Experimental and quantum chemical study on the transformation behavior of bisphenol S by radical-driven persulfate oxidation†
Received
1st August 2021
, Accepted 11th November 2021
First published on 23rd November 2021
Abstract
An in-depth study of the degradation of bisphenol S (BPS) by both single-walled carbon nanotubes and heat activated persulfate (PS) was conducted in detail. The effects of various factors, namely, material dosage, initial substrate concentration, initial pH and the water matrix, on the removal of BPS were evaluated, and 10 μM BPS was completely removed in 90 min under the optimal conditions of [BPS]0
:
[PS]0 = 1
:
100, T = 25 °C, pH0 = 7.0, and [N-SWCNTs] = 20 mg L−1. Fast removal of BPS was also obtained when the reaction temperature reached 65 °C without catalyst. There were 15 intermediates identified in total, and hydroxylation, sulfate addition, carboxylation, S–C bond cleavage and polymerization were considered to be the main transformation pathways of BPS in both the systems based on LC-MS analysis. The discrepancy in the proportion of hydroxyl and sulfate radicals involved in the two systems led to different distribution and abundance of the observed products. According to quantum chemical calculations, hydroxylation, hydrogen atom abstraction and sulfate addition occurred as the initial reactions between radicals and BPS. Furthermore, the intrinsic reaction coordinate (IRC) paths of the generated primary products were obtained using the program Gaussian 09. Low reaction barriers (22.20, 25.06 and 13.85 kJ mol−1, respectively) revealed that the H atom linked to the phenoxy group and the ortho-C of BPS were the most likely sites to react. The present work reveals the overall transformation behavior of BPS in a radical-triggered PS system by combining experimental and theoretical study.
Water impact
Bisphenol S (BPS), an alternative to bisphenol A, has been removed by various methods because it is more persistent and increasingly detected. In this study, the discrepancies between the transformation behavior of BPS in two radical-driven persulfate systems were systematically studied by combining experiments and quantum chemistry, and the main degradation pathways were proposed. The results further enrich our knowledge on the transformation behavior, trends and fate of BPS.
|
1. Introduction
Bisphenol S (BPS), a substitution product of bisphenol A (BPA), is very widely utilized as a starting material of some esters (e.g., Makrolon), additives and fire retardants, etc., due to its favorable properties. Previous studies have shown that BPS has been widely detected in food,1–3 paper products,4 personal care products,5 surface water,6–8 indoor dust9,10 and even human urine.9,11,12 Its frequency of detection and concentrations are comparable with those of BPA. For example, Cao et al. measured BPS and BPA in food composite samples at concentration levels of 1.2–35.0 ng g−1 and 5.3–41.0 ng g−1, and their detection frequencies were 5.9% and 6.2%, respectively.1 Furthermore, the BPS content has increased over the years.7,8 It is proven that this compound possesses estrogenic activity,13–15 acute toxicity,16,17 neurotoxicity,18,19 immunotoxicity,17,20–22 and reproductive and developmental toxicity.23–25 In addition, BPS is strongly associated with obesity and steatosis.26–28 It is much worse that, compared to BPA, BPS has a longer half-life period and poorer bioavailability,29 which makes its removal more difficult and more expensive.30
With the ever-rising concern regarding this problem, various methods, such as chlorination,31 biodegradation,32 photochemical degradation,33 and manganese dioxide and ferrate oxidation,34,35 have been applied to eliminate BPS. Nevertheless, high time consumption, high cost, complex pretreatment, etc., hinder the practical application of the aforementioned methods. Furthermore, in these systems, BPS may be transformed into multifarious intermediates with unpredictable toxicity, although complete removal can also be achieved. In this regard, a green, effective and environmentally friendly technology is urgently needed.
As a prospective technique, advanced oxidation processes (AOPs) can generate multitudes of reactive free radicals to destroy pollutants with a high mineralization degree.36 The past few decades have witnessed progress in the comprehensive study of persulfate (PS), and it is well-documented that sulfate radicals (SR, SO4˙−, E0 = 2.6–3.1 V) and hydroxyl radicals (˙OH, E0 = 1.9–2.8 V) can be formed after PS activation.37–40 Common activation approaches include heat,41 ultraviolet irradiation,41,42 ultrasound,43 base,44 transition metals (e.g., nanosized zero-valent iron (nZVI), Fe3O4 Co2+),41,45–48 natural organic matter (NOM),49 ozone,42 quinones,50 and electrochemical reactions.51 For instance, Liu et al. and Lu et al. used Fe@C-activated peroxymonosulfate (PMS) and ultrasound-triggered PS, respectively, to remove aqueous BPS, and the complete removal of BPS at the micromole level was obtained in minutes, indicating the excellent performance of SR-AOPs.43,52 Nevertheless, only 5 or 7 byproducts were reported in these works. The specific transformation intermediates and overall pathways of BPS under SR-AOP conditions are still less studied. Additionally, phenolic organic compounds tend to polymerize when treated by general chemical methods.53–56 As a kind of bisphenol, it is unknown whether BPS can couple into its corresponding dimers, trimers and other complexes.
Currently, ongoing interest has been focused on nanocarbon-based catalysts for heterogeneous PS activation.57 Extensive research has elucidated the excellent PS catalysis ability of carbonaceous materials, such as graphene (GP),58 reduced graphene oxide (rGO)59 and carbon nanotubes (CNTs),57,60,61 to eliminate persistent organic pollutants efficiently. Furthermore, doping with heteroatoms (e.g., N) can significantly improve their catalytic ability. Unfortunately, little knowledge of the removal of BPS using nanocarbon-based material activated PS has been obtained so far.
In this context, we investigated the overall BPS transformation behavior (including the kinetics, intermediates and reaction pathways) using (N-doped) carbonaceous materials and heat-activated PS. The purposes of the present research are to 1) test the effects of catalyst dosage, solution pH, initial substrate concentration and water matrix on BPS abatement in the catalyzed PS system; 2) identify the probable reaction products and deduce the possible oxidation pathways of BPS by combining the results of LC-TOF-MS analysis and transition state calculations; and 3) explore the underlying effect of reaction temperature on the distribution and abundance of the identified intermediates of BPS. This research can provide a useful technique to treat the water and wastewater containing BPS and its analogues, and enrich our knowledge of the transformation behavior, trends and fate of BPS.
2. Materials and methods
2.1 Reagents and water matrices
Details of the reagents and water matrices used in this study are given in Text S1.†
2.2 Preparation and characterization of nitrogen-doped-nanocarbon-based materials
The nitrogen-doped-nanocarbon-based materials were prepared using a thermal decomposition method, and the specific preparation is shown in Text S2,† along with their respective characterization results and discussion.
2.3 Experimental procedure
A series of kinetics experiments of BPS were carried out in a 250 mL conical flask containing a certain amount of BPS and the catalyst at 25 °C in a SHA-B thermostatic water bath shaker (Boyuan, China). After being stirred for 30 min, the above reaction system reached adsorption–desorption equilibrium (see Fig. S5†). By adding 0.8 mL of 0.1 M PS, the catalytic reaction was initiated. At selected time points, 1 mL of the reaction solution was sampled and quickly filtered through a 0.22 μm Teflon filter into a HPLC vial pre-loaded with 50 μL of 0.2 M Na2S2O3 as terminating agent.
No catalyst was added for the PS-only oxidation at ambient temperature as a control. Consequently, the sampled reaction solution could be directly analyzed using the HPLC instrument. Other experimental procedures were as same as those used with the catalyst. In order to determine the difference in the transformation of BPS during PS oxidation with and without catalyst, we also carried out the tests of heat-activated PS oxidation at 65 °C. We conducted all experiments in triplicate and maintained the initial pH at the desired value by adding 0.1 M NaOH and H2SO4.
2.4 Analytical methods
The residual concentrations of BPS were quantified using a Hitachi high-performance liquid chromatography (HPLC) system (Hitachi, Japan) equipped with a Zorbax 300SB-C18 column (150 × 4.6 mm, 5 μm) and a 5420 UV-vis detector. The HPLC parameters were set as: 3‰ formic acid aqueous solution and methanol (v/v = 45/55) as the mobile phase at a flow rate of 1 mL min−1, an injection volume of 80 μL, and a detection wavelength of 298 nm. The limit of detection, defined as three times the ratio of signal to instrument noise (3 S/N), was 0.01 μM in the HPLC system.
Before LC-MS analysis, sample solutions were purified using solid phase extraction (SPE) equipment to remove salts and particles. The specific processes can be found elsewhere.62 The possible reaction intermediates of BPS were detected using an X500R QTOF mass spectrometer system (AB Sciex Pte. Ltd., USA) equipped with a Thermo BDS Hypersil C18 column (2.1 × 100 mm, 2.4 mm, Thermo Fisher Scientific, USA). The detailed HPLC-MS/MS setups are listed in the ESI,† Text S3. All data were analyzed using the software SCIEX OS 1.6 (AB Sciex).
The EPR spectra were recorded using an E500-9.5/12 electron paramagnetic resonance spectrometer (Bruker, Germany), using DMPO as a spin-trapping reagent for SO4˙− and ˙OH detection.
The residual concentration of PS anions was measured using a Persee UV-vis spectrophotometer (Beijing, China) at 352 nm.
2.5 Transition state calculations
Transition state calculations for the reactions of SO4˙− and ˙OH with BPS were implemented using the software Gaussian 09 with the m062x/lanl2dz basis set. The presence of only one negative eigenvalue of the Hessian matrix and intrinsic reaction coordinate (IRC) analysis were used to further verify the structures of the transition states (TSs). The energies (E) of the reactants, TSs and products were acquired from their relative output files by searching for the keyword “HF”, and the reaction barrier was then calculated to infer the possible reaction pathways. The atom coordinates in the theoretical calculations are presented in the ESI† (Tables S6–S8).
3. Results and discussion
3.1 Removal of BPS by activated PS
3.1.1 Reaction kinetics of BPS in the PS/(N-)SWCNTs system.
The five nanocarbon-based materials, especially SWCNTs, showed good adsorption and activation of PS to remove aqueous BPS, and their performance was promoted after N doping (Text S4†). Thus, SWCNTs and N-SWCNTs were chosen for subsequent research.
Fig. 1(a–c) reveals the effects of the material concentration, initial substrate concentration and solution pH on the removal of BPS in the PS/N-SWCNTs system, respectively. As shown in Fig. 1(a), the elimination efficiency of the substrate increased with increasing N-SWCNTs concentration, likely due to the increasing BPS adsorption capacity and greater amounts of hydroxyl radicals in the reaction solution (see Text S5†). Moreover, the BPS elimination rate was positively related to the N-SWCNTs dosage. To be specific, the pseudo-first order reaction rate constants (kobs) were determined to be 0.0216, 0.0726 and 0.1574 min−1 when the N-SWCNTs dosage was 10, 20 and 30 mg L−1, respectively. This result was also observed in the reports of Liu et al. and Cheng et al.52,63
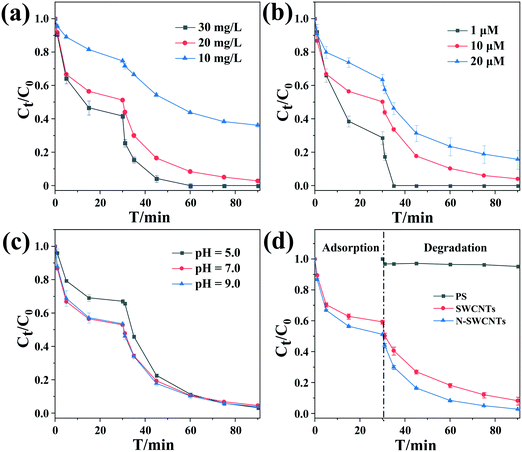 |
| Fig. 1 Effects of material concentration (a), initial substrate concentration (b), solution pH (c), and N doping (d) on the degradation of BPS in the PS/N-SWCNTs system. Conditions: [BPS]0 = 10 μM, [PS]0 = 1.0 mM, T = 25 °C, pH0 = 7.0, [(N-)SWCNTs] = 20 mg L−1. | |
We also studied the effects of the initial substrate concentrations (1, 10 and 20 μM) on BPS removal. From Fig. 1(b), it can be seen that as the initial BPS concentration increased, the removal efficiency decreased from 100.0% to 84.3%. Accordingly, the kobs values dropped from 0.5310 to 0.0487 min−1. For a given PS concentration, we assumed that a constant amount of active species was generated in solution, which led to a high removal efficiency at a low initial BPS concentration. Additionally, a higher concentration of the target compound would result in the formation of more reaction intermediates, and their competition with BPS for oxidants and active sites on the N-SWCNTs inevitably inhibited the removal of the parent compound.
In Fig. 1(c), there is no obvious difference in the removal trend of BPS at initial pH values of 5.0, 7.0 and 9.0, and the corresponding kobs values are 0.0753, 0.0726 and 0.0711 min−1. Additionally, the decreased adsorption of the target compound at pH 5.0 might be attributed to the existence of surface oxygenic functional groups (e.g., –COOH and –OH) on the SWCNTs, as indicated by the absorption peaks in the FT-IR spectra (Fig. S4†). The restrained deprotonation of the acidic functional groups (–COOH and –OH) of SWCNTs at this pH further inhibited the π-electron-donor ability of the graphene surface, which therefore resulted in weakened π–π electron-donor–acceptor interactions between the aromatic compound and the SWCNTs.64 In fact, the solution pH is a vital factor during the persulfate oxidation process, and variation in the pH can greatly change the removal efficiency of the substrates via impacting the species distribution and contribution of the generated reactive oxygen species (ROS), as well as changing the states in which the organic compounds are present.65 On account of the proliferating ROS content derived from the catalytic decomposition of persulfate by nanocarbon-based materials,57 the impact of pH at pH 5.0–7.0 could be ignored. Since buffer was not added, the change in the pH during the reaction for different initial pH values was investigated (Fig. S6†). As shown, two pH drops occurred in the reaction process. One resulted from the introduction of a large amount of CO2 due to the violent oscillation during the adsorption stage, and the other was attributed to the addition of PS. In the degradation stage, the pH value was maintained at values near pH 4.2, 5.7 and 6.2, respectively. That is, the effect of pH on the PS activation process was actually studied in the pH range of 4.2–6.2. This result suggested that the PS/nanocarbon-based catalysts technique can be applied over a wide pH range.
Fig. 1(d) depicts the removal of BPS by PS activated with SWCNTs and N-SWCNTs under the optimal conditions: 1.0 mM PS, 20 mg L−1 catalyst, initial pH 7.0 and 25 °C. As shown, 96.0% removal of 10 μM BPS could be obtained in 90 min of reaction in the case of N-SWCNTs. As discussed above, the promotion of both adsorption and activation after N doping was observed. This activation effect was highly consistent with the residual PS concentration results (Fig. S7†).
3.1.2 Degradation of BPS in different water matrices.
Three different water samples, namely, tap water, river water, and secondary effluent, were used to explore the application potential of the N-SWCNTs/PS technique for the elimination of BPS. The reaction temperature was maintained at 25 °C and no pH adjustment was conducted. As shown in Fig. S8,† (nearly) complete removal of BPS in ultrapure water and tap water was obtained, while the removal declined to 80.3% and 52.3% for river water and secondary effluent, respectively, due to the high TOC level shown in Table S4.†
3.2 Intermediate identification and reaction pathway elucidation
The experiments for LC-MS analysis were carried out under the optimal kinetics conditions for the PS–catalyst system. Given the possible effect of the catalyst on BPS transformation, we also conducted the degradation experiment in a heat-activated PS system for comparison. As shown in Fig. S10,† heat-activated PS could also degrade BPS effectively, and thus, we took 65 °C as an example. Based on our detailed analysis, overall, we identified a total of 15 intermediates in two regimes (12 for the PS–catalyst system and 14 for the PS–heat system). Moreover, all the calculated errors between the measured mass values and the proposed theoretical molecular formulas were not more than 5 ppm (Table S5†), demonstrating the high reliability of the structural assignments. The specific structures of these intermediates and the inferred transformation pathways in the PS/N-SWCNTs and PS–heat systems are summarized in Fig. 2, 3 and S14.†
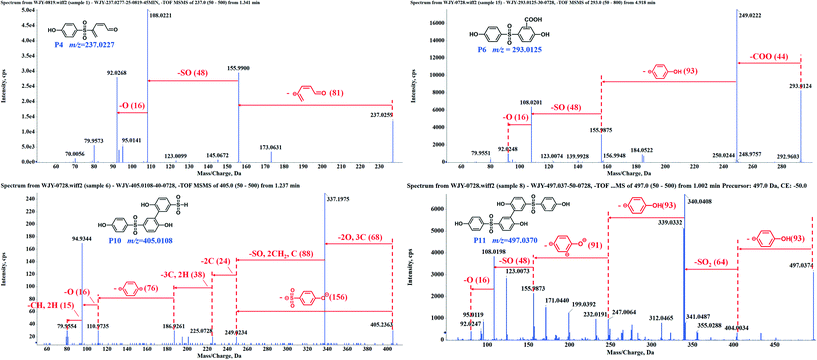 |
| Fig. 2 MS/MS spectra of the main products (P4, P6, P10 and P11) in the PS/N-SWCNTs system. | |
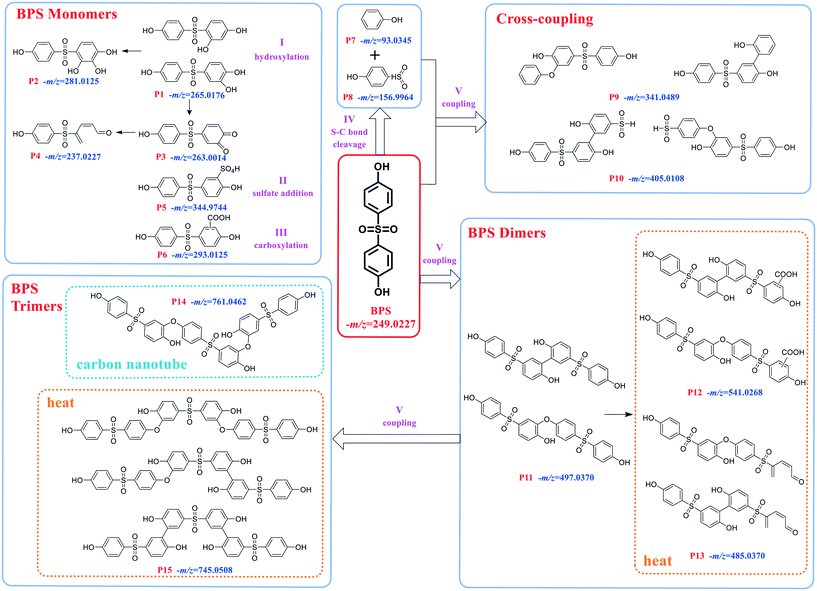 |
| Fig. 3 Transformation pathways of BPS in the PS/N-SWCNTs system and PS–heat system. Conditions: [BPS]0 = 10 μM, [PS]0 = 1.0 mM, pH0 = 7.0. Note that the intermediates not specified in this figure were common products in the two systems. | |
3.2.1 The PS/N-SWCNTs system.
The m/z values ranged from 93.03 to 761.04 for the 12 by-products (labeled as P1–P11 and P14) detected in the PS/N-SWCNTs system. The m/z values of P1–P6 were very close to that of BPS; moreover, the m/z values of P1–P6, P9–P11 and P14 were 1.4–3.1 times as high as 249.02, implying that they might be the analogues and coupling products of BPS. The highest peak area of all 12 by-products obtained from the LC-MS spectra are recorded in Fig. S11.† Obviously, the peak areas of P4, P6, P10 and P11 were much greater than those of the others, indicating they were the major intermediates of BPS. Therefore, their MS/MS spectra are illustrated in detail in Fig. 2 as examples. As shown, three daughter ions with m/z values of 155.99, 108.02 and 92.03 in the compound P4 were attributed to the stepwise losses of C5H5O (81 Da), SO (48 Da) and O (16 Da), respectively. Hence, P4 was recognized as a decarbonylation derivative of P3 (a secondary by-product of BPS), and its structure is displayed in Fig. 2. For compound P6, a BPS fragment ion with m/z 249.02 was observed, which resulted from the loss of a carboxyl group (–COO) from the parent ion of m/z 293.01, indicating that P6 was a carboxylation product of BPS. The BPS fragment ion was also found in the MS/MS spectrum of compound P10 (m/z 405.01), which corresponded to the loss of a sulfonylphenol fragment ion (156 Da). Therefore, P10 was identified as a cross-coupling product of the BPS substrate and sulfonylphenol P8. Similarly, P11 could be inferred to be the dimer of BPS. The rest of the MS/MS spectra can be found in Fig. S14.† Herein, there were four pairs of isomers, including hydroxylation product P1 and coupling products P9, P10 and P11.
Based on the identified intermediates, we proposed five transformation pathways of BPS in PS/N-SWCNTs solution, shown in Fig. 3. Pathway I was regarded as the hydroxylation of BPS, leading to the formation of P1 and P2. Additionally, P4 was generated by the decarbonylation of P3, an intermediate that came from the direct oxidation of P1. Potakis et al. also found that PS treatment could cause the benzene ring of BPA to open.66 Pathway II and pathway III were sulfate addition and carboxylation, respectively, and correspondingly produced the sulfate addition product P5 and the carboxylation product P6. Recent studies on SR-AOPs have reported sulfate addition and carboxylation products, such as in the oxidation process of dimethyl phthalate,67 terbutaline,68 nonylphenol triclosan,65 BPA,69,70 diclofenac,71 and natural organic matter.72 Given that these two kinds of addition products have been identified as intermediates for various substrates, sulfate addition and carboxylation should be the significant pathways during SR-AOPs and are worth considering in depth.
As illustrated in Fig. 3, the cleavage of the S–C bond was degradation pathway IV of BPS to further generate the phenol P7 and sulfonyl phenol P8. These two mono-benzene ring products resulting from BPS scission have been detected in various systems, such as ultrasound/PS,43 heat/PS,73 CuCo2S4/PMS,74 and boron/PS systems,75 indicating that the cleavage of the S–C bond is a common pathway during BPS oxidation.
After undergoing H abstraction of hydroxyl radicals or electron transfer of sulfate radicals, BPS and its phenolic intermediates could self- and cross-couple into its oligomers (P9–P11, and P14) as pathway V. Based on the highest abundance of P10 and P11 as shown in Fig. S11,† the polymerization reaction was mainly responsible for BPS transformation in this system. In general, the radical polymerization of organic compounds can occur via two routes, namely, C–C and C–O coupling, thus resulting in the two different structures of P9–P11 in this work. Surprisingly, we failed to detect the trimer of BPS in this system, but its hydroxylated product P14 with an m/z value of 761.05 appeared. The opposite data were obtained in the PS–heat system (discussed below). Therefore, we deduced that P14 should be formed from the cross-coupling reaction of P1 and P11, while the self-coupling of BPS radicals yielded the related trimer P15. Many studies have revealed that the coupling behaviors derive from the reaction of the corresponding radicals.74–77 Our previous work even found a multitude of coupling products during the photodegradation of polyfluorinated dibenzo-p-dioxins (PFDDs) on silica gel.78 In the studies mentioned above, the coupling reaction always plays a vital role in the transformation of all the selected compounds. Therefore, it warrants further in-depth study.
3.2.2 The PS–heat system.
The main reaction intermediates and transformation pathways of BPS in the PS–heat reaction solution were the same as those in the PS/N-SWCNTs system. Specifically, eleven intermediates (P1–P11) occurred in these two systems. Although the reaction mechanisms in the two solutions were the same (radical control), some unique products were identified. For example, the hydroxylated trimers of BPS (P14) disappeared, while the coupling products P12, P13 and P15 were detected under the PS–heat conditions. Furthermore, the abundance of these reaction products was largely different. The changes in the relative contents of the common products (P1–P11) with reaction time are shown in Fig. S13.† As can be seen, all 11 intermediates were detected at 5 min in the two studied systems, and additionally, a typical evolution trend was observed in which they accumulated to the maximum concentration in the first stage of reaction and then gradually degraded into other products in the later stage. Clearly, the contents of these products were generally higher in the PS–heat solution than in the PS–catalyst system. Among them, the distribution of the coupling products (P11) exhibited the most significant difference. Zrinyi et al. in 2017 systematically investigated the effect of reaction temperature on the transformation pathway of benzoic acid oxidized by PS, and the results showed that the product distribution did indeed differ with changing the temperature.79 They also found that SO4˙− mainly contributed to the transformation of the substrate at 70 °C (very similar to the temperature of 65 °C used in this work), whereas ˙OH was considered to be the dominant active species using the PS activated by N-doped single-wall carbon nanotubes. The dominant role of SO4˙− might produce more radicals of BPS and its phenolic intermediates via electron transfer and further lead to greater generation of polymers in the PS–heat system. Another possibility was attributed to the loss of some BPS by nanocarbon materials adsorption when sampled.
In summary, the observations showed that BPS would be subjected to hydroxylation, sulfate addition, carboxylation, S–C bond cleavage and polymerization during PS oxidation. However, the distribution and abundance of the products differed depending on the dominant ROS.
3.3 Theoretical calculations
In general, three reaction mechanisms, namely, radical adduct formation (RAF), hydrogen atom abstraction (HAA), and single electron transfer (SET) are deduced as three possible initial oxidation reactions of SO4˙− and ˙OH with phenolic organic contaminants (POCs), as suggested by previous investigations.80,81 The experimental and theoretical results demonstrate that SO4˙− can oxidize POCs with electron-rich groups via the SET mechanism.82–84Fig. 4(a) depicts the specific SET mechanism of SO4˙− with BPS. As shown, BPS was first transformed into a transient cationic intermediate via electron transfer by SO4˙−, and then the BPS radical (C12H9O4S˙) was yielded rapidly after the loss of a proton. The combination of C12H9O4S˙ with its resonance forms caused by the delocalization of the unpaired electron resulted in the formation of polymeric intermediates.76,85 Additionally, the TSs between SO4˙− and BPS were calculated to examine the possible sites at which RAF could occur (Fig. 4(b)). Due to the interaction between SO4˙− and the other hydroxyl group of BPS, we cannot accurately calculate the reaction barriers of 3C and 5C. Therefore, the reaction barriers at C (2) and C (6) are shown in Fig. 4(b). As can be seen, their ΔE values were quite low (13.85 kJ mol−1 for C (2) and 14.00 kJ mol−1 for C (6)), further confirming the specific structure of sulfate addition product P5.
 |
| Fig. 4 Scheme of the SET mechanism (a) and energy profiles (ΔE, kJ mol−1) calculated at the m062x/lanl2dz level for the RAF mechanism (b) of SO4˙− with BPS. | |
For ˙OH, RAF and HAA are generally suggested as the initial oxidation reactions. Therefore, considering the symmetry of the molecular structure of BPS, steric hindrance and electron donating group, four possible carbon sites (2C, 3C, 5C and 6C) and five possible hydrogen sites (1H, 2H, 3H, 4H and 5H) for ˙OH attack were calculated. The corresponding energy profiles are shown in Fig. 5. It can be seen that the ΔE values at the C (2), C (3), C (5) and C (6) sites were 22.20, 23.75, 24.07 and 26.95 kJ mol−1, respectively, which are comparable to those shown in Fig. 4(b). The reaction barriers for HAA at the five hydrogen sites were much higher than those for RAF, except for ΔE at H (1); and their specific values were 25.06 (1), 70.93 (2), 64.20 (3 and 4) and 54.88 (5) kJ mol−1. Consequently, RAF and HAA could easily occur at the C (2), C (3), C (5), C (6) and H (1) positions. However, only two peaks of the hydroxylation product P1 were detected from the total ion current (TIC) chromatograph, suggesting that the hydroxyl group preferred to link to the C (2) and C (3) sites of BPS, as depicted in Fig. 3. The C12H9O4S˙ radical generated after ˙OH abstracted H could participate in the subsequent coupling reaction. The detection of hydroxyl addition and coupling products P1, P2 and P9–P15 further verified this result.
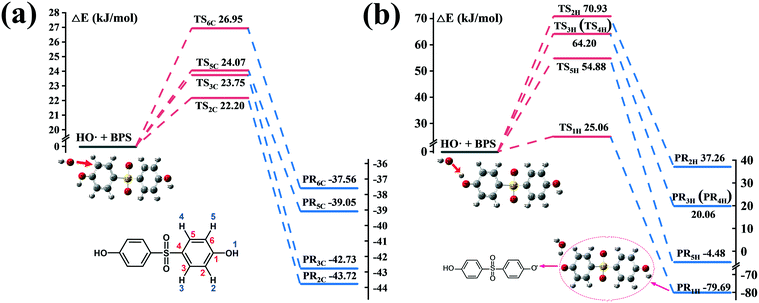 |
| Fig. 5 Energy profiles (ΔE, kJ mol−1) calculated at the m062x/lanl2dz level for the RAF (a) and HAA (b) mechanisms of ˙OH with BPS. | |
3.4 Application potential
Due to its good stability, high solubility, and low price, the application of PS in environmental pollution control is promising. Based on the kinetics results in Text S2 and Fig. S1–S3,† effective removal of BPS can be achieved under the experimental conditions of BPS
:
PS = 1
:
100, pH0 = 7.0, T = 25 °C and catalyst = 20 mg L−1 for the PS–catalyst system. The concentration of PS used in our reaction solutions was 1.0 mM PS, which is much lower than that used for BPA removal in previous works (5 mM PS and 0.5 g L−1 catalyst,86 and 4.385 mM PS and 0.122 g L−1 catalyst,87 respectively). Based on the market prices (∼9.85 × 10−3 dollar per g for PS and 58.94 dollar per g for SWCNTs), the costs of using 1.0 mM PS and 20 mg L−1 N-SWCNTs to treat 1 liter of water were calculated to be ∼2.66 × 10−3 dollar and 1.18 dollar, respectively. Assuming that the loss of catalyst is ∼10% for each cycle, the cost of the PS/N-SWCNTs system would be <0.45 dollar per L. Therefore, this work represents a relatively economical method for wastewater treatment.
4. Conclusion
N-SWCNTs and heat showed good PS activation potential for the removal of aqueous BPS. Under the conditions [BPS]0
:
[PS]0 = 1
:
100, T = 25 °C, pH0 = 7.0, and [N-SWCNTs] = 20 mg L−1, 10 μM BPS could be completely removed in 90 min. The removal efficiency was improved with increased material dosage at a low initial concentration of BPS. TOC content was highly related with BPS elimination in real water matrices. According to LC-MS analysis, 12 products were generated in the PS/N-SWCNTs system, and hydroxylation, sulfate addition, carboxylation, S–C bond cleavage and polymerization were responsible for their generation. Some unique products were found under the PS–heat conditions. The distribution and abundance of the BPS intermediates differed depending on the dominant ROS involved. The reaction barriers for hydroxylation and the hydrogen atom and sulfate addition reaction calculated based on transition state theory further verified the above proposed mechanisms.
Author contributions
Junyan Wei: investigation, data curation, writing – original draft, software. Linning Yin: data curation. Ruijuan Qu: validation. Xiaoxue Pan: methodology, writing – review & editing. Zunyao Wang: writing – review & editing, supervision.
Conflicts of interest
There are no conflicts of interest to declare.
Acknowledgements
This research was financially supported by the Major Science and Technology Program for Water Pollution Control and Treatment of China (No. 2018ZX07208001-06), the Fundamental Research Funds for the Central Universities (No. 021114380173) and the National Natural Science Foundation of China (No. 22076076, 21876082).
References
- X. L. Cao, I. Kosarac, S. Popovic, S. Zhou, D. Smith and R. Dabeka, LC-MS/MS analysis of bisphenol S and five other bisphenols in total diet food samples, Food Addit. Contam., Part A, 2019, 36(11), 1740–1747 CrossRef CAS PubMed
.
- J. Zhou, X. H. Chen, S. D. Pan, J. L. Wang, Y. B. Zheng, J. J. Xu, Y. G. Zhan, Z. X. Cai and M. C. Jin, Contamination status of bisphenol A and its analogues (bisphenol S, F and B) in foodstuffs and the implications for dietary exposure on adult residents in Zhejiang Province, Food Chem., 2019, 294, 160–170 CrossRef CAS PubMed
.
- C. Liao and K. Kannan, A survey of bisphenol A and other bisphenol analogues in foodstuffs from nine cities in China, Food Addit. Contam., Part A, 2014, 31, 319–329 CrossRef CAS PubMed
.
- C. Liao, F. Liu and K. Kannan, Bisphenol S, a new bisphenol analogue, in paper products and currency bills and its association with bisphenol A residues, Environ. Sci. Technol., 2012, 46, 6515–6522 CrossRef CAS PubMed
.
- C. Liao and K. Kannan, A Survey of Alkylphenols, Bisphenols, and Triclosan in Personal Care Products from China and the United States, Arch. Environ. Contam. Toxicol., 2014, 67, 50–59 CrossRef CAS PubMed
.
- E. Yamazaki, N. Yamashita, S. Taniyasu, J. Lam, P. K. S. Lam, H.-B. Moon, Y. Jeong, P. Kannan, H. Achyuthan, N. Munuswamy and K. Kannan, Bisphenol A and other bisphenol analogues including BPS and BPF in surface water samples from Japan, China, Korea and India, Ecotoxicol. Environ. Saf., 2015, 122, 565–572 CrossRef CAS PubMed
.
- H. Jin and L. Zhu, Occurrence and partitioning of bisphenol analogues in water and sediment from Liaohe River Basin and Taihu Lake, China, Water Res., 2016, 103, 343–351 CrossRef CAS PubMed
.
- Y. Liu, S. Zhang, N. Song, R. Guo, M. Chen, D. Mai, Z. Yan, Z. Han and J. Chen, Occurrence, distribution and sources of bisphenol analogues in a shallow Chinese freshwater lake (Taihu Lake): Implications for ecological and human health risk, Sci. Total Environ., 2017, 599–600, 1090–1098 CrossRef CAS PubMed
.
- C. Liao, F. Liu, H. Alomirah, V. D. Loi, M. A. Mohd, H.-B. Moon, H. Nakata and K. Kannan, Bisphenol S in Urine from the United States and Seven Asian Countries: Occurrence and Human Exposures, Environ. Sci. Technol., 2012, 46, 6860–6866 CrossRef CAS PubMed
.
- W. Wang, K. O. Abualnaja, A. G. Asimakopoulos, A. Covaci, B. Gevao, B. Johnson-Restrepo, T. A. Kumosani, G. Malarvannan, T. B. Minh, H.-B. Moon, H. Nakata, R. K. Sinha and K. Kannan, A comparative assessment of human exposure to tetrabromobisphenol A and eight bisphenols including bisphenol A via indoor dust ingestion in twelve countries, Environ. Int., 2015, 83, 183–191 CrossRef CAS
.
- X. Ye, L.-Y. Wong, J. Kramer, X. Zhou, T. Jia and A. M. Calafat, Urinary Concentrations of Bisphenol A and Three Other Bisphenols in Convenience Samples of U.S. Adults during 2000–2014, Environ. Sci. Technol., 2015, 49, 11834–11839 CrossRef CAS
.
- H.-J. Lehmler, B. Liu, M. Gadogbe and W. Bao, Exposure to Bisphenol A, Bisphenol F, and Bisphenol S in U.S. Adults and Children: The National Health and Nutrition Examination Survey 2013–2014, ACS Omega, 2018, 3, 6523–6532 CrossRef CAS
.
- M. Y. Chen, M. Ike and M. Fujita, Acute toxicity, mutagenicity, and estrogenicity of bisphenol-A and other bisphenols, Environ. Toxicol., 2002, 17, 80–86 CrossRef CAS PubMed
.
- K. Okuda, T. Fukuuchi, M. Takiguchi and S. I. Yoshihara, Novel pathway of metabolic activation of bisphenol A-related compounds for estrogenic activity, Drug Metab. Dispos., 2011, 39, 1696–1703 CrossRef CAS PubMed
.
- I. Sidorkiewicz, K. ZaręBa, S. A. Wołczyński and J. Czerniecki, Endocrine-disrupting chemicals-Mechanisms of
action on male reproductive system, Toxicol. Ind. Health, 2017, 33(7), 601–609 CrossRef CAS
.
- J. Moreman, O. Lee, M. Trznadel, A. David, T. Kudoh and C. R. Tyler, Acute Toxicity, Teratogenic, and Estrogenic Effects of Bisphenol A and Its Alternative Replacements Bisphenol S, Bisphenol F, and Bisphenol AF in Zebrafish Embryo-Larvae, Environ. Sci. Technol., 2017, 51, 12796–12805 CrossRef CAS PubMed
.
- W. Qiu, H. Shao, P. Lei, C. Zheng, C. Qiu, M. Yang and Y. Zheng, Immunotoxicity of bisphenol S and F are similar to that of bisphenol A during zebrafish early development, Chemosphere, 2018, 194, 1–8 CrossRef CAS PubMed
.
- B. Kim, E. Colon, S. Chawla, L. N. Vandenberg and A. Suvorov, Endocrine disruptors alter social behaviors and indirectly influence social hierarchies via changes in body weight, Environ. Health, 2015, 14, 64 CrossRef PubMed
.
- C. D. Kinch, K. Ibhazehiebo, J. H. Jeong, H. R. Habibi and D. M. Kurrasch, Low-dose exposure to bisphenol A and replacement bisphenol S induces precocious hypothalamic neurogenesis in embryonic zebrafish, Proc. Natl. Acad. Sci. U. S. A., 2015, 112, 1475–1480 CrossRef CAS PubMed
.
- M. Ma, D. Crump, R. Farmahin and S. W. Kennedy, Comparing the effects of tetrabromobisphenol-A, bisphenol A, and their potential replacement alternatives, TBBPA-bis(2,3-dibromopropyl ether) and bisphenol S, on cell viability and messenger ribonucleic acid expression in chicken embryonic hepatocytes, Environ. Toxicol. Chem., 2015, 34, 391–401 CrossRef CAS PubMed
.
- C. Zhao, Z. Tang, J. Yan, J. Fang, H. Wang and Z. Cai, Bisphenol S exposure modulate macrophage phenotype as defined by cytokines profiling, global metabolomics and lipidomics analysis, Sci. Total Environ., 2017, 592, 357–365 CrossRef CAS
.
- X. Dong, Z. Zhang, S. Meng, C. Pan, M. Yang, X. Wu, L. Yang and H. Xu, Parental exposure to bisphenol A and its analogs influences zebrafish offspring immunity, Sci. Total Environ., 2018, 610–611, 291–297 CrossRef CAS PubMed
.
- M. Naderi, M. Y. L. Wong and F. Gholami, Developmental exposure of zebrafish (Danio rerio) to bisphenol-S impairs subsequent reproduction potential and hormonal balance in adults, Aquat. Toxicol., 2014, 148, 195–203 CrossRef CAS
.
- M. D. Mersha, B. M. Patel, D. Patel, B. N. Richardson and H. S. Dhillon, Effects of BPA and BPS exposure limited to early embryogenesis persist to impair non-associative learning in adults, Behav. Brain Funct., 2015, 11, 27 CrossRef
.
- H. Ullah, A. Ambreen, N. Ahsan and S. Jahan, Bisphenol S induces oxidative stress and DNA damage in rat spermatozoa in vitro and disrupts daily sperm production in vivo, Toxicol. Environ. Chem., 2017, 1–23 CAS
.
- L. Peyre, P. Rouimi, G. de Sousa, C. Héliès-Toussaint, B. Carré, S. Barcellini, M.-C. Chagnon and R. Rahmani, Comparative study of bisphenol A and its analogue bisphenol S on human hepatic cells: A focus on their potential involvement in nonalcoholic fatty liver disease, Food Chem. Toxicol., 2014, 70, 9–18 CrossRef CAS PubMed
.
- B. Liu, H.-J. Lehmler, Y. Sun, G. Xu, Y. Liu, G. Zong, Q. Sun, F. B. Hu, R. B. Wallace and W. Bao, Bisphenol A substitutes and obesity in US adults: analysis of a population-based, cross-sectional study, Lancet Planet. Health, 2017, 1, e114–e122 CrossRef PubMed
.
- R. Rezg, A. Abot, B. Mornagui, S. Aydi and C. Knauf, Effects of Bisphenol S on hypothalamic neuropeptides regulating feeding behavior and apelin/APJ system in mice, Ecotoxicol. Environ. Saf., 2018, 161, 459–466 CrossRef CAS PubMed
.
- V. Gayrard, M. Z. Lacroix, F. C. Grandin, S. H. Collet and N. Picard-Hagen, Oral Systemic Bioavailability of Bisphenol A and Bisphenol S in Pigs, Environ. Health Perspect., 2019, 127, 077005 CrossRef CAS
.
- Y. J. Choi and L. S. Lee, Aerobic soil biodegradation of bisphenol (BPA)
alternatives bisphenol S and bisphenol AF compared to BPA, Environ. Sci. Technol., 2017, 51, 13698–13704 CrossRef CAS
.
- Y. Gao, J. Jiang, Y. Zhou, S.-Y. Pang, J. Ma, C. Jiang, Y. Yang, Z.-s. Huang, J. Gu and Q. Guo, Chlorination of bisphenol S: Kinetics, products, and effect of humic acid, Water Res., 2018, 131, 208–217 CrossRef CAS PubMed
.
- X. Wang, J. Chen, R. Ji, Y. Liu, Y. Su and R. Guo, Degradation of Bisphenol S by a Bacterial Consortium Enriched from River Sediments, Bull. Environ. Contam. Toxicol., 2019, 103, 630–635 CrossRef CAS PubMed
.
- A. Kovačič, C. Gys, T. Kosjek, A. Covaci and E. Heath, Photochemical degradation of BPF, BPS and BPZ in aqueous solution: Identification of transformation products and degradation kinetics, Sci. Total Environ., 2019, 664, 595–604 CrossRef PubMed
.
- J. Li, S. Y. Pang, Y. Zhou, S. Sun, L. Wang, Z. Wang, Y. Gao, Y. Yang and J. Jiang, Transformation of bisphenol AF and bisphenol S by manganese dioxide and effect of iodide, Water Res., 2018, 143, 47–55 CrossRef CAS PubMed
.
- T. Yang, L. Wang, Y. Liu, Z. Huang, H. He, X. Wang, J. Jiang, D. Gao and J. Ma, Comparative study on ferrate oxidation of BPS and BPAF: Kinetics, reaction mechanism, and the improvement on their biodegradability, Water Res., 2019, 148, 115–125 CrossRef CAS
.
- X. Duan, H. Sun, Z. Shao and S. Wang, Nonradical reactions in environmental remediation processes: uncertainty and challenges, Appl. Catal., B, 2018, 224, 973–982 CrossRef CAS
.
- W. D. Oh, Z. Dong and T. T. Lim, Generation of sulfate radical through heterogeneous catalysis for organic contaminants removal: Current development, challenges and prospects, Appl. Catal., B, 2016, 194, 169–201 CrossRef CAS
.
- S. Wacławek, H. V. Lutze, K. Grübel, V. V. T. Padil, M. Černík and D. D. Dionysiou, Chemistry of persulfates in water and wastewater treatment: A review, Chem. Eng. J., 2017, 330, 44–62 CrossRef
.
- I. A. Ike, K. G. Linden, J. D. Orbell and M. Duke, Critical review of the science and sustainability of persulphate advanced oxidation processes, Chem. Eng. J., 2018, 338, 651–669 CrossRef CAS
.
- J. Lee, U. von Gunten and J.-H. Kim, Persulfate-Based Advanced Oxidation: Critical Assessment of Opportunities and Roadblocks, Environ. Sci. Technol., 2020, 54, 3064–3081 CrossRef CAS PubMed
.
- X. Pan, L. Yan, R. Qu and Z. Wang, Degradation of the UV-filter benzophenone-3 in aqueous solution using persulfate activated by heat, metal ions and light, Chemosphere, 2018, 196, 95–104 CrossRef CAS PubMed
.
- M. Izadifard, G. Achari and C. H. Langford, Degradation of sulfolane using activated persulfate with UV and UV-Ozone, Water Res., 2017, 125, 325–331 CrossRef CAS PubMed
.
- X. Lu, J. Zhao, Q. Wang, D. Wang, H. Xu, J. Ma, W. Qiu and T. Hu, Sonolytic degradation of bisphenol S: Effect of dissolved oxygen and peroxydisulfate, oxidation products and acute toxicity, Water Res., 2019, 165, 114969 CrossRef CAS PubMed
.
- H. Peng, L. Xu, W. Zhang, F. Liu, X. Lu, W. Lu, M. Danish and K. Lin, Different kinds of persulfate activation with base for the oxidation and mechanism of BDE209 in a spiked soil system, Sci. Total Environ., 2017, 574, 307–313 CrossRef CAS PubMed
.
- J. Monteagudo, A. Durán, R. González and A. Expósito, In situ chemical oxidation of carbamazepine solutions using persulfate simultaneously activated by heat energy, UV light, Fe2+ ions, and H2O2, Appl. Catal., B, 2015, 176, 120–129 CrossRef
.
- H. Dong, K. Hou, W. Qiao, Y. Cheng, L. Zhang, B. Wang, L. Li, Y. Wang, Q. Ning and G. Zeng, Insights into enhanced removal of TCE utilizing sulfide-modified nanoscale zero-valent iron activated persulfate, Chem. Eng. J., 2019, 359, 1046–1055 CrossRef CAS
.
- K. Hou, Z. Pi, F. Yao, B. Wu, L. He, X. Li, D. Wang, H. Dong and Q. Yang, A critical review on the mechanisms of persulfate activation by iron-based materials: Clarifying some ambiguity and controversies, Chem. Eng. J., 2021, 407, 127078 CrossRef CAS
.
- Y. Zong, X. Guan, J. Xu, Y. Feng, Y. Mao, L. Xu, H. Chu and D. Wu, Unraveling the Overlooked Involvement of High-Valent Cobalt-Oxo Species Generated from the Cobalt(II)-Activated Peroxymonosulfate Process, Environ. Sci. Technol., 2020, 54, 16231–16239 CrossRef CAS PubMed
.
- J. Lu, W. Dong, Y. Ji, D. Kong and Q. Huang, Natural organic matter exposed to sulfate radicals increases its potential to form halogenated disinfection byproducts, Environ. Sci. Technol., 2016, 50, 5060–5067 CrossRef CAS PubMed
.
- G. Fang, J. Gao, D. D. Dionysiou, C. Liu and D. Zhou, Activation of persulfate by quinones: free radical reactions and implication for the degradation of PCBs, Environ. Sci. Technol., 2013, 47, 4605–4611 CrossRef CAS PubMed
.
- A. Farhat, J. Keller, S. Tait and J. Radjenovic, Removal of persistent organic contaminants by electrochemically activated sulfate, Environ. Sci. Technol., 2015, 49, 14326–14333 CrossRef CAS PubMed
.
- Y. Liu, H. Guo, Y. Zhang, X. Cheng, P. Zhou, J. Wang and W. Li, Fe@C carbonized resin for peroxymonosulfate activation and bisphenol S degradation, Environ. Pollut., 2019, 252, 1042–1050 CrossRef CAS PubMed
.
- J. Chen, Y. Qi, X. Pan, N. Wu, J. Zuo, C. Li, R. Qu, Z. Wang and Z. Chen, Mechanistic insights into the reactivity of Ferrate(VI) with phenolic compounds and the formation of coupling products, Water Res., 2019, 158, 338–349 CrossRef CAS
.
- A. A. Dar, X. Wang, S. Wang, J. Ge, A. Shad, F. Ai and Z. Wang, Ozonation of pentabromophenol in aqueous basic medium: Kinetics, pathways, mechanism, dimerization and toxicity assessment, Chemosphere, 2019, 220, 546–555 CrossRef CAS
.
- W. Cao, Y. Yu, J. Wei, G. Al-Basher, X. Pan, B. Li, X. Xu, N. Alsultan, J. Chen, R. Qu and Z. Wang, KMnO4-mediated reactions for hexachlorophene in aqueous solutions: Direct oxidation, self-coupling, and cross-coupling, Chemosphere, 2020, 259, 127422 CrossRef CAS PubMed
.
- W. Xiang, R. Qu, X. Wang, Z. Wang, M. Bin-Jumah, A. A. Allam, F. Zhu and Z. Huo, Removal of 4-chlorophenol, bisphenol A and nonylphenol mixtures by aqueous chlorination and formation of coupling products, Chem. Eng. J., 2020, 402, 126140 CrossRef CAS
.
- X. Pan, J. Chen, N. Wu, Y. Qi, X. Xu, J. Ge, X. Wang, C. Li, R. Qu and V. K. Sharma, Degradation of aqueous 2, 4, 4′-Trihydroxybenzophenone by persulfate activated with nitrogen doped carbonaceous materials and the formation of dimer products, Water Res., 2018, 143, 176–187 CrossRef CAS PubMed
.
- H. Chen and K. C. Carroll, Metal-free catalysis of persulfate activation and organic-pollutant degradation by nitrogen-doped graphene and aminated graphene, Environ. Pollut., 2016, 215, 96–102 CrossRef CAS
.
- Q. Wang, L. Li, L. Luo, Y. Yang, Z. Yang, H. Li and Y. Zhou, Activation of persulfate with dual-doped reduced graphene oxide for degradation of alkylphenols, Chem. Eng. J., 2019, 376, 120891 CrossRef CAS
.
- X. Cheng, H. Guo, Y. Zhang, G. V. Korshin and B. Yang, Insights into the mechanism of nonradical reactions of persulfate activated by carbon nanotubes: Activation performance and structure-function relationship, Water Res., 2019, 157, 406–414 CrossRef CAS
.
- J. Peng, Z. Wang, S. Wang, J. Liu, Y. Zhang, B. Wang, Z. Gong, M. Wang, H. Dong and J. Shi, Enhanced removal of methylparaben mediated by cobalt/carbon nanotubes (Co/CNTs) activated peroxymonosulfate in chloride-containing water: Reaction kinetics, mechanisms and pathways, Chem. Eng. J., 2021, 409, 128176 CrossRef CAS
.
- H. Liu, J. Yao, L. Wang, X. Wang, R. Qu and Z. Wang, Effective degradation of fenitrothion by zero-valent iron powder (Fe0) activated persulfate in aqueous solution: Kinetic study and product identification, Chem. Eng. J., 2019, 358, 1479–1488 CrossRef CAS
.
- X. Cheng, H. Guo, Y. Zhang, X. Wu and Y. Liu, Non-photochemical production of singlet oxygen via activation of persulfate by carbon nanotubes, Water Res., 2017, 113, 80–88 CrossRef CAS PubMed
.
- J. Chen, W. Chen and D. Zhu, Adsorption of Nonionic Aromatic Compounds to Single-Walled Carbon Nanotubes: Effects of Aqueous Solution Chemistry, Environ. Sci. Technol., 2008, 42, 7225–7230 CrossRef CAS PubMed
.
- Y. Qi, J. Wei, R. Qu, G. Al-Basher, X. Pan, A. A. Dar, A. Shad, D. Zhou and Z. Wang, Mixed oxidation of aqueous nonylphenol and triclosan by thermally activated persulfate: Reaction kinetics and formation of co-oligomerization products, Chem. Eng. J., 2021, 403, 126396 CrossRef CAS
.
- N. Potakis, Z. Frontistis, M. Antonopoulou, I. Konstantinou and D. Mantzavinos, Oxidation of bisphenol A in water by heat-activated persulfate, J. Environ. Manage., 2016, 195, 125–132 CrossRef
.
- L. J. Xu, W. Chu and L. Gan, Environmental application of graphene-based CoFe2O4 as an activator of peroxymonosulfate for the degradation of a plasticizer, Chem. Eng. J., 2015, 263, 435–443 CrossRef CAS
.
- L. Zhou, M. Sleiman, C. Ferronato, J.-M. Chovelon, P. de Sainte-Claire and C. Richard, Sulfate radical induced degradation of β2-adrenoceptor agonists salbutamol and terbutaline: Phenoxyl radical dependent mechanisms, Water Res., 2017, 123, 715–723 CrossRef CAS PubMed
.
- J. Sharma, I. M. Mishra and V. Kumar, Mechanistic study of photo-oxidation of Bisphenol-A (BPA) with hydrogen peroxide (H2O2) and sodium persulfate (SPS), J. Environ. Manage., 2016, 166, 12–22 CrossRef CAS PubMed
.
- J. Ding, L. Bu, Q. Zhao, F. T. Kabutey, L. Wei and D. D. Dionysiou, Electrochemical activation of persulfate on BDD and DSA anodes: Electrolyte influence, kinetics and mechanisms in the degradation of bisphenol A, J. Hazard. Mater., 2020, 388, 121789 CrossRef CAS PubMed
.
- M. Wu, K. Fu, H. Deng and J. Shi, Cobalt tetracarboxyl phthalocyanine-manganese octahedral molecular sieve (OMS-2) as a heterogeneous catalyst of peroxymonosulfate for degradation of diclofenac, Chemosphere, 2019, 219, 756–765 CrossRef CAS PubMed
.
- K. Jing, D. Kong and J. Lu, Change of disinfection byproducts formation potential of natural organic matter after exposure to persulphate and bicarbonate, Water Res., 2020, 182, 115970 CrossRef CAS PubMed
.
- Q. Wang, X. Lu, Y. Cao, J. Ma, J. Jiang, X. Bai and T. Hu, Degradation of Bisphenol S by heat activated persulfate: kinetics study, transformation pathways and influences of co-existing chemicals, Chem. Eng. J., 2017, 328, 236–245 CrossRef CAS
.
- X. Haodan, W. Da, M. Jun, Z. Tao, L. Xiaohui and C. Zhiqiang, A superior active and stable spinel sulfide for catalytic peroxymonosulfate oxidation of bisphenol S, Appl. Catal., B, 2018, 238, 557–567 CrossRef
.
- P. Shao, X. Duan, J. Xu, J. Tian, W. Shi, S. Gao, M. Xu, F. Cui and S. Wang, Heterogeneous activation of peroxymonosulfate by amorphous boron for degradation of bisphenol S, J. Hazard. Mater., 2017, 322, 532–539 CrossRef CAS PubMed
.
- C. Chen, Z. Wu, S. Zheng, L. Wang, X. Niu and J. Fang, Comparative Study for Interactions of Sulfate Radical and Hydroxyl Radical with Phenol in the Presence of Nitrite, Environ. Sci. Technol., 2020, 54, 8455–8463 CrossRef CAS PubMed
.
- J. Yao, R. Qu, X. Wang, V. K. Sharma, A. Shad, A. A. Dar and Z. Wang, Visible light and fulvic acid assisted generation of Mn (III) to oxidize bisphenol A: The effect of tetrabromobisphenol A, Water Res., 2020, 169, 115273 CrossRef CAS PubMed
.
- R. Qu, X. Pan, C. Li, J. Liu, X. Wang, X. Zeng and Z. Wang, Formation of hydroxylated derivatives and coupling products from the photochemical transformation of polyfluorinated dibenzo-p-dioxins (PFDDs) on silica surfaces, Chemosphere, 2019, 231, 72–81 CrossRef CAS PubMed
.
- N. Zrinyi and A. L.-T. Pham, Oxidation of benzoic acid by heat-activated persulfate: effect of temperature on transformation pathway and product distribution, Water Res., 2017, 120, 43–51 CrossRef CAS PubMed
.
- P. Neta, V. Madhavan, H. Zemel and R. W. Fessenden, Rate constants and mechanism of reaction of sulfate radical anion with aromatic compounds, J. Am. Chem. Soc., 1977, 99, 163–164 CrossRef CAS
.
- C. vonSonntag, Degradation of aromatics by Advanced Oxidation Processes in water remediation: Some basic considerations, J. Water Supply: Res. Technol.--AQUA, 1996, 45, 84–91 CAS
.
- F. Minisci, A. Citterio and C. Giordano, Electron-transfer processes: peroxydisulfate, a useful and versatile reagent in organic chemistry, Acc. Chem. Res., 1983, 16, 27–32 CrossRef CAS
.
- X. Pan, L. Yan, C. Li, R. Qu and Z. Wang, Degradation of UV-filter benzophenone-3 in aqueous solution using persulfate catalyzed by cobalt ferrite, Chem. Eng. J., 2017, 326, 1197–1209 CrossRef CAS
.
- Q. Mei, J. Sun, D. Han, B. Wei, Z. An, X. Wang, J. Xie, J. Zhan and M. He, Sulfate and hydroxyl radicals-initiated degradation reaction on phenolic contaminants in the aqueous phase: Mechanisms, kinetics and toxicity assessment, Chem. Eng. J., 2019, 373, 668–676 CrossRef CAS
.
- J. Dec and J. M. Bollag, Dehalogenation of Chlorinated Phenols during Oxidative Coupling, Environ. Sci. Technol., 1994, 28, 484–490 CrossRef CAS PubMed
.
- B. Liu, M. Qiao, Y. Wang, L. Wang, Y. Gong, T. Guo and X. Zhao, Persulfate enhanced photocatalytic degradation of bisphenol A by g-C3N4 nanosheets under visible light irradiation, Chemosphere, 2017, 189, 115–122 CrossRef CAS PubMed
.
- F. Gao, Y. Li and B. Xiang, Degradation of bisphenol A through transition metals activating persulfate process, Ecotoxicol. Environ. Saf., 2018, 158, 239–247 CrossRef CAS PubMed
.
Footnote |
† Electronic supplementary information (ESI) available. See DOI: 10.1039/d1ew00545f |
|
This journal is © The Royal Society of Chemistry 2022 |
Click here to see how this site uses Cookies. View our privacy policy here.