DOI:
10.1039/C5RA24070K
(Paper)
RSC Adv., 2016,
6, 1958-1965
Electro-Fenton treatment of imidazolium-based ionic liquids: kinetics and degradation pathways
Received
14th November 2015
, Accepted 16th December 2015
First published on 18th December 2015
Abstract
In this study, the removal of five imidazolium-based ionic liquids (ILs) from water was accomplished by a heterogeneous electro-Fenton treatment. Different ILs were selected in order to study the effect of the alkyl chain length and the nature of the anion. Initially, the effect of the catalyst (iron alginate beads) dosage and current were evaluated. The results showed that the optimum conditions were attained when operating with 4.27 g of catalyst and 0.3 A, achieving degradation yields and TOC reductions higher than 95% after 90 min and 80% after 480 min, respectively. Regarding the ILs with common cations, lower degradation rates were obtained for those with longer alkyl chains, thus 1-ethyl-3-methylimidazolium dicyanamide, exhibits faster degradation than 1-hexyl-3-methylimidazolium dicyanamide and 1-butyl-3-methylimidazolium dicyanamide. In addition, the influence of the counter anions on the oxidation rate of different ILs under an electro-Fenton treatment was demonstrated, following the order: methylsulfate > dicyanamide > acetate. The Microtox ecotoxicity tests of the initial and treated samples revealed that none of the three studied ILs with shorter alkyl chain lengths showed toxicity and only 1-hexyl-3-methylimidazolium dicyanamide and 1-butyl-3-methylimidazolium dicyanamide presented toxic levels, with EC50 values of 269.85 and 47.55 mg L−1, respectively. Nonetheless, after 480 min of heterogeneous electro-Fenton treatment at 0.3 A, the toxicity of both was completely reduced. Finally, to confirm the mineralization of these compounds, the identification of several reaction intermediates in the heterogeneous electro-Fenton treatment of three ILs was assayed and a plausible degradation pathway was proposed.
1. Introduction
Over the past decade, the use of ionic liquids (ILs) has been extended to a broad number of industrial applications such as catalysts,1 biocatalysts,2 biotechnology, synthetic chemistry and electrochemistry.3
Typical ILs are salts composed of an organic or inorganic cation with delocalized charges and an inorganic or organic anion, generally a liquid below 100 °C. Because of their negligible vapour pressure, low-flammability and high chemical and thermal stability, they have been regarded as “green” solvents.4 However, due to their high stability certain amounts of ILs would be found in a few years in the conventional wastewater treatment plants.
Even though the abiotic and biotic effect of ILs on the environment is still unknown, their environmental fate is being carefully regarded. Recently, biological evaluations have pointed out the negative effect that these “green” solvents can provoke on microorganisms, even more harmful than the conventional solvents.5–7
Furthermore, it has been demonstrated the strong influence of the alkyl chain length of the cation on the removal of ILs.8–10 They found a significant primary biodegradation for (eco)toxicologically unfavourable compounds carrying long alkyl side chains (C6 and C8). In contrast for (eco)toxicologically more recommendable imidazolium ionic liquids with short alkyl (≤C6) and short functionalised side chains, no biological degradation could be found.9
At the present time, scarce studies have been conducted to evaluate the degradation of ILs; however these pollutants are being introduced into the environment. Thus, the development of new technologies able to treat and ensure the complete removal of these compounds from aquatic environments is required.
Recently, the so-called electrochemical advanced oxidation processes (EAOPs) have been on the spotlight of the scientific community. They are based on the electrochemical generation of oxidants used for the degradation of the pollutants. Thus, EAOPs have several advantages such as their high efficiency, versatility and safety since they operate at mind conditions. Among them, electro-Fenton is almost the most typical.11
The electro-Fenton treatment is based on the generation of powerful oxidant radicals such as ˙OH, able to degrade a huge number of hazardous compounds. In this process, many reactions take place and had been described in detail by Zhao et al.12 Among them, the main reaction is the generation of hydroxyl radicals13 (eqn (1)) from Fenton's reaction between Fe2+ present in the medium and the H2O2 electrochemically generated by the continuous air flow on a suitable cathode.
|
Fe2+ + H2O2 → Fe3+ + ˙OH + OH−
| (1) |
Some of the main advantages of this technique are the onsite production of H2O2 and the continuous regeneration of Fe3+ to Fe2+ at the cathode. However, it is difficult the operation in continuous mode when the classic homogeneous electro-Fenton process is used.
Over the past years, a wide range of inexpensive heterogeneous iron catalysts, in which iron can be effectively binding into the structure of several materials, have been developed demonstrating their potential to address this problem. Recently, Bocos et al.14 synthesized polyacrylamide iron enriched hydrogels able to be used as heterogeneous catalysers in the electro-Fenton treatment of dye polluted streams. Furthermore, it has been extensively reported the high catalytic activity of iron alginate beads (FeAB) on the elimination of several organic compounds with minimal iron leaching.15,16 FeAB have demonstrated their high efficiency and stability working in batch and continuous mode during the decontamination of wastewater by an electro-Fenton process.16,17 Furthermore, their high versatility has made of them an interesting alternative when treating different pollutants such as dyes or pesticides.18,19
Up to now, only few studies have reported the application of advanced oxidation processes (AOPs) to the elimination of ILs from water. Stepnowski and Zaleska,20 studied the degradation of ILs comparing different photodegradation methods at room temperature. Fabianska et al.21 studied the elimination of five ILs using BDD anodes by anodic oxidation, demonstrating the great influence of the IL composition on the degradation rate of the pollutant as well as the influence of chlorine on this treatment, which enhances the elimination of the parent compound. Another interesting study was performed by Siedlecka et al.,22 who elucidate the degradation pathway of the IL of 1-butyl-3-methylimidazolium chloride by anodic oxidation treatment using 4 anode materials: IrPt, IrO2, PbO2 and BDD. As expected, these authors determined that BDD anode is the most suitable for the elimination of this IL from water. More recently, Munoz et al.23 reported the elimination of different families of ILs by the application of Fenton treatment at different temperatures ranging from 70° to 90 °C. However, to our best knowledge there are not studies dealing with the elimination of ILs by an electro-Fenton treatment.
On this work, the breakdown of five imidazolium-based ILs by electro-Fenton process using FeAB as heterogeneous catalyst was evaluated and different key parameters were optimized. Thus, the effect of FeAB dosage and current, on the degradation kinetic of the ILs and their mineralization, were determined. Besides, it was evaluated the contribution of the alkyl chain length as well as the nature of the anion on their elimination. To determine the toxicity of the solutions, ecotoxicity assays with the bacterium Vibrio fischeri were performed. Finally, mineralization pathways for the degradation of different ILs studied were proposed based on the identification of the degradation products and different cations released along the electro-Fenton treatment and determined by GC/MS and ion-exclusion HPLC.
2. Materials and methods
2.1. Chemicals
Five ILs from the imidazolium family were purchased to Iolitec (GmbH, Germany). Their complete and abbreviated names, as well as their chemical structures are depicted in Table 1. The initial concentration of the five ILs, used in all assays, was 0.5 g L−1. Na2SO4 used as electrolyte was provided by Sigma-Aldrich (Barcelona, Spain). All aqueous solutions were prepared with Millipore filtered water and volumetric lab equipment.
Table 1 Chemical name, abbreviated name and molecular structure of the five ILs studied
IL name |
Abbreviated name |
Chemical structure |
Molecular structure |
1-Hexyl-3-methylimidazolium dicyanamide |
[C6mim][N(CN)2] |
C12H19N5 |
 |
1-Butyl-3-methylimidazolium dicyanamide |
[C4mim][N(CN)2] |
C10H15N5 |
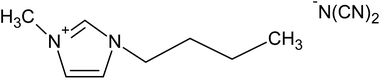 |
1-Ethyl-3-methylimidazolium dicyanamide |
[C2mim][N(CN)2] |
C8H11N5 |
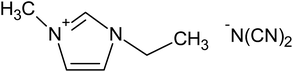 |
1-Ethyl-3-methylimidazolium acetate |
[C2mim][CH3CO2] |
C8H14N2O2 |
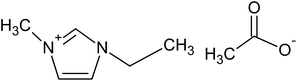 |
1-Ethyl-3-methylimidazolium methylsulfate |
[C2mim][CH3SO4] |
C2H14N2O4S |
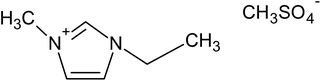 |
2.2. Electrochemical experiments
All experiments were carried out in a 0.25 L cylindrical glass reactor with 0.20 L of IL solution and Na2SO4 (0.05 M) at pH 3. Besides, different FeAB dosage (3.20 g, 4.27 g and 5.33 g) were added to the bulk as catalyst in order to test the effect of iron concentration in the treatment. The catalyst was prepared following the procedure optimized by Iglesias et al.24 The solution was continuously agitated with a magnetic stirrer to avoid concentration gradients. H2O2 was in situ electrochemically generated through the continuous bubbling of compressed air (0.75 L min−1) on the cathode surface. The electric parameters were monitored with a multimeter (Fluke 175). A constant current was applied (0.1, 0.2 or 0.3 A) by two electrodes connected to a direct power supply (HP model 3662). Carbon felt (Carbon Lorraine, France) and boron-doped diamond (BDD) supplied by DIACHEM®, Germany (4–5 μm diamond film thickness and doping level around 2500 ppm) were selected as cathode and anode, respectively. Carbon Felt was placed on the inner wall of the cell (6 × 12 cm), covering the total internal perimeter while BDD (3 × 6 × 0.2 cm) was centred in the reactor. All the experiments were performed in duplicate at ambient temperature. The reported results were the mean values with a standard deviation lower than 5%.
Samples were drawn periodically from the reactor and centrifuged at 10
000 rpm for 5 min. The supernatant was separated to analyse pH, and IL concentration.
2.3. Kinetic studies
Kinetic studies were conducted to determine the model of behaviour of the degradation each IL. ILs concentrations along the time were fitted to a suitable kinetic equation and the rate constants were calculated using the software Sigma plot. Based on the Marquard–Levenberg algorithm, this software uses an iterative procedure that seeks the values of the parameters to minimize the sum of the square differences between the predicted and the observed values.
2.4. Analytical method
GC/MS analysis. Extractions of 200 mL of the aqueous sample were performed three times with 30 mL of ethyl acetate each time. After extraction, samples were dried with a rotary evaporator and taken up to 200 μL of ethyl acetate for their analysis by GC-MS. Thus, the identification of the degradation products formed during the electro-Fenton treatment of the ILs was performed using a 6850 Agilent GC equipped with a 5955C VLMSD equipped with a HP-5-MS column. Hydrogen was the carrier gas at a flow rate of 1.2 mL min−1. For the GC separation, the GC injection port temperature was set at 280 °C. The program temperature started at 50 °C (held during 5 min). Subsequently, the temperature ramp was set at 5°C min−1 to 280 °C. The temperature was maintained at 280 °C for 5 min The MS detector was operated in EI mode (70 eV).
Total organic carbon analysis. Total organic carbon (TOC) was determined using a multi N/C 3100 equipment (Analytic Jena) coupled with a NDIR detector (C.A.C.T.I, University of Vigo). From these results, the TOC percentages abatements were calculated from the following equation (eqn (2)): |
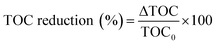 | (2) |
where ΔTOC is the variation of TOC (mg L−1) during the treatment and TOC0 (mg L−1) is the TOC value at the beginning of the treatment.
2.5. Toxicity tests
The potential toxicity of the five ILs and their reaction intermediates was evaluated following the bioluminescence of the marine bacterium Vibrio fischeri (Lumistox LCK 487), by means of the Microtox® method according to the international standard process 81.9% Basic Test. The value of the inhibition of the bacterium luminescence (%) was measured after 15 min of exposition of Vibrio fischeri to the studied solutions at 20 °C. The bioluminescence measurements were carried out in samples extracted at initial time and after 480 min of treatment by electro-Fenton at constant current (0.3 A) and in control samples without ILs.
3. Results and discussion
There are numerous families of ILs, such as pyridinium, guanidinium, phosphonium or imidazolium. Those with aromatic cations, as for example imidazolium have showed to be more toxic and to have antimicrobial properties. Furthermore, the toxicity of these latter is variable, being especially increased for cations with long alkyl chain and anions with high lipophilicity or difficult to be hydrolysed.9 Given the elevated use, in different industrial fields, of imidazolium-based ILs, this study deals the elimination from water of five ILs from this family. Furthermore the influence of the cation cores and the anion nature will be studied in depth.
3.1. Effect of FeAB dosage on the degradation kinetic of the ILs
It is well known that iron concentration is one of the key parameters controlling the electro-Fenton process, since it rules the generation of hydroxyl radicals from Fenton's reaction. On this way, high or low dosages can inhibit the efficiency of the treatment25 by the increase or reduction on the rate of the reaction described on eqn (1).
Initially, the electro-Fenton process was carried out at constant current of 0.1 A and different FeAB dosages (3.20, 4.27 or 5.3 g) were added to the medium in order to follow the degradation of the different ILs. Table 2 shows the obtained results, which indicated that the degradation of the different ILs could be quantitatively described by a first-order kinetic equation with respect to the IL concentration. In addition, Fig. 1 represent the profiles of the IL [C6mim][N(CN)2] degradation by electro-Fenton treatment at different FeAB dosage. Notably, the removal of the IL from the solution was faster with FeAB dosages of 4.27 g. Besides, as it can be concluded from Table 2, same trend was observed for the different ILs studied. The use of higher FeAB dosage did not provide positive effects; on the contrary, a delay in ILs oxidation rates was observed when the FeAB dosage was increased in the medium. This fact could be explained as a result of secondary reactions between hydroxyl radical and the excess of iron present.26 Moreover, at low dosage the reaction rate was reduced. Thus a dosage of 4.27 g of FeAB in 0.2 L of reaction medium seemed to be the optimal catalyst concentration. These results are in accordance with the obtained by Iglesias et al.24 They already pointed out, that working with 4.27 g of FeAB dosage improves the efficiency of the electro-Fenton treatment of the pesticide imidacloprid. Thus, after obtain similar results during degradation experiments; this concentration was used henceforth in the following experiments.
Table 2 Influence of FeAB dosage on the kinetic rate constant of the five ILs studieda
IL |
FeAB (g) |
k (min−1) |
R2 |
k is the kinetic coefficient for the first-order reaction. |
[C6mim][N(CN)2] |
3.20 |
0.0094 |
0.9973 |
4.27 |
0.0154 |
0.9968 |
5.33 |
0.0032 |
0.9957 |
[C4mim][N(CN)2] |
3.20 |
0.0129 |
0.9924 |
4.27 |
0.0195 |
0.9990 |
5.33 |
0.0102 |
0.9966 |
[C2mim][N(CN)2] |
3.20 |
0.0207 |
0.9996 |
4.27 |
0.0356 |
0.9974 |
5.33 |
0.0157 |
0.9937 |
[C2mim][CH3CO2] |
3.20 |
0.0198 |
0.9968 |
4.27 |
0.0317 |
0.9971 |
5.33 |
0.0158 |
0.9959 |
[C2mim][CH3SO4] |
3.20 |
0.0254 |
0.9975 |
4.27 |
0.0370 |
0.9983 |
5.33 |
0.0164 |
0.9991 |
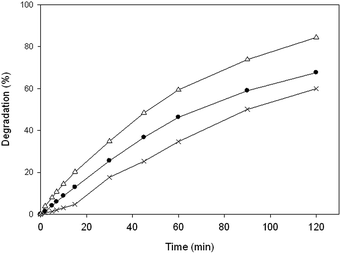 |
| Fig. 1 Effect of the FeAB dosage on the degradation profile of the IL [C6mim][N(CN)2] by electro-Fenton process at constant current of 0.1 A. Symbols: circles: 3.20 g of FeAB; triangles: 4.27 g of FeAB; crosses: 5.33 g of FeAB. | |
3.2. Effect of the alkyl chain length and the counter anion on the degradation kinetic
To determine the effect of the alkyl chain length and the counter anion on the degradation of ILs, three different alkyl chain length such as [C6mim][N(CN)2], [C4mim][N(CN)2] and [C2mim][N(CN)2] and three ILs with different anion like [C2mim][N(CN)2], [C2mim][CH3CO2] and [C2mim][CH3SO4] were used as model pollutants in this study. The values of first-order kinetic parameter and the statistical correlation parameters of the different studied ILs after 120 min of electro-Fenton treatment are listed in Table 2. As expected, the lowest apparent rate constants were obtained when the compounds of longest alkyl chain were treated. Increasing the alkyl chain length, the imidazolium ring is less targeted to be attacked by the oxidant radicals. These results are in accordance with those previously reported for the degradation of ILs by different systems such as UV, UV/H2O2 or Fenton systems.20,27 Zhou et al.27 for example, concluded that the degradation rate of ILs was much faster for [C2mim]Br and [C4mim]Br than for [C6mim]Br and [C8mim]Br and [C10mim]Br in a nZVI/H2O2 system.
Recently, Domínguez et al.28 demonstrated the influence of the counter anions on the oxidation rate of different ILs under a Fenton's treatment, following the order: methanesulfonate > methylsulfate > chloride > acetate. In our assays the degradation rate followed the order: methylsulfate > dicyanamide > acetate. Similarly to the results found by Domínguez et al.,28 it was detected the slower degradation of [C2mim][CH3CO2] which could be associated with the precipitation of iron species detected in these experiments, with the resulting inhibition of its catalytic action.
3.3. Influence of the current on the degradation and mineralization yields of the ILs
It has been extensively reported that the current plays an important role on the pollutant degradation achieved under an electrochemical treatment. Usually, as the current is incremented, the degradation rates can be accelerated25 due to the higher production of homogeneous ˙OH from the reaction of the hydrogen peroxide formed at the bulk (eqn (1) and (3)). In addition, the generation of heterogeneous radicals on the electrode surface following eqn (4) permits the degradation of organic matter (eqn (5)) and this fact can considerably enhance the results attained during the treatment. |
O2 + 2H+ + 2e− → H2O2
| (3) |
|
BDD + H2O → BDD (˙HO) + H+ + e−
| (4) |
|
BDD(˙OH) + R →BDD + CO2 + H2O
| (5) |
where BDD is anode material; BDD(˙OH) is heterogeneous ˙OH radicals formed at the anode surface and R is organic matter.
Many authors have reported that higher current values can provoke a decrease of the mineralization current efficiency since more energy is spent in oxygen evolution and as consequence there are less ˙OH species able to oxidize the organic pollutants.29,30 In 2008, Özcan et al.31 reported that the disappearance of the pesticide picloram become longer after raise the current at upper values than 0.3 A. As these organics decrease at the bulk, there is less matter to degrade, thus promoting parasitic reactions that can affect the efficiency of the process.
In this work, current intensities ranging from 0.1 to 0.3 A were studied, following their influence on the degradation and mineralization of the target compounds. In relation to the effect of current on degradation of IL, in Fig. 2 is showed the obtained results for all ILs studied. It is highlighted the high degradation levels, around 90%, were attained after 120 min of treatment at 0.1 and 0.2 A of current; while the complete degradation of the ILs was yielded after the same treatment time using 0.3 A of current. However, more time is required to obtain near complete mineralization. The mineralization level of all ILs was measurement as TOC reduction percentage. The results depicted in Fig. 2 reveal the progressive mineralization decay, however the increase on the alkyl chain length has an important contribution on the TOC reduction. In this way, the rate of elimination of [C6mim][N(CN)2] and [C4mim][N(CN)2] was much lower than the found for the ILs with [C2mim] cation. Likely, the attack of the oxidants would begin by the cleavage of the alkyl side chain from the N atom of the ring, which is known to be a less stable bond than others present on the ring.27,32
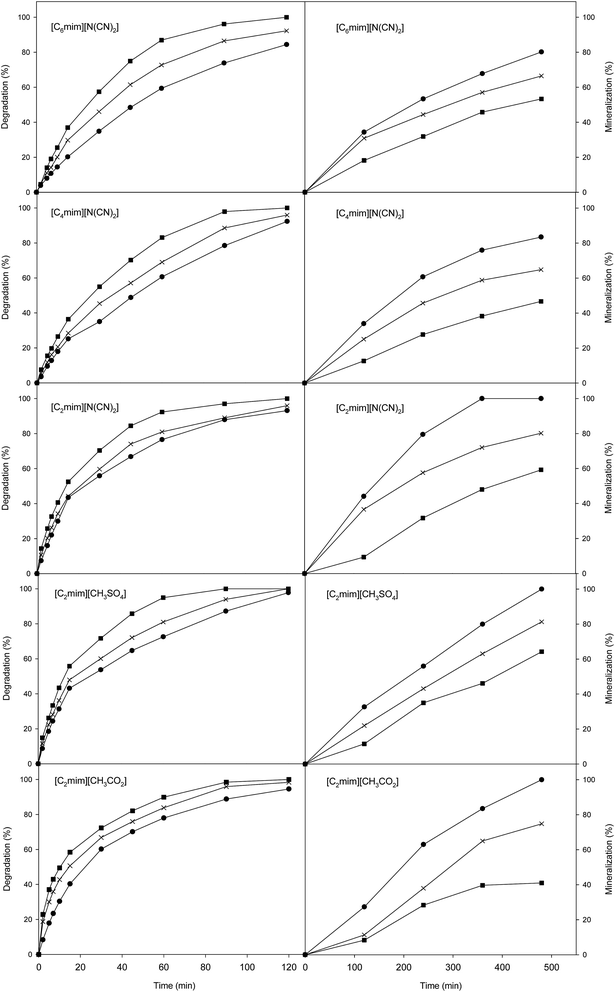 |
| Fig. 2 Effect of the current on degradation (left) and mineralization (right) of [C6mim][N(CN)2], [C4mim][N(CN)2], [C2mim][N(CN)2], [C2mim][CH3SO4] and [C2mim][CH3CO2] by electro-Fenton process with 4.27 g of FeAB. Symbols on degradation: circles 0.1 A; crosses 0.2 A; squares 0.3 A. Symbols on mineralization: squares 0.1 A; crosses 0.2 A; circles 0.3 A. | |
As can be seen in Fig. 2, the mineralization rates achieved by the ILs with [C2mim] cation followed the same order than those previously found during the degradation assays. Thus, highest removal rates were obtained for the [C2mim][CH3SO4] while [C2mim][N(CN)2] and [C2mim][CH3CO2] showed lowest mineralization rates. Moreover, in this assay the system attained the maximum oxidation rate at 0.3 A.
3.4. Microtox toxicity tests
The toxicity tests were performed following the inhibition on the luminescence of the bacterium Vibrio fischeri due to its exposure to the ILs. Initially, for all ILs concentration–response curves were obtained and EC50 values were calculated following the method described in Section 2. The obtained EC50 values of ILs samples revealed that none of the ILs with the cation C2mim showed elevated contribution in the toxicity levels. It was only detected toxic effects for [C4mim][N(CN)2] and [C6mim][N(CN)2] with EC50 values of 269.85 and 47.55 mg L−1, respectively. These results are in agreement with those previously reported by Cvetko Bubalo et al.6 and Domínguez et al.,28 who pointed out that the lipophilic character of the IL is increased with the length of the alkyl chain thus increasing the disruption into the bacterium luminescence. On this way, the nature of the anion did not show a significant contribution on the toxicity assays. However, since the initial values were below the EC50 this could not be consider as a concluding result.
It has been shown that only [C4mim][N(CN)2] and [C6mim][N(CN)2] have toxicities in the bioassay used. For this reason, after 480 min of heterogeneous electro-Fenton treatment of solution of [C6mim][N(CN)2] and [C4mim][N(CN)2] at 0.3 A, samples were drawn and the inhibition of the Vibrio fischeri luminescence was determined. The assays showed negligible inhibition values that revealed that the toxicity of these solutions was completely reduced by the oxidation of these compounds.
3.5. Degradation pathway
The analysis of the degradation intermediates of the three ILs with the same cation ([C6mim][N(CN)2], [C4mim][N(CN)2] and [C2mim][N(CN)2]) were performed by GC/MS at 0, 30 and 60 min of treatment. Similar intermediate products were detected in the samples obtained in the degradation of studied ILs and at different times, therefore the degradation pattern was established. In all cases, as previously reported by some authors23,27,32 the first step was de hydroxylation of the ILs, resulting in the formation of higher molecular structure than the initial sample. In addition, after oxidation of the imidazolium ring, it was opened and further degraded in new by-products. Subsequently, the hydroxyl radicals further attack to these formed by-products, ending on the formation of carboxylic acids at the end of the treatment. In addition, the analysis of the carboxylic acids reveals the presence in the samples of oxamic, oxalic, glycolic, acetic and formic acid. It is known that these compounds are normally found after electro-Fenton processes, finally ending on the formation of CO2 and H2O. As example in the Fig. 3 a schema with the molecular structures of the intermediates generated in the degradation of [C6mim][N(CN)2] is showed. The highest peaks of the compounds a–g appeared at 30 min and then they gradually were diminished. Therefore, the different intermediates determined in the samples confirm that the application of a heterogeneous electro-Fenton system on the treatment of different ILs it allows their complete mineralization.
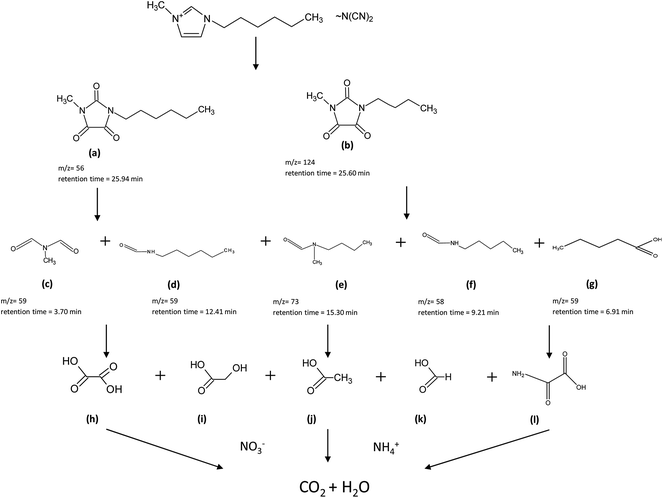 |
| Fig. 3 Degradation pathway of the IL [C6mim][N(CN)2]: (a) 1-ethyl-3-hexyl-2,4,5 trioxoimidazolidine, (b) 1-ethyl-3-butyl-2,4,5-trioxoimidazolidine, (c) N-formyl-N-methyl, (d) formamide, N-hexyl, (e) formamide, N-butyl-N-methyl, (f) formamide, N-pentyl, (g) pentanoic acid (h) oxalic acid, (i) glycolic acid, (j) acetic acid, (k) formic acid, (l) oxamic acid. | |
4. Conclusions
In this work, the efficiency of a heterogeneous electro-Fenton system on the treatment of different ILs was probed. It was demonstrated the effect of FeAB dosage and current in the degradation rate. Moreover, the effect of the current on the degradation yields and the degradation kinetic was studied. Regarding to the ILs, it was demonstrated that lowest degradation rates were obtained for those with longest alkyl chain and the influence of the counter anions on the oxidation rate of different ILs under electro-Fenton treatment, following the order: methylsulfate > dicyanamide > acetate. The ecotoxicity test of the initial and treated samples showed initial toxicity upper than the EC50 only for longest alkyl chain length. However, after the heterogeneous electro-Fenton treatment the toxicity was completely reduced.
Finally, the identification of carboxylic acids by ion-exclusion HPLC allowed the verification of the feasibility of the heterogeneous electro-Fenton system on the treatment of the studied ILs.
Acknowledgements
This research has been financially supported by the Spanish Ministry of Economy and Competitiveness, Xunta de Galicia and ERDF Funds (Projects CTM2014-52471-R and GRC 2013/003). The authors are grateful to the Spanish Ministry of Economy and Competitiveness for the financial support of Elvira Bocos under the FPI program and Marta Pazos under the Ramón y Cajal program.
References
- H. Olivier-Bourbigou, L. Magna and D. Morvan, Appl. Catal., A, 2010, 373, 1–56 CrossRef CAS.
- A. P. M. Tavares, B. Pinho, O. Rodriguez and E. A. Macedo, Procedia Eng., 2012, 42, 226–230 CrossRef.
- T. Chen, H. Xiong, W. Wen, X. Zhang and S. Wang, Bioelectrochemistry, 2013, 91, 8–14 CrossRef CAS PubMed.
- P. Tundo, A. Perosa and F. Zecchini, Methods Reagents Green Chem., 2007, 1–314 Search PubMed.
- J. Ranke, K. Mölter, F. Stock, U. Bottin-Weber, J. Poczobutt, J. Hoffmann, B. Ondruschka, J. Filser and B. Jastorff, Ecotoxicol. Environ. Saf., 2004, 58, 396–404 CrossRef CAS PubMed.
- M. Cvjetko Bubalo, K. Radoševic, I. Radojcic Redovnikovic, J. Halambek and V. Gaurina Srcek, Ecotoxicol. Environ. Saf., 2014, 99, 1–12 CrossRef CAS PubMed.
- K. S. Egorova and V. P. Ananikov, ChemSusChem, 2014, 7, 336–360 CrossRef CAS PubMed.
- K. M. Docherty, J. K. Dixon and C. F. Kulpa Jr, Biodegradation, 2007, 18, 481–493 CrossRef CAS PubMed.
- S. Stolte, S. Abdulkarim, J. Arning, A. Blomeyer-Nienstedt, U. Bottin-Weber, M. Matzke, J. Ranke, B. Jastorff and J. Thöming, Green Chem., 2008, 10, 214–224 RSC.
- M. Markiewicz, C. Jungnickel, A. Markowska, U. Szczepaniak, M. Paszkiewicz and J. Hupka, Molecules, 2009, 14, 4396–4405 CrossRef CAS PubMed.
- C. A. Martínez-Huitle and E. Brillas, Appl. Catal., B, 2009, 87, 105–145 CrossRef.
- C. Zhao, L. E. Arroyo-Mora, A. P. DeCaprio, V. K. Sharma, D. D. Dionysiou and K. E. O'Shea, Water Res., 2014, 67, 144–153 CrossRef CAS PubMed.
- M. A. Oturan and J. Aaron, Crit. Rev. Environ. Sci. Technol., 2014, 44, 2577–2641 CrossRef CAS.
- E. Bocos, M. Pazos and M. A. Sanromán, J. Chem. Technol. Biotechnol., 2014, 89, 1235–1242 CrossRef CAS.
- O. Iglesias, M. A. F. de Dios, T. Tavares, M. A. Sanromán and M. Pazos, J. Ind. Eng. Chem., 2015, 27, 276–282 CrossRef CAS.
- E. Rosales, O. Iglesias, M. Pazos and M. A. Sanromán, J. Hazard. Mater., 2012, 213–214, 369–377 CrossRef CAS PubMed.
- O. Iglesias, E. Rosales, M. Pazos and M. A. Sanromán, Environ. Sci. Pollut. Res., 2013, 20, 2252–2261 CrossRef CAS PubMed.
- E. Alfaya, O. Iglesias, M. Pazos and M. A. Sanromán, RSC Adv., 2015, 5, 14416–14424 RSC.
- Y. Dong, W. Dong, Y. Cao, Z. Han and Z. Ding, Catal. Today, 2011, 175, 346–355 CrossRef CAS.
- P. Stepnowski and A. Zaleska, J. Photochem. Photobiol., A, 2005, 170, 45–50 CrossRef CAS.
- A. Fabianska, T. Ossowski, P. Stepnowski, S. Stolte, J. Thöming and E. M. Siedlecka, Chem. Eng. J., 2012, 198–199, 338–345, DOI:10.1016/j.cej.2012.05.108.
- E. M. Siedlecka, A. Fabiañska, S. Stolte, A. Nienstedt, T. Ossowski, P. Stepnowski and J. Thöming, Int. J. Electrochem. Sci., 2013, 8, 5560–5574 CAS.
- M. Munoz, C. M. Domínguez, Z. M. de Pedro, A. Quintanilla, J. A. Casas and J. J. Rodriguez, Catal. Today, 2015, 240, 16–21 CrossRef CAS.
- O. Iglesias, J. Gómez, M. Pazos and M. A. Sanromán, Appl. Catal., B, 2014, 144, 416–424 CrossRef CAS.
- E. Brillas and C. A. Martínez-Huitle, Appl. Catal., B, 2015, 166–167, 603–643 CrossRef CAS.
- M. Panizza and M. A. Oturan, Electrochim. Acta, 2011, 56, 7084–7087, DOI:10.1016/j.electacta.2011.05.105.
- H. Zhou, Y. Shen, P. Lv, J. Wang and P. Li, J. Hazard. Mater., 2015, 284, 241–252 CrossRef CAS PubMed.
- C. M. Domínguez, M. Munoz, A. Quintanilla, Z. M. de Pedro, S. P. M. Ventura, J. A. P. Coutinho, J. A. Casas and J. J. Rodriguez, J. Chem. Technol. Biotechnol., 2014, 89, 1197–1202 CrossRef.
- E. M. Siedlecka, W. Mrozik, Z. Kaczynski and P. Stepnowski, J. Hazard. Mater., 2008, 154, 893–900 CrossRef CAS PubMed.
- A. Dirany, I. Sirés, N. Oturan, A. Özcan and M. A. Oturan, Environ. Sci. Technol., 2012, 46, 4074–4082 CrossRef CAS PubMed.
- A. Özcan, Y. Sahin, A. S. Koparal and M. A. Oturan, J. Hazard. Mater., 2008, 153, 718–727 CrossRef PubMed.
- J. Gao, L. Chen, Y. Y. He, Z. C. Yan and X. J. Zheng, J. Hazard. Mater., 2014, 265, 261–270 CrossRef CAS PubMed.
|
This journal is © The Royal Society of Chemistry 2016 |