DOI:
10.1039/C3RA43928C
(Paper)
RSC Adv., 2014,
4, 348-357
A novel strategy to design latent ratiometric fluorescent pH probes based on self-assembled SNARF derivatives†‡
Received
26th July 2013
, Accepted 24th October 2013
First published on
28th October 2013
Abstract
In this study, we describe a rational design strategy to develop a latent ratiometric fluorescent pH probe from self-assembled seminaphthorhodafluor (SNARF) derivatives in aqueous conditions. Analysis of the characteristics of SNARF derivatives with protected phenolic groups allowed classification of the threshold between the assembled and monomeric states according to the Hansch–Fujita hydrophobic parameters for a substituent inserted as a SNARF-OH phenolic-protecting group. The esterase-activated latent ratiometric fluorescent pH probe SNARF-OBn(OAc) was characterized in the application of tuneable small-molecule aggregation and disaggregation. Before esterase treatment, SNARF-OBn(OAc) formed self-assembled clusters, emitting no fluorescence. The significant fluorescence was observed after esterase treatment because the self-assembled clusters of SNARF-OBn(OAc) diffuse as SNARF-OH monomers, demonstrating the intracellular application of SNARF-OBn(OAc) as a latent ratiometric fluorescent pH probe for floating cells. Furthermore, to directly visualize the state of self-assembled SNARF derivatives for investigating the mechanism of their cellular uptake, SNARF-Dan was rationally designed and applied for cellular imaging. Through the cellular application of SNARF-Dan, it was suggested that the self-assembled SNARF derivatives were introduced into the cell via macropinocytosis.
Introduction
Fluorescence imaging is one of the most powerful techniques for the continuous observation of dynamic biochemical and cellular processes of living systems. Fluorescent probes that emit a specific intensity or wavelength in response to recognition of or reaction with a specific target biomolecule are very important for fluorescence imaging. Thus, fluorescent probes continue to be developed to support various approaches with different design strategies.1
When compared to simple intensity-changing fluorescent probes, ratiometric fluorescent probes typically offer the advantage of less sensitivity to errors associated with probe concentration, photobleaching, instrument sensitivity, and environmental effect.2 For intracellular usage, ratiometric fluorescent probes based on small-molecules must be loaded into the cells. Thus, probes having intrinsic fluorescence may obscure a change of intracellular signal if the background fluorescence of remaining extracellular probes was high.3 In order to reduce background fluorescence, it is necessary to remove culture medium or wash off excess extracellular probes. However, washing off excess probes introduces more variation in assays using adherent cells, thus making the assays cumbersome and not amenable to throughput screening. There are further difficulties in performing assays when using loosely adherent or floating cells.4 To overcome these disadvantages of using ratiometric fluorescent probes that are based on small-molecule fluorophores, it is necessary to minimize extracellular background fluorescence.
Since tracking the dynamics of intracellular pH (pHi) is crucial to understanding the mechanisms by which many physiological functions of cell and tissue activities are regulated,4,5 various types of fluorescent pHi probes have been developed. Some of these probes have been successfully applied to monitor pHi.6 Among these, SNARF (seminaphthorhodafluor) and its derivatives7–10 are most commonly used ratiometric fluorescent pH probes, because of their unique fluorescent characteristics: visible light excitation, dual-emission properties, and a neutral pKa region.5,7 These distinctive properties result from the critical phenolic substituent of SNARF when it exists in an equilibrium between the phenol and phenolate anion. SNARF derivatives in which the phenolic substituent is protected have spectral characteristics of the phenol form that are independent of pH.9b,c Interestingly, a certain kind of SNARF derivative shows unique off/on switching properties in fluorescence, which, as we previously demonstrated, causes it to self-assemble in aqueous condition.9b Based on these unique characteristics, we developed a sophisticated ratiometric fluorescent pH probe (referred to as the latent ratiometric fluorescent pH probe) that minimizes background extracellular fluorescence and activates in the intracellular environment to effectively monitor pHi in a ratiometric manner.9a,b Here, we describe several features of our latent ratiometric fluorescent pH probe. Detailed investigation of the characteristics of self-assembled SNARF derivatives, the structure–activity relationship of SNARF derivatives, the characteristics of esterase-activated latent ratiometric fluorescent pH probe in the test tube, their application to monitor the pHi change of floating cells, and the mechanism of internalization of self-assembled SNARF derivatives, reveals that the methodology used in this study is generally valuable for the rational design of latent ratiometric fluorescent pH probes based on self-assembled SNARF derivatives.
Results and discussion
Investigation of the fluorescent properties of SNARF-OH
Initially, we investigated the absorption and fluorescent properties of SNARF-OH in different solvent conditions, that is, in aqueous medium of pH 5.0 or 9.0, and in MeCN, as shown in Fig. 1. SNARF-OH produced maximal absorption and strong fluorescence at 544 nm and 583 nm in pH 5.0, and 573 nm and 627 nm in pH 9.0, respectively. The observed dual emission occurs because SNARF-OH exists in the quinoid form in aqueous conditions, and, as previously mentioned, because the phenolic substituent of SNARF-OH is critical for its dual-emission properties, in that an equilibrium exits between the acidic phenol form (written as SNARF(A)) and the basic phenolate form (written as SNARF(B)).6 In contrast, SNARF-OH in MeCN displayed no significant absorption above 400 nm and no fluorescence. This is because of an additional equilibrium involving the formation of a colourless and non-fluorescent lactone, which is the dominant form of SNARF-OH in hydrophobic environments (written as SNARF(L)).4,7 The results indicated that the fluorescence of SNARF derivatives can be controlled in a rational manner, if we can control the equilibrium of SNARF in aqueous media.
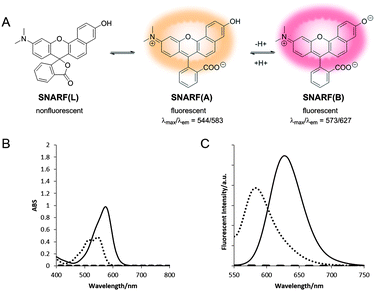 |
| Fig. 1 (A) Equilibria of SNARF-OH. (B and C) Solvent-environment-dependent spectra of SNARF-OH: (B) absorption spectra and (C) emission spectra. (Solid line: pH 9.0 in aqueous conditions, dotted line: pH 5.0 in aqueous conditions, dashed line: MeCN). [SNARF-OH] = 10 μM in pH 5.0 or pH 9.0; 10 mM Tris, Hepes, and acetate buffer or MeCN. | |
Characterization of the SNARF derivatives
SNARF derivatives in which the phenolic group of SNARF-OH were converted to methoxy or benzoxy substituents, termed SNARF-OMe or SNARF-OBn (UTX-38),9a respectively, were designed and synthesized (Fig. 2A). As shown in Fig. 2B, at pH 7.0, SNARF-OMe and SNARF-OBn showed different fluorescence properties compared to SNARF-OH. SNARF-OMe produced maximal absorption and fluorescence emission at 545 nm and 581 nm, respectively, whereas SNARF-OBn displayed no significant absorption above 400 nm, and no fluorescence emission (Fig. 2C and D).
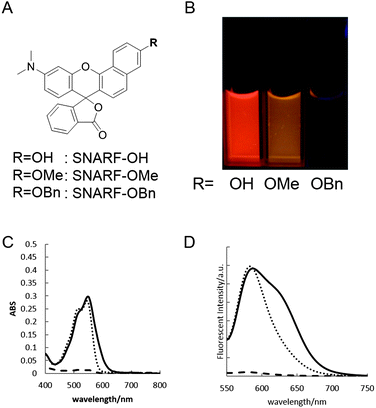 |
| Fig. 2 (A) The structure, (B) the photograph in pH 7.0 buffer, (C) the absorption spectra, and (D) the emission spectra (excited at 534 nm) of SNARF-OH, SNARF-OMe, and SNARF-OBn. [(C and D) solid line: SNARF-OH, dotted line: SNARF-OMe, dashed line: SNARF-OBn]. [SNARF derivatives] = 10 μM in pH 7.0 10 mM Tris, Hepes, and acetate buffer. | |
Like SNARF-OMe, SNARF derivatives with a protected phenolic group should have the spectral characteristics of SNARF(A), which are independent of pH.9c On the other hand, the absorption and emission spectral characteristics of SNARF-OBn indicated that SNARF-OBn existed as SNARF(L) in aqueous media through the formation of self-assembled clusters that themselves created a hydrophobic environment.9b To confirm the self-assembly of clusters, scanning electron microscopy (SEM) and dynamic light scattering (DLS) analysis were conducted (Fig. 3). SEM data showed the formation of particles of approximately 70 nm in size (Fig. 3A). DLS measurements of SNARF-OBn showed particles with a mean diameter of 79.1 ± 7.5 nm (Fig. 3B and Table 1). In the presence of NaCl (100 mM), the diameter of the particles was drastically increased (205.5 ± 43.1 nm) (Table 1). Correspondingly, a negligible DLS intensity was obtained in MeOH (Table 1). These results strongly suggested that the particles were self-assembled clusters of SNARF-OBn formed by hydrophobic interaction, and were then confirmed in conditions of physiological pH (from pH 5.0 to pH 9.0), and in wide concentration ranges (from 5 μM to 50 μM). The critical aggregate concentration of SNARF-OBn was determined to be <5 μM, indicating that SNARF-OBn stably formed self-assembled clusters in physiological aqueous conditions.
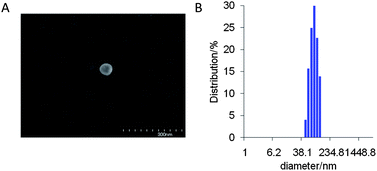 |
| Fig. 3 (A) SEM image of SNARF-OBn. (B) DLS analysis of SNARF-OBn. | |
Table 1 DLS data of SNARF-OBn under various conditions
Solution |
Concentration |
Diameter (nm) |
10 mM Tris, HEPES, and Acetate buffer,
10 mM Tris, HEPES, and Acetate buffer with 100 mM NaCl,
100% MeOH.
Cannot be determined.
|
pH 7.0a |
10 μM |
79.1 ± 7.5 |
pH 7.0 with 100 mM NaClb |
10 μM |
205.5 ± 43.1 |
MeOHc |
10 μM |
n.d.d |
pH 5.0a |
10 μM |
1209.8 ± 226.9 |
pH 6.0a |
10 μM |
228.7 ± 53.5 |
pH 8.0a |
10 μM |
84.0 ± 19.3 |
pH 9.0a |
10 μM |
87.2 ± 33.1 |
pH 7.0a |
5 μM |
67.5 ± 14.7 |
pH 7.0a |
20 μM |
81.9 ± 17.6 |
pH 7.0a |
30 μM |
72.5 ± 16.2 |
pH 7.0a |
40 μM |
80.1 ± 17.7 |
pH 7.0a |
50 μM |
91.5 ± 19.7 |
Various derivatives, in which the hydrophobicity of the substituent inserted as a protectant of phenolic hydroxyl was taken to be a measure of the calculated Hansch–Fujita hydrophobic parameter (π), were designed and synthesized (Table 2 and Fig. S1†).11 The absorption intensity and fluorescence emission of these derivatives were greatly altered depending on their π value (Fig. 4 and S2†). Derivatives with a π value lower than 0.0 (π < 0.0, classified as Group A), showed high absorption intensity and strong fluorescence. Derivatives with a π value above 0.3 (π > 0.3, classified as Group L), showed drastically decreased absorption intensity and fluorescence. These trends were in agreement with the observations of DLS intensity. These results indicate that the clusters were principally formed from SNARF(L). The threshold level between SNARF(A) as a fluorescent dispersion and SNARF(L) as non-fluorescent cluster falls in the π value range of 0.0–0.3. From these data, we can deduce that the fluorescent property of our designed SNARF derivatives can be rationally controlled by tuning the π value of the phenolic hydroxyl protective group. The self-assembly induced lactone formation (SAILac) especially, is a unique and useful characteristic that can be utilized to rationally control the fluorescent property of SNARF derivatives between the off and on states.
Table 2 Comparison of the π values of R, Abs, and the diameter-determined DLS analysis of SNARF derivatives
SNARF derivatives |
π of Ra |
Absb (544 nm) |
The diameter determined by DLSb |
The π value was calculated by ACD/LABS 12.0,
10 μM in pH 5.0 10 mM Tris, HEPES, and Acetate buffer.
Cannot be determined.
|
SNARF-OBn-βGal |
−1.85 |
0.194 |
n.d.c |
SNARF-OH |
−1.38 |
0.189 |
n.d.c |
SNARF-OβGal |
−1.01 |
0.200 |
n.d.c |
SNARF-OMe |
−0.72 |
0.225 |
n.d.c |
SNARF-OAM |
−0.62 |
0.191 |
n.d.c |
SNARF-OβGal(OAc) |
0.07 |
0.068 |
n.d.c |
SNARF-OBn(OAc) |
0.37 |
0.027 |
75.7 ± 7.7 nm |
SNARF-OBn(pNO2) |
0.76 |
0.021 |
123.6 ± 27.4 nm |
SNARF-OBn |
1.03 |
0.023 |
79.1 ± 7.5 nm |
SNARF-OP |
1.24 |
0.031 |
183.6 ± 25.6 nm |
SNARF-OBn-βGal(OAc) |
1.43 |
0.017 |
160.1 ± 10.6 nm |
SNARF-ONaph |
2.27 |
0.020 |
143.0 ± 26.4 nm |
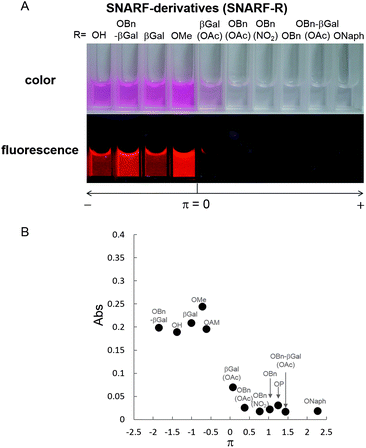 |
| Fig. 4 (A) Comparison of the absorption of SNARF derivatives divide by Hansch–Fujita hydrophobic parameter (π) of the inserted substituent of SNARF. (B) Relationships between the π value of the inserted substituent and the absorbance measured the following condition. [SNARF derivatives] = 10 μM in pH 5.0 10 mM Tris, HEPES, and acetate buffer. The detail of each π value and the absorbance of SNARF derivatives were shown in Table 2. The chemical structure of these SNARF derivatives were shown in Fig. S1.† The name of SNARF derivatives (SNARF-R) were shown inside the graph. | |
Characterization of esterase-activated latent ratiometric fluorescent pH probe
To study SNARF derivatives capable of being activated by enzymes, SNARF-OBn(OAc) (UTX-40)9b (π value of R is 0.90, Fig. 5A), with a p-acetoxybenzyl moiety directly linked to the SNARF scaffold through ether linkage, was designed as a latent ratiometric fluorescent pH probe that is activated by esterase. Before treatment with esterase, SNARF-OBn(OAc) displayed negligible absorption and fluorescence emission (Fig. 5B–D) because it existed as self-assembled clusters like SNARF(L). SEM and DLS data strongly supported the existence of self-assembled clusters (Fig. 5E and F and Table 2). When SNARF-OBn(OAc) was treated with esterase, an incremental change in absorption and fluorescence was observed (Fig. 5B–D), indicating that SNARF-OBn(OAc) (π = 0.90) was being converted from SNARF(L) to a mixture of SNARF(A) and SNARF(B) during the treatment (Fig. 5G). The self-assembled clusters diffused away as SNARF-OH monomers (π = −1.38). These results were strongly supported by size-exclusion chromatographic analysis performed before and after the esterase treatment (Fig. S3†). Before the esterase treatment, a faster effluent (fractions from 3 to 5) indicated the presence of self-assembled clusters. After the esterase treatment, on the other hand, a slower effluent (fractions from 22 to 35) was observed, with drastic decrease of the faster effluent, suggesting the presence of SNARF-OH. The disappearance of the self-assembled cluster after esterase treatment was also confirmed by the lack of significant SEM image and DLS intensity evidence (data not shown). This monomerizing property of SNARF-OBn(OAc) was then compared with the properties of SNARF-OAM (π = −0.62), which existed in the monomeric state, and was also converted to SNARF-OH through esterase treatment (Fig. S4†). SNARF-OAM was found to convert from SNARF(A) to a mixture of SNARF(A) and SNARF(B) with observed ratiometric fluorescent change during esterase treatment. The theoretical curves of ratiometric change of SNARF-OBn(OAc) and SNARF-OAM during ester hydrolysis are shown Fig. S5.† In the hydrolysis of SNARF-OAM, the ratio value was accurately indicated only after the yield of hydrolysis was over 80%. In other words, SNARF-OAM does not show the actual pH ratio value until 80% conversion has occurred. On the other hand, the correct ratio value was obtained after only 30% yield from the hydrolysis of SNARF-OBn(OAc). Incomplete ester hydrolysis is one of the most serious problems faced when using ratiometric fluorescent probes.4 Even with incomplete ester hydrolysis, however, the ratio values of SNARF-OBn(OAc) during esterase hydrolysis showed an ideal ratio value within 10 min (Fig. S6B†), although the kinetics of SNARF-OBn(OAc) hydrolysis reached saturation value around 80 min (Fig. S6A†). The results indicated that SNARF-OBn(OAc) is capable of determining the real ratio value under lower yields of hydrolysis. Such characteristics are useful for application of probes in cells with low levels of intracellular esterase activity.4
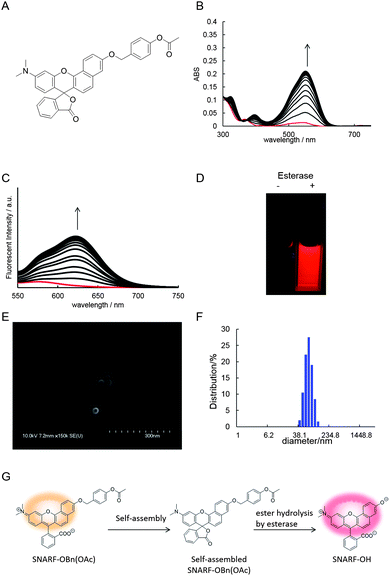 |
| Fig. 5 (A) The chemical structure of SNARF-OBn(OAc). (B) Real-time absorbance and (C) real-time fluorescence (excited at 534 nm) change via esterase-catalysed ester hydrolysis of SNARF-OBn(OAc). The red line is the spectrum before esterase treatment. (D) Photo of fluorescence of SNARF-OBn(OAc) before and after esterase treatment. (E) SEM image of SNARF-OBn(OAc). (F) DLS analysis of SNARF-OBn(OAc). (G) Schematic illustration of SNARF-OBn(OAc) as a latent ratiometric fluorescent pH probe that is activated by esterase. | |
Demonstration of latent ratiometric fluorescent pH probe for floating cells
The properties of SNARF-OBn(OAc) were evaluated for suitability as a pHi probe. Rat NR8383 macrophages were chosen as models of floating cells. Once pre-treated with organelle probes (mitochondria: Mito tracker Green FM, nuclei: Hoechst 33258), the NR8383 cells were treated with SNARF-OBn(OAc) and immediately observed by fluorescent microscopy (Fig. 6 and S8†). Immediately after the addition of SNARF-OBn(OAc), fluorescence signals characteristic of SNARF-OH were not observed either inside or outside the cells (Fig. 6C and S8C†). After 15 min of incubation, strong fluorescence was observed inside the NR8383 cells (Fig. 6D and S8D†). For the control experiment, NR8383 cells were treated with SNARF-OAM, which still exhibited the strong fluorescence identified with SNARF(A) (Fig. S7†). Strong fluorescence was observed not only inside the cells, but also in the medium immediately after SNARF-OAM was added, and after 15 min incubation. These results strongly indicated the advantages of SNARF-OBn(OAc) as a pHi probe. The actual pHi values of NR8383 cells in phosphate-buffered saline (PBS) (pH 7.2) were determined by using SNARF-OBn(OAc) without washing. Fig. 7 shows the fluorescent spectra of SNARF-OH inside the cell, which were measured in the conventional way by using a microplate reader. The ratio values, R (583 nm/627 nm), were determined to be 1.27 ± 0.04. A standard curve (Fig. 7B) was obtained by the conventional methods,12 and the pHi was accurately determined to be 6.96 ± 0.05, consistent with the known pHi range.13 When nigericin was added as an ionophore to equilibrate pHi and extracellular pH, the value of R became 1.13 ± 0.03. This R value correspond to the converted pH of 7.16 ± 0.04, which is the value of extracellular pH (pH 7.2). These results establish the superiority of SNARF-OBn(OAc) as a pHi probe in a high-throughput manner.
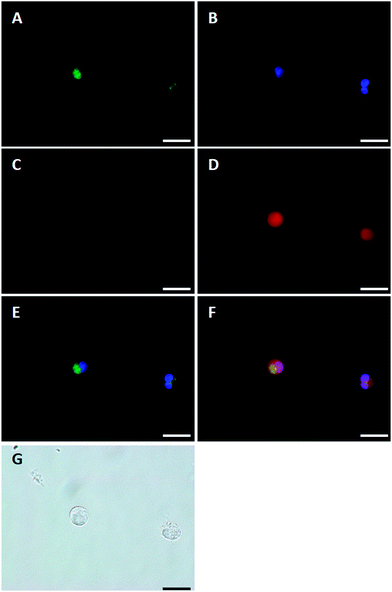 |
| Fig. 6 (A–F) Fluorescent images and (G) bright-field transmission of NR8383 cells pre-treated with Mito tracker Green® FM and Hoechst 33258 after the addition SNARF-OBn(OAc) to extracellular solution (FBS-containing F-12K medium). NR8383 cells were imaged simultaneously for (A) Mito Tracker Green® FM, (B) Hoechst 33258 and (C and D) SNARF-OBn(OAc) (C: 0 min, D: 15 min). The overlay image of (A), (B) and (C) is shown in (E). The overlay image of (A), (B) and (D) is shown in (F). (G) is a transmission image. The scale bar is 10 μm. | |
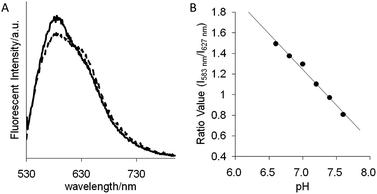 |
| Fig. 7 (A) Intracellular fluorescence spectra (excited at 488 nm) of SNARF-OBn(OAc)-derived SNARF-OH in NR8383 cells, measured by microplate reader before (solid line) and after (dashed line) addition of nigericin (10 mg mL−1) in assay buffer, pH 7.2. (B) The standard curve with pH values used to determine the intracellular pH. The assay buffer consisted of 140 mM KOH, 2 mM CaCl2, 1 mM MgCl2, 5 mM D(+)-glucose, 20 mM Tris, and MES. | |
Investigation of the mechanism of cellular uptake of self-assembled SNARF derivatives
In order to visualize the state of self-assembled SNARF derivatives directly, SNARF-Dan (π value of R is 1.81, Fig. 8A), a fluorescent self-assembled SNARF derivative with dansyl fluorophore directly linked to the SNARF scaffold, was designed. SNARF-Dan displayed absorption and fluorescence emission due to dansyl fluorophore, but negligible absorption and fluorescence emission due to SNARF in an aqueous condition (Fig. 8D–F). DLS measurements of SNARF-Dan supported the existence of self-assembled cluster with a mean diameter of 78.4 ± 17.7 nm (Fig. 8C). These results indicated that SNARF-Dan exists as a fluorescent self-assembled cluster in aqueous condition, as we expected. On the other hand, interestingly, SNARF-Dan displayed absorption and fluorescence emission from both dansyl and SNARF fluorophores in the presence of sodium dodecyl sulphate (SDS); that is, the self-assembled SNARF-Dan diffused in the presence of SDS and appeared the fluorescence of SNARF (Fig. 8D–F). As shown in Fig. 8B, the fluorescent colour change was observed in the absence and presence of SDS; that is, SNARF-Dan could monitor its own state in a ratiometric manner by monitoring both the fluorescence of dansyl and SNARF (Fig. 8G).
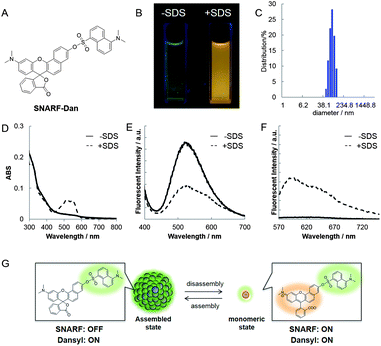 |
| Fig. 8 (A) The chemical structure of SNARF-Dan. (B) Photo of fluorescence of SNARF-Dan without and with 10 mM SDS. (C) DLS analysis of SNARF-Dan. (D) Absorbance and (E and F) fluorescence (excited at (E) 355 nm or (F) 534 nm) spectra in the absence or presence of SDS. The solid line and the dashed line indicate spectra obtained without (−) and with (+) 10 mM SDS, respectively. (G) Schematic illustration of colorimetric fluorescent change of SNARF-Dan between assembled and monomeric states. | |
Based on this characteristic of SNARF-Dan, the process of cellular uptake and the state of self-assembled SNARF-Dan inside the cell were investigated. HeLa cells were incubated with SNARF-Dan for 1 h, fluorescent microscopic analysis was performed after exchanging the medium. As shown in Fig. 9A, endosome-like punctate fluorescence from the dansyl fluorophore was observed.14 In contrast, negligible fluorescence from SNARF was observed inside the cells (Fig. 9B). When HeLa cells were sequentially treated with SNARF-Dan and SNARF-OBn(OAc), which responds to intracellular esterase, both endosome-like punctate fluorescence from the dansyl fluorophore (Fig. 9E) and diffuse fluorescence from SNARF, localized mainly in cytosol and mitochondria (Fig. 9F), were observed.9b These results suggested that SNARF-Dan was introduced inside the cell as the self-assembled clusters. In order to clarify the process by which cellular uptake of self-assembled clusters occur, the uptake efficiency of SNARF-Dan was compared under different temperature. When cells were incubated with SNARF-Dan for 1 h at 4 °C, the fluorescence due to self-assembled SNARF-Dan significantly decreased compared to that of cells incubated for 1 h at 37 °C (Fig. S9†). Since endocytosis is an energy dependent pathway that is often suppressed at low temperatures, these facts strongly suggest that the internalization pathway of self-assembled SNARF-Dan is endocytosis.14,15 We next determined whether cellular uptake of SNARF-Dan occurs through specific endocytic pathways. The cells pre-treated with inhibitors for a specific endocytic pathway were treated with self-assembled SNARF-Dan for 1 h, and the fluorescent image given by self-assembled SNARF-Dan was observed. As shown in Fig. S10,† the cellular uptake of self-assembled SNARF-Dan was inhibited by treatment with 5-(N-ethyl-N-isopropyl) amirolide (EIPA) or cytochalasin D. EIPA is a Na+/H+ exchange protein inhibitor, while cytochalasin D prevents actin polymerization, and both compounds have been documented to inhibit macropinocytosis.15,16 On the other hand, cellular uptake was not drastically inhibited by treatment with chlorpromazine, a cationic amphipathic drug that inhibits clathrin-mediated endocytosis, or nystatin, a sterol-binding agent that inhibits caveolae mediated endocytosis.15 These results strongly suggest that macropinocytosis plays a major role in the endocytic uptake of self-assembled SNARF-Dan.16
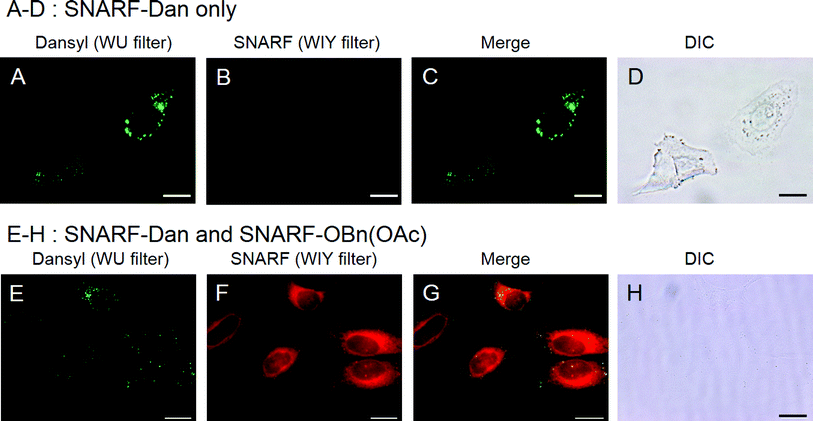 |
| Fig. 9 (A–C, E and F) Fluorescent images and (D and H) bright-field transmission of HeLa cells pre-treated with (A–D) SNARF-Dan only or (E–H) SNARF-Dan and SNARF-OBn(OAc) in the extracellular solution (FBS-containing EMEM medium). HeLa cells were imaged simultaneously for (A and E) dansyl fluorescence and (B and F) SNARF fluorescence (C and D). The overlay image of (A) and (B) is shown in (C). The overlay image of (E) and (F) is shown in (G). The scale bar is 10 μm. | |
Conclusions and perspective
The aggregation of small molecules has long plagued drug discovery efforts by acting as an unrestrained inhibitor.17 However, in recent years, there has been growing focus in various fields on the biological applications of the aggregates.18,19 The property of changing fluorescence, seen in fluorophores like aggregation-caused quenching (ACQ)20 and aggregation-induced emission (AIE),21 was one of the major applications of these aggregates, and was useful as the trigger of target-selective recognition or reaction. Thus far, several target-selective fluorescent nano-probes based on the aggregation of small molecules have been demonstrated.22,23 However, the aggregates of small molecules are relatively less studied, compared to the self-assembling aggregates of amphiphilic polymers, dendrimers, and biomacromolecules. The morphology is hard to control due to the diverse properties of small molecules. Furthermore, only a few examples of their cellular applications and characterization have been reported.9a,b,17b,22c Thus, the accumulation of the experimental example was desired.
We have successfully developed, for the first time, a rational design strategy for latent ratiometric fluorescent pH probes based on self-assembled SNARF derivatives in physiological aqueous conditions.9a,b The threshold between the assembled state and the monomeric state was classified by the Hansch–Fujita hydrophobic parameter, π, for the phenolic protecting group of SNARF-OH. Notably, the self-assembled states of the SNARF derivatives have unique characteristics. For example, the general aggregation state of xanthene derivatives induces absorbance spectral shifts, not the disappearance of xanthene-derived absorption.24 In contrast to the absorbance shifts, the disappearance of absorption caused by lactone formation may have the potential to provide higher S/N ratios and better photostability.25 The esterase-activated latent ratiometric fluorescent probe was designed as an application of tuneable aggregation/disaggregation, characterized in the test tube, and applied to measure the pHi of floating cell without washing. The design of the probe was based on the deprotection-driven disassembly of the phenolic group protected in SNARF derivatives, and the effective intracellular uptake of SNARF-derived fluorescent probes was confirmed in the case of SNARF-OBn(OAc), which was activated by intracellular esterase.9b Theoretically, the latent ratiometric fluorescent pH probe can be designed to target different activators by changing the substituent for the phenolic protecting group of SNARF-OH. In fact, SNARF-OBn(pNO2) (UTX-12) has been designed as a latent fluorescent pH probe that is activated in hypoxic conditions.9a Notably, the mechanism of cellular uptake of self-assembled SNARF derivatives was investigated by using rationally designed SNARF-Dan, which was able to monitor the transformation between the aggregation and disaggregation states in a ratiometric manner. The results suggested that enzyme-insensitive self-assembled SNARF-Dan was introduced into the cell via macropinocytosis, and that it existed as the self-assembled cluster for a long time period.26 Though the details of how endosomal escape after macropinocytic uptake of self-assembled SNARF derivatives occurs still remain ambiguous, the utility of self-assembled SNARF derivatives to minimize background fluorescence of outside of the cell is strongly indicated. We believe that the present strategy of designing a latent ratiometric fluorescent pH probe has great potential for use in tissue or in vivo as a targeted cell-specific ratiometric fluorescent pH probe.
Experimental
General
1H-NMR spectra were recorded on a JEOL JNM-EX400 spectrometer (400 MHz) using tetramethylsilane as the internal standard. Chemical shifts are reported in ppm. Coupling constants are reported in Hz. HRMS was measured on a JOEL JMS-700 mass spectrometer using the FAB method. Reactions were monitored by analytical TLC using Merck Silica Gel 60 F254 aluminium plates. Column chromatography was performed on Kanto Chemical Silica Gel 60 N (230–400 mesh). Absorption spectra were recorded on a Hitachi U3300 spectrometer or Infinite M200 from Tecan. Fluorescent spectra were recorded on a Hitachi F-4500 spectrometer or Infinite M200 from Tecan. Dynamic light scattering (DLS) was measured with a DLS-7000DL from Otsuka Electronics. SEM images were recorded on S-4700 Field-Emission Scanning Electron Microscope (Hitachi). Ion beam spattering was performed on ion sputter E-1020 (Hitachi). Fluorescent microscopy was measured with BIOREVO from KEYENCE or OLYMPUS IX71N-22FL/PH. Fluorescent spectra within cells were measured by the Infinite M200 from Tecan. All chemicals were purchased from Wako Pure Chemical Industries, Ltd (Osaka, Japan), Kanto Chemical Co., Inc. (Tokyo, Japan), Tokyo Chemical Industry Co., Ltd (Tokyo, Japan), Sigma-Aldrich Japan (Tokyo, Japan), or Invitrogen (Tokyo, Japan).
Synthesis of SNARF derivatives and preparation of stock solution
We have previously described the synthetic methods and characterization data for SNARF-OH, SNARF-OBn, SNARF-OAM, SNARF-OBn(OAc), and SNARF-OBn(pNO2).9a,b The details of the synthetic methods and characterization data for SNARF-OMe, SNARF-OP, SNARF-ONaph, SNARF-βGal(OAc), SNARF-βGal, SNARF-OBn-βGal(OAc), SNARF-OBn-βGal, and SNARF-Dan were described in ESI.† The stock solution (2 mM DMSO) of SNARF derivatives was prepared and used in subsequent experiments by diluting the assay buffer.
Calculation of the calculated Hansch–Fujita hydrophobic parameter (π)
The Hansch–Fujita hydrophobic parameter (π)11 was calculated using eqn (1).PRX and PRH represent the partition coefficients of a derivative and the parent molecule, respectively. The c
log
PRX and c
log
PRH (R = C6H5) were determined by ACD/LABS 12.0 software.
Absorption spectrophotometry
Absorption spectra of SNARF derivatives were measured in 10 mM Tris, HEPES, and acetate buffer from pH 5.0 to pH 10.0 at 20 ± 1 °C by U-3300 spectrometer (Hitachi) or Infinit M200 (Tecan).
Fluorescence spectrophotometry
Fluorescence spectra of SNARF derivatives were measured with excitation at 534 nm in 10 mM acetate, HEPES, and Tris buffer (pH 5.0, pH 7.0 or pH 9.0) at 20 ± 1 °C by F-4500 spectrometer (Hitachi) or Infinit M200 (Tecan).
DLS analysis
DLS measurements were taken by placing SNARF derivatives in 10 mM Tris, HEPES, and acetate buffer with corresponding pH, NaCl concentration, and SNARF derivative concentration.
SEM imaging
SNARF derivatives (10 μM) in distilled water were dropped on a glass plate (9 mm × 9 mm) and dried at room temperature for 24 h. Pt–Pd coating was applied for 30 s.
Size-exclusion chromatographic analysis
Esterase from porcine liver (PLE: E.C. 3.1.1.1.) was purchased from SIGMA Co. Ltd. SNARF-OBn(OAc) (10 μM) in 10 mM Tris, HEPES, and acetate buffer at pH 7.5, before and after addition of PLE (1.0 U mL−1), were loaded onto the pre-packed gel filtration column (PD-10, GE Healthcare) and eluted with 10 mM Tris, HEPES, and acetate buffer at pH 7.5. The eluted fraction was evaluated with UV-vis spectra by Infinit M200 (Tecan).
Ester hydrolysis of SNARF derivatives by esterase
The solution of SNARF-OBn(OAc) or SNARF-OAM (10 μM) in the assay buffer (10 mM HEPES buffer (pH 7.5)) at 20 ± 1 °C was mixed with esterase (PLE) (final concentration: 0.5 U mL−1). The fluorescence spectra (excitation wavelength: λex = 534 nm) and UV-vis spectra were monitored for 90 min. DLS measurements were performed by taking the sample of SNARF-OBn(OAc) and SNARF-OAM before or after esterase treatment for 90 min in a tubular-type cell.
Live cell imaging for NR8383 cells
NR8383 cells were cultured in F-12K medium without phenol red containing 15% (v/v) fetal bovine serum (witten for F-12K(+)) at 37 °C in 5% CO2. For live cell imaging by fluorescent microscopy, NR8383 cells (5 × 104 cells) in the late log phase were seeded in a 35 mm glass base dish. After growth in a CO2 incubator for 24 h, the cells were incubated for 40 min in F-12K(+) containing Hoechst 33258 (H3569: invitrogen) (500 μM) and Mito Tracker Green FM (M7514: invitrogen) (0.2 μM). Then, the culture medium was exchanged for 1 mL of flesh F-12K(+). Then, 1 mL of F-12K(+) containing SNARF derivatives (20 μM) was added (final concentration of SNARF derivatives was 10 μM). The cells were observed with fluorescent microscopy for 15 min.
Measurement of intracellular fluorescent spectra and calibration method of intracellular pH
For measurement of intracellular fluorescent spectra, the suspension of cells (1.0 × 106 cells per mL) was treated with 10 μM SNARF derivatives and incubated at 27 °C for 3 h. The suspension was diluted by assay buffer (140 mM KOH, 2 mM CaCl2, 1 mM MgCl2, 5 mM D(+)-glucose, 20 mM Tris and MES) having different pH values (pH 6.6–7.6), and transferred to a 96-well microplate (FIA black plate from Greiner bio-one), and then subjected to fluorescence analysis using Infinit M200 (Tecan). After the first measurement, nigericin (final concentration of 10 μg mL−1) was added to each well, the cells were incubated for 30 min, and a second measurement was taken. The calibration curves were constructed by slight modification of previously-reported methods9b introduced by Thomas and co-workers.12
Live cell imaging for HeLa cells
HeLa cells were cultured in Eagle minimum essential medium without phenol red containing 12.5% (v/v) fetal bovine serum and kanamycin (60 mg L−1) (written for EMEM(+)) at 37 °C in 5% CO2. For live cell imaging by fluorescent microscopy, HeLa cells (5 × 104 cells) in the late log phase were seeded in a 35 mm glass base dish. After growth in a CO2 incubator for 24 h, the cells were incubated for 1 h in EMEM(+) at 4 or 37 °C. Then, the culture medium was exchanged for 1 mL of flesh EMEM(+). Then, 1 mL of EMEM(+) containing SNARF-Dan (20 μM) was added (final concentration of SNARF-Dan was 10 μM). In the case of sequential treatment with SNARF-Dan and SNARF-OBn(OAc), separately prepared SNARF-OBn(OAc) solution (final concentration of SNARF-OBn(OAc) was 10 μM) was added after a moment. After 1 h incubation, the cells were washed with EMEM(+) and observed with fluorescent microscopy under the same machine condition (WU filter for dansyl fluorophore and WIY filter for SNARF fluorophore).
For the confirmation of the localization of self-assembled SNARF-Dan, the cells were co-stained with various organelles specific probes, such as LysoTracker Yellow HCK-123 (L-12491: invitrogen), ER tracker Green (E34251: invitrogen), NBD C6 ceramide complexed to BSA (N22651: invitrogen), Hoechst 33258 (H1398: invitrogen), Mito Tracker Green FM (M7514: invitrogen), DiD (D307: invitrogen), Dil-LDL (L-3482: invitrogen), or transferrin-Alexa 488 conjugate (T-13342: invitrogen). The filter condition were shown in the caption of Fig. S11 and S12.†
For the confirmation of the mechanism of cellular uptake of SNARF-Dan, the cells were pre-treated with various inhibitor (0.1 μg mL−1 cytochalasin D, 1 μM EIPA, 5 μg mL−1 chlorpromazine, or 25 μg mL−1 nystatin) for 1 h at 37 °C, followed by treatment with 10 μM SNARF-Dan and inhibitor at 37 °C. After 1 h incubation, the cells were washed with EMEM(+) containing inhibitor (without EIPA) and the cells were observed with fluorescent microscopy (WU filter for dansyl fluorophore) under the same machine condition.
Acknowledgements
This work was supported in part by a Grant-in-Aid for Scientific Research from the Ministry of Education, Culture, Sports, Science and Technology, Japan to E. N. (No. 20241051, 24107513). The authors thank Dr Atsushi Tabata (Tokushima university) for his help with the cell preparation, and Ms Maki Nakamura, Ms Emiko Okayama and the staff at the faculty of Tokushima university for measurement of NMR, and elemental analysis. We also thank Dr Ikuhiko Nakase (Osaka prefecture university) for his useful discussion with the mechanism of cellular uptake.
Notes and references
-
(a) L. Lavis and R. T. Rains, ACS Chem. Biol., 2008, 3, 142 CrossRef CAS PubMed;
(b) M. Kobayashi, R. Ogawa, P. Alford, L. Choyke and Y. Urano, Chem. Rev., 2010, 110, 2620 CrossRef PubMed;
(c) T. Ueno and T. Nagano, Nat. Methods, 2011, 8, 642 CrossRef CAS PubMed;
(d) J. Chan, S. C. Dodani and C. J. Chang, Nat. Chem., 2012, 4, 973 CrossRef CAS PubMed;
(e) T. Terai and T. Nagano, Pflügers Archiv - European Journal of Physiology, 2013, 46, 347 CrossRef PubMed.
-
(a) K. Kikuchi, H. Takakusa and T. Nagano, TrAC, Trends Anal. Chem., 2004, 23, 407 CrossRef CAS;
(b) A. P. Cemchenko, Anal. Biochem., 2005, 343, 1 CrossRef PubMed;
(c) N. Johnsson and K. Johnsson, ACS Chem. Biol., 2007, 2, 31 CrossRef CAS PubMed;
(d) H. Wang, E. Nakata and I. Hamachi, ChemBioChem, 2009, 10, 2560 CrossRef CAS PubMed;
(e) T. Doussineau, A. Schultz, A. L. Fernandez, A. Moro, S. Korsten, S. Trupp and G. J. Mohr, Chem.–Eur. J., 2010, 16, 10290 CrossRef CAS PubMed;
(f) A. P. Demchenko, J. Fluoresc., 2010, 20, 1099 CrossRef PubMed;
(g) L. Yuan, W. Lin, L. Zheng and S. Zhu, Acc. Chem. Res., 2013, 46, 1462–1473 CrossRef CAS PubMed.
- L. B. Bustin, Clin. Appl. Immunol. Rev., 2000, 1, 5 CrossRef.
-
R. P. Haugland, M. T. Z. Spence, I. D. Johnson and A. Basey, The Handbook: A Guide to Fluorescent Probes and Labeling Technologies, Molecular Probes, Eugene, OR, 10th edn, 2005 Search PubMed.
- J. Han and K. Burgess, Chem. Rev., 2010, 11, 2709 CrossRef PubMed.
-
(a) Y. Urano, D. Asanuma, Y. Hama, Y. Koyama, T. Barrett, M. Kamiya, T. Nagano, T. Watanabe, A. Hasegawa, P. L. Choyke and H. Kobayashi, Nat. Med., 2009, 15, 104 CrossRef CAS PubMed;
(b) J. Han, A. Loudet, R. Barhoumi, R. C. Burghardt and K. Burgess, J. Am. Chem. Soc., 2009, 131, 1642 CrossRef CAS PubMed;
(c) B. Tang, F. Yu, P. Li, L. Tong, X. Duan, T. Xie and X. Wang, J. Am. Chem. Soc., 2009, 131, 3016 CrossRef CAS PubMed;
(d) T. Myochin, K. Kiyose, K. Hanaoka, H. Kojima, T. Terai and T. Nagano, J. Am. Chem. Soc., 2011, 133, 3401 CrossRef CAS PubMed.
- J. E. Whitaker, R. P. Haugland and F. G. Prendergast, Anal. Biochem., 1991, 194, 330 CrossRef CAS.
-
(a) J. Liu, Z. Diwu and W.-Y. Leung, Bioorg. Med. Chem. Lett., 2001, 11, 2903 CrossRef CAS;
(b) E. Nakata, Y. Nazumi, Y. Yukimachi, Y. Uto, H. Maezawa, T. Hashimoto, Y. Okamoto and H. Hori, Bioorg. Med. Chem. Lett., 2011, 21, 1663 CrossRef CAS PubMed.
-
(a) E. Nakata, Y. Yukimachi, H. Kariyazono, S. Im, C. Abe, Y. Uto, H. Maezawa, T. Hashimoto, Y. Okamoto and H. Hori, Bioorg. Med. Chem., 2009, 17, 6952 CrossRef CAS PubMed;
(b) E. Nakata, Y. Yukimachi, Y. Nazumi, Y. Uto, H. Maezawa, T. Hashimoto, Y. Okamoto and H. Hori, Chem. Commun., 2010, 46, 3526 RSC;
(c) E. Nakata, Y. Yukimachi, Y. Nazumi, Y. Uto, T. Hashimoto, Y. Okamoto and H. Hori, Chem. Lett., 2010, 39, 734 CrossRef CAS.
-
(a) K. Honda, E. Nakata, A. Ojida and I. Hamachi, Chem. Commun., 2006, 4024 RSC;
(b) E. Nakata, H. Wang and I. Hamachi, ChemBioChem, 2008, 9, 25 CrossRef CAS PubMed;
(c) C. Annoni, E. Nakata, T. Tamura, F. F. Liew, S. Nakano, M. L. Gelmi and T. Morii, Org. Biomol. Chem., 2012, 10, 8767 RSC.
-
(a) C. Hansch, P. P. Maloney, T. Fujita and R. M. Muir, Nature, 1962, 194, 178 CrossRef CAS;
(b) R. N. Smith, C. Hansch and M. M. Ames, J. Pharm. Sci., 1975, 64, 599 CrossRef CAS;
(c) T. Fujita, J. Iwasa and C. Hansch, J. Am. Chem. Soc., 1964, 86, 5175 CrossRef CAS.
-
(a) J. A. Thomas, R. N. Buchsbaum, A. Zimniak and E. Racker, Biochemistry, 1979, 18, 2210 CrossRef CAS;
(b) J. Bond and J. Varley, Cytometry, Part A, 2005, 64, 43 CrossRef PubMed.
-
(a) M. C. Brahimi-Horn and J. Pouyssegur, FEBS Lett., 2007, 581, 3582 CrossRef CAS PubMed;
(b) P. Swietach, R. D. Vaughan-Jones and A. L. Harris, Cancer Metastasis Rev., 2007, 26, 299 CrossRef CAS PubMed.
- The localization of self-assembled SNARF-Dan was investigated by co-staining with various organelle-specific probes, such as LysoTracker, ER tracker, Mito Tracker, Hoechst 33258, and C6 ceramide. As shown in Fig. S11,† SNARF-Dan did not co-localize with these probes. When self-assembled SNARF-Dan was co-stained with DiD as the membrane marker, SNARF-Dan did not co-localize with DiD when incubated for a short period of time (1 h). Notably, SNARF-Dan partially co-localized with DiD, which was internalized during endocytic sorting of lipid when incubated long-term (12 h). These results suggested that the internalization of self-assembled SNARF-Dan occurs through a type of endocytosis.
-
(a) A. I. Ivanov, Methods Mol. Biol., 2008, 440, 15 CrossRef CAS;
(b) I. Nakase, T. Takeuchi, G. Tanaka and S. Futaki, Adv. Drug Delivery Rev., 2008, 60, 598 CrossRef CAS PubMed.
- The localization of self-assembled SNARF-Dan was examined by co-staining with Dil-LDL (indicator of receptor-mediated endocytosis) or transferrin-alexa 488 conjugate (indicator of clathrin-mediated endocytosis). As shown in Fig. S12,† SNARF-Dan did not co-localize with these indicators. These results suggested that SNARF-Dan is not internalized through these endocytic pathways.
-
(a) S. L. McGovern, B. T. Helfand, B. Feng and B. K. Shoichet, J. Med. Chem., 2003, 46, 4265 CrossRef CAS PubMed;
(b) S. C. Owen, A. K. Doak, P. Wassam, M. S. Shoichet and B. K. Shoichet, ACS Chem. Biol., 2012, 7, 1429 CrossRef CAS PubMed;
(c) M. F. Sassano, A. K. Doak, B. L. Roth and B. K. Shoichet, J. Med. Chem., 2013, 56, 2406 CrossRef CAS PubMed.
-
(a) Z. Yang, G. Liang and B. Xu, Acc. Chem. Res., 2008, 41, 315 CrossRef CAS PubMed;
(b) S. S. Agasti, S. Rana, M. H. Park, C. K. Kim, C. C. You and V. M. Rotello, Adv. Drug Delivery Rev., 2010, 62, 316 CrossRef CAS PubMed;
(c) J. Hu, G. Zhang and S. Liu, Chem. Soc. Rev., 2012, 41, 5933 RSC;
(d) L. Wang, L. L. Li, H. L. Ma and H. Wang, Chin. Chem. Lett., 2013, 24, 351 CrossRef CAS PubMed;
(e) B. Gong and Z. Shao, Acc. Chem. Res. DOI:10.1021/ar400030e , Article ASAP.
-
(a) H. Kasai, T. Murakami, Y. Ikuta, Y. Koseki, K. Baba, H. Oikawa, H. Nakanishi, M. Okada, M. Shoji, M. Ueda, H. Imahori and M. Hashida, Angew. Chem., Int. Ed., 2012, 51, 10315 CrossRef CAS PubMed;
(b) M. A. Azagarsamy, P. Sokkalingam and S. Thayumanavan, J. Am. Chem. Soc., 2009, 131, 14184 CrossRef CAS PubMed;
(c) Y. Takaoka, T. Sakamoto, S. Tsukiji, M. Narazaki, T. Matsuda, H. Tochio, M. Shirakawa and I. Hamachi, Nat. Chem., 2009, 1, 557 CrossRef CAS PubMed;
(d) M. A. Azagarsamy, V. Yesilyurt and S. Thayumanavan, J. Am. Chem. Soc., 2010, 132, 4550 CrossRef CAS PubMed.
-
(a)
J. B. Briks, Photophysics of Aromatic Molecules, Wiley, London, 1970, p. 718 Search PubMed;
(b) H. Kobayashi and P. L. Chyke, Acc. Chem. Res., 2011, 44, 83 CrossRef CAS PubMed;
(c)
J. R. Lakowicz, in Principles of Fluorescence Spectroscopy, Springer, New York, 3rd edn, 2006, p. 331 Search PubMed.
-
(a) Y. Hong, J. W. Y. Lam and B. Z. Tang, Chem. Commun., 2009, 4332 RSC;
(b) M. Wang, G. Zhang, D. Zhang, D. Zhu and B. Z. Tang, J. Mater. Chem., 2010, 20, 1858 RSC;
(c) Y. Hong, J. W. Y. Lam and B. Z. Tang, Chem. Soc. Rev., 2011, 40, 5361 RSC.
-
(a) K. Mizusawa, Y. Ishida, Y. Takaoka, M. Miyagawa, S. Tsukiji and I. Hamachi, J. Am. Chem. Soc., 2010, 132, 7291 CrossRef CAS PubMed;
(b) B. Wang and C. Yu, Angew. Chem., Int. Ed., 2010, 49, 1485 CrossRef CAS PubMed;
(c) K. Mizusawa, Y. Takaoka and I. Hamachi, J. Am. Chem. Soc., 2012, 134, 13386 CrossRef CAS PubMed.
-
(a) H. Shi, J. Liu, J. Geng, B. Z. Tang and B. Liu, J. Am. Chem. Soc., 2012, 134, 9569 CrossRef CAS PubMed;
(b) H. Shi, R. T. K. Kwok, J. Liu, B. Xing, B. Z. Tang and B. Liu, J. Am. Chem. Soc., 2012, 134, 17972 CrossRef CAS PubMed.
-
(a) O. Valdes-Aguilera and D. C. Neckers, Acc. Chem. Res., 1989, 22, 171 CrossRef CAS;
(b) M. Ogawa, N. Kosaka, P. L. Choyke and H. Kobayashi, ACS Chem. Biol., 2009, 4, 535 CrossRef CAS PubMed.
-
(a) T. Nagano, Proc. Jpn. Acad., Ser. B, 2010, 86, 837 CrossRef CAS;
(b) M. Kamiya, D. Asanuma, E. Kuranaga, A. Takeishi, M. Sakabe, M. Miura, T. Nagano and Y. Urano, J. Am. Chem. Soc., 2011, 133, 12960 CrossRef CAS PubMed;
(c) M. Sakabe, D. Asanuma, M. Kamiya, R. J. Iwatate, K. Hanaoka, T. Terai, T. Nagano and Y. Urano, J. Am. Chem. Soc., 2013, 135, 409 CrossRef CAS PubMed;
(d) G. Lukinavičius, K. Umezawa, N. Olivier, A. Honigmann, G. Yang, T. Plass, V. Mueller, L. Reymond, I. R. Corrêa, Jr, Z. G. Luo, C. Schultz, E. A. Lemke, P. Heppenstall, C. Eggeling, S. Manley and K. Johnsson, Nat. Chem., 2013, 5, 132 CrossRef PubMed;
(e) J. B. Grimm, A. J. Sung, W. R. Legant, P. Hulamm, S. M. Matlosz, E. Betzig and L. D. Lavis, ACS Chem. Biol., 2013, 8, 1303 CrossRef CAS PubMed.
- As shown in Fig. S11F,† the endosome-like punctate fluorescence emitted by the dansyl fluorophore component of SNARF-Dan still remained after 12 h incubation.
Footnotes |
† Electronic supplementary information (ESI) available: Chemical structure of SNARF derivatives, detail of synthesis, spectra data and cell image. See DOI: 10.1039/c3ra43928c |
‡ Dedicated to Professor Seiji Shinkai on the occasion of his 70th birthday. |
|
This journal is © The Royal Society of Chemistry 2014 |
Click here to see how this site uses Cookies. View our privacy policy here.