameters a, b, and c are calculated by Bragg equation and found to be 3.621, 4.506, and 6.062 Å, respectively, which are close to the standard values.
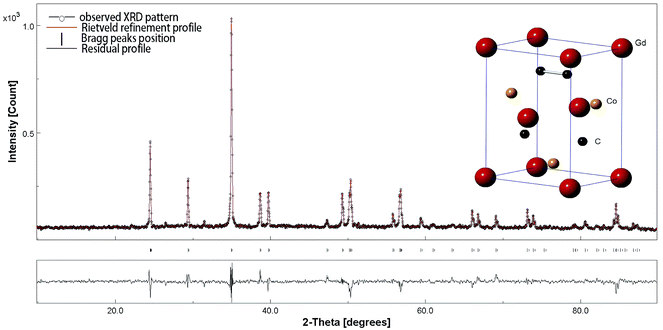 |
| Fig. 1 XRD pattern for GdCoC2 together with the Rietveld refinement profiles, Bragg peak positions, Rietveld profiles. The inset shows the crystal structure diagram. | |
The zero field cooling (ZFC) and field cooling (FC) magnetization (M) at magnetic field (H) of 0.2 T is shown in the inset of Fig. 2. A typical paramagnetic to ferromagnetic (PM–FM) phase transition can be observed at the Curie temperature (TC) ∼15 K (defined as the minimum value of dM/dT curve). No obvious difference can be observed between ZFC and FC curves, which is a typical behavior for the second order phase transition materials. The temperature dependence of magnetization (M) and the inverse susceptibility (1/χ) under magnetic field (H) of 1 T are shown in Fig. 2. At high temperature, the 1/χ–T curve shows Curie–Weiss behavior. The evaluated value of the effective magnetic moment (8.0 μB/f.u.) for GdCoC2 is close to that of the free ion value of Gd3+ (7.94 μB). A set of magnetization isotherm (M–H) was measured at temperatures from 3 K to 45 K under magnetic field up to 7 T, and several M–H curves with increasing field are shown in Fig. 3. Among them, the hysteresis cycles at 3 K, 13 K, 15 K, 20 K, and 30 K are shown in the figure as well. No obvious hysteresis is observed and the magnetization rapidly changes with varying temperature around TC, i.e. a large reversible MCE may appear around 15 K. The inset of Fig. 3 shows the M2versus H/M curves (also named as Arrot plot) from 3 K to 45 K. Based on the Banerjee criterion,27 the present GdCoC2 undergoes a second-order magnetic phase transition since neither negative slope nor inflection can be observed in the whole temperature range.
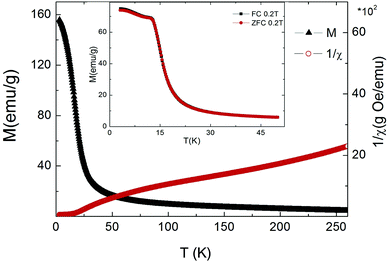 |
| Fig. 2 Temperature dependence of magnetization (left scale) and the inverse susceptibility (right scale) for GdCoC2. The inset shows the temperature dependence of zero field cooling (ZFC) and field cooling (FC) magnetization (M) under magnetic fields (H) = 0.2 T. | |
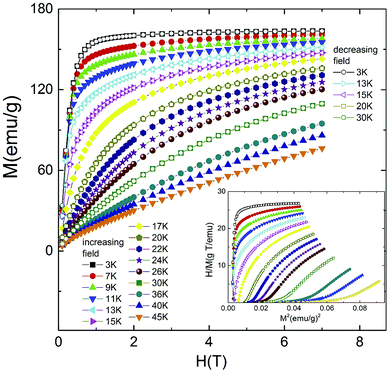 |
| Fig. 3 Magnetic field (H) dependence of the magnetization (M) for GdCoC2 at different temperatures. The inset shows the Arrot plots curves (M2versus H/M). | |
The isothermal magnetic-entropy change ΔSM is obtained from magnetization isotherms by integrating the Maxwell relation
The temperature-dependent −ΔSM under magnetic field changes up to 0–7 T is shown in Fig. 4. −ΔSM increases with the increasing value of ΔH. The maxima of −ΔSM appears around 17 K, which is close to the paramagnetic to ferromagnetic (PM–FM) phase transition. The values of −ΔSmaxM are equal to 9.3 J kg−1 K−1, 20.9 J kg−1 K−1, 28.4 J kg−1 K−1, 32.9 J kg−1 K−1 under the field changes of 0–1 T, 0–3 T, 0–5 T and 0–7 T, respectively. These values are around two times of those for TbCoC2 and larger than those of most of recently reported giant MCE materials in the same temperature range, indicating that the presently studied GdCoC2 compound belongs to a class of giant MCE materials. The origin of giant MCE in GdCoC2 is probably related to the large saturation moment, especially under low magnetic field as well as its field and temperature sensitive magnetic phase transition. Additionally, the large value of −ΔSmaxM of 16.0 J kg−1 K−1 is reached under the field changes of 0–2 T with a quite wide temperature range for present GdCoC2, which is quite beneficial to application.
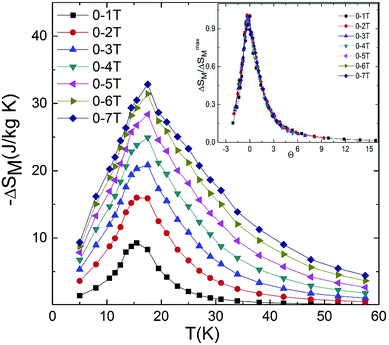 |
| Fig. 4 Temperature-dependent magnetic entropy change −ΔSM for GdCoC2 in various magnetic fields changes. The inset shows the universal curves. | |
Franco et al. revealed a universal behavior of the field dependence of ΔSM for the materials with a second-order transition, i.e. in ref. 28, the ΔSMversus T curves under different magnetic fields can collapse into a universal curve; therefore the influence of different magnetic fields can be ignored. All the curves are normalized to their respective maximum value as ΔSM/ΔSmaxM and the axis of temperature is rescaled to θ below and above TC,
Tr1 and
Tr2 are the temperatures of the two reference points of each curve that corresponds to 0.6 −Δ
SmaxM. The curves of normalized entropy change Δ
SM/Δ
SmaxMversus the rescaled temperature
θ under different magnetic fields are shown in the inset of
Fig. 4. All the curves under different magnetic fields collapse onto a single line for GdCoC
2, which further confirms that the present GdCoC
2 undergoes a second order magnetic phase transition.
The relative cooling power (RCP) and refrigerant capacity (RC) are important factors to evaluate refrigeration materials. The values of RCP can be calculated as
δTFWHM is the full width at half maximum and −Δ
SmaxM is the maximum of magnetic entropy change, respectively.
4 The values of RCP for GdCoC
2 are 216, 566, 769 J kg
−1 for Δ
H = 0–2, 0–5 and 0–7 T, respectively. The values of RC can be calculated as
T1 and
T2 are the temperatures of half-maximum value of −Δ
SM peak.
4 The value of RC for GdCoC
2 are equal to 160, 369, 514 J kg
−1 for Δ
H = 0–2, 0–5 and 0–7 T, respectively. For a comparison, the transition temperature (
TM), together with the values of −Δ
SmaxM, RCP and RC with the field change from 0 to 2 T and 5 T for GdCoC
2 and some recently reported large/giant MCE materials with
TM around 15 K are shown in
Table 1. These values for GdCoC
2 are obviously larger than most of those reported materials in the similar working temperature range.
Table 1 The transition temperature (TM), −ΔSmaxM and relative cooling power (RCP) under 2 T and 5 T for GdCoC2 and other materials with large
|
−ΔSmaxM (J kg−1 K−1) |
RCP (J kg−1) |
Reference |
T
M (K) |
2 T |
5 T |
2 T |
5 T |
GdCoC2 |
15 |
16.0 |
28.4 |
216 |
566 |
Present |
Eu8Ga16Ge30 |
11 |
4.5 |
11.5 |
80 |
400 |
7
|
GdNiBC |
15 |
9.3 |
19.8 |
188 |
474 |
8
|
HoAgAl |
18 |
3.8 |
10.3 |
99 |
344 |
10
|
ErFeSi |
22 |
14.2 |
23.1 |
∼171 |
∼486 |
29
|
GdCo2B2 |
25 |
9.3 |
17.1 |
167 |
462 |
14
|
TbCoC2 |
28 |
7 |
15.3 |
∼115 |
∼375 |
15
|
4. Conclusions
In summary, a single phase GdCoC2 compound is synthesized, and its crystal structure, magnetic properties and magnetocaloric properties have been investigated. The compound undergoes a second-order paramagnetic to ferromagnetic (PM–FM) transition at the Curie temperature TC ∼ 15 K. Accompanied with the transition, giant reversible MCE is observed. The values of −ΔSmaxM for GdCoC2 reach 16.0, 28.4, and 32.9 J kg−1 K−1 for the field changes of 0–2 T, 0–5 T and 0–7 T, respectively. The corresponding values of RCP (RC) are 216 (160), 566 (369), and 769 (514) J kg−1. For the reason that the compound has large MCE and small hysteresis in a wide temperature range, it could be considered as potential refrigerant material for low temperature magnetic refrigeration.
Acknowledgements
This work was supported by the National Natural Science Foundation of China (Grant No. 11374081) and the Fundamental Research Funds for the Central Universities (Grant No. N150905001 and N140901001).
References
- E. Bruck, J. Phys. D: Appl. Phys., 2005, 38, R381 CrossRef.
- K. A. Gschneidner, V. K. Pecharsky and A. O. Tsokol, Rep. Prog. Phys., 2005, 68, 1479 CrossRef CAS.
- B. G. Shen, J. R. Sun, F. X. Hu, H. W. Zhang and Z. H. Cheng, Adv. Mater., 2009, 21, 4545 CrossRef CAS.
- L. W. Li, Chin. Phys. B, 2016, 25, 037502 CrossRef.
- A. Smith, C. R. H. Bahl, R. Bjørk, K. Engelbrecht, K. K. Nielsen and N. Pryds, Adv. Energy Mater., 2012, 2, 1288 CrossRef CAS.
- A. I. Vinokur and D. C. Fredrickson, Inorg. Chem., 2016, 55, 6148 CrossRef CAS PubMed.
- A. Chaturvedi, S. Stefanoski, M. H. Phan, G. S. Nolas and H. Srikanth, Appl. Phys. Lett., 2011, 99, 162513 CrossRef.
- L. W. Li, M. Kadonaga, D. X. Huo, Z. H. Qian, T. Namiki and K. Nishimura, Appl. Phys. Lett., 2012, 101, 122401 CrossRef.
- Y. Zhang, Y. Yang, X. Xu, L. Hou, Z. Ren, X. Li and G. Wilde, J. Phys. D: Appl. Phys., 2016, 49, 145002 CrossRef.
- Y. Zhang, B. Yang and G. Wilde, J. Alloys Compd., 2015, 619, 12 CrossRef CAS.
- K. P. Shinde, S. H. Jang, J. W. Kim, D. S. Kim, M. Ranot and K. C. Chung, Dalton Trans., 2015, 20386 RSC.
- T. Paramanik, T. Samanta, R. Ranganathan and I. Das, RSC Adv., 2015, 5, 47860 RSC.
- B. Sattibabu, A. K. Bhatnagar, K. Vinod, S. Rayaprol, A. Mani, V. Siruguri and D. Das, RSC Adv., 2016, 6, 48636 RSC.
- L. W. Li, K. Nishimura and H. Yamane, Appl. Phys. Lett., 2009, 94, 102509 CrossRef.
- B. Li, W. J. Hu, X. G. Liu and F. Yang, Appl. Phys. Lett., 2008, 92, 242508 CrossRef.
- S. Yin, V. Sharma, A. McDannald, F. A. Reboredo and M. Jain, RSC Adv., 2016, 6, 9475 RSC.
- Y. Zhang, L. Hou, Z. Ren, X. Li and G. Wilde, J. Alloys Compd., 2016, 565, 635 CrossRef.
- B. Uthaman, K. S. Anand, R. K. Rajan, H. H. Kyaw, S. Thomas, S. Al-Harthi, K. G. Suresh and M. R. Varma, RSC Adv., 2015, 5, 86144 RSC.
- Y. K. Zhang, X. Xu, Y. Yang, L. Hou, Z. Ren, X. Li and G. Wilde, J. Alloys Compd., 2016, 667, 130 CrossRef CAS.
- H. Amanai, H. Onodera, M. Ohashi, S. Matsuo, H. Yamauchi, Y. Yamaguchi and N. Sato, J. Magn. Magn. Mater., 1995, 148, 413 CrossRef CAS.
- H. Onodera, N. Uchida, M. Ohashi, H. Yamauchi, Y. Yamaguchi and N. Sato, J. Magn. Magn. Mater., 1994, 137, 35 CrossRef CAS.
- P. A. Kotsanidis, J. K. Yakinthos and W. Schafer, J. Alloys Compd., 1996, 242, 90 CrossRef CAS.
- W. Schafer, G. Will, P. A. Kotsanidis and J. K. Yakinthos, J. Magn. Magn. Mater., 1990, 88, 13 CrossRef.
- W. Schafer, W. Kockelmann, G. Will, J. K. Yakinthos and P. A. Kotsanidis, J. Alloys Compd., 1997, 250, 565 CrossRef.
- M. Reehuis, J. Rodriguez-Carvajal, M. E. Danebrock and W. Jeitschko, J. Magn. Magn. Mater., 1995, 151, 273 CrossRef CAS.
- W. Schafer, W. Kockelman, G. Will, P. A. Kotsanidis, J. K. Yakinthos and J. Linhart, J. Magn. Magn. Mater., 1994, 132, 243 CrossRef.
- B. K. Banerjee, Phys. Lett., 1964, 12, 16 CrossRef.
- V. Franco, A. Conde, V. Provenzano and R. D. Shull, J. Magn. Magn. Mater., 2010, 322, 218 CrossRef CAS.
- H. Zhang, B. G. Shen, Z. Y. Xu and J. Shen, Appl. Phys. Lett., 2013, 102, 092401 CrossRef.
|
This journal is © The Royal Society of Chemistry 2016 |