DOI:
10.1039/D3RA07059J
(Review Article)
RSC Adv., 2024,
14, 9571-9586
Recent advancements in the specific determination of carcinoembryonic antigens using MOF-based immunosensors
Received
17th October 2023
, Accepted 29th February 2024
First published on 21st March 2024
Abstract
Carcinoembryonic antigens (CEAs) are prominent cancer biomarkers that enable the early detection of numerous cancers. For effective CEA screening, rapid, portable, efficient, and sensitive diagnosis approaches should be devised. Metal–organic frameworks (MOFs) are porous crystalline materials that have received major attention for application in high-efficiency signal probes owing to their advantages such as large specific surface area, superior chemical stability and tunability, high porosity, easy surface functional modification, and adjustable size and morphology. Immunoassay strategies using antigen–antibody specific interaction are one of the imperative means for rapid and accurate measurement of target molecules in biochemical fields. The emerging MOFs and their nanocomposites are synthesized with excellent features, providing promising potential for immunoassays. This article outlines the recent breakthroughs in the synthesis approaches of MOFs and overall functionalization mechanisms of MOFs with antigen/antibody and their uses in the CEA immunoassays, which operate according to electrochemical, electrochemiluminescent and colorimetric techniques. The prospects and limitations of the preparation and immunoassay applications of MOF-derived hybrid nanocomposites are also discussed at the end.
1. Introduction
Over the past few years, massive incidences of cancer triggered its early diagnosis by the quantification of specific substances, i.e. biomarkers, produced by tumor cells. Carcinoembryonic antigens (CEAs) are a type of glycoprotein involved in cell adhesion, which are produced during fetal development.1 The CEA is a highly sensitive and specific tumor marker for pancreatic, breast, and colon cancers. The risk of these diseases increases when the level of CEAs exceeds 5 ng mL−1 in the serum/plasma. In the presence of a CEA identified as a tumor marker, the body produces antibodies (Abs) to fight them. For the effective treatment and clinical diagnosis of cancer, the accurate and rapid determination of CEAs is crucial. Furthermore, after adjuvant chemotherapy or surgical resection, the CEA concentration determination can help monitor and identify metastatic cases or tumor recurrences.2 Hence, the selective and sensitive determination of CEAs has garnered rapid advancement due to its significance in clinical medicine.
Abs as developing critical immunoglobulin macromolecules play vital roles in diverse applications (e.g., biosensing, catalysis, disease treatment, medical diagnosis, and scientific research). Abs exhibit the merits of low immunogenicity, high solubility, conformational and colloidal stability, high biodegradability, and specificity in sophisticated and fundamental reactions. Despite these advantages, the propensity for degradation/aggregation and inferior biophysical stability pose significant challenges to the expansion of the applicability of biomolecular techniques.3 Moreover, therapeutic proteins can be degraded by perturbation environments such as chemical reagent corrosion, mechanical force impact, and temperature alteration leading to low efficacy.4 Nevertheless, they can further cause biosafety risks and impose extra costs for Ab applications. For instance, the employment of sugars for lyophilization and vitrification of proteins can cause unpredictable immunogenicity or aggregation owing to the uncontrollable crystallization of sugars.5 Furthermore, polymer additives as enhancers of thermal stability of proteins maintained the possible immunological risks and separation difficulty.6 Therefore, the development of controlled conditions and efficient and facile approaches is an urgent requirement for the stabilization of these biopharmaceutical proteins. Fortunately, the immobilization of Abs on diverse supports has been extended significantly in recent years to overcome the above-mentioned complications. In diagnostic immunoassays, Abs are immobilized on semiconductor chips, electrodes, or optical fibers via various strategies by the formation of distinct linkages to improve the catalytic and stability performance of Abs. Since the loss of biological activity upon immobilization of Abs is problematic in many cases, the development of efficient immobilization supports is a study hotspot in biomedical analysis. An ideal support platform must provide a biocompatible environment that prevents Ab aggregation through chemical, mechanical, and thermal stability along with a high capacity to load Abs. In recent investigations, the commonly employed substrates for the immobilization of Abs include molybdenum disulfide (MOS2), nanoparticles (NPs), polymers, graphenes, and metal–organic frameworks (MOFs), which cannot only improve their stability and activity but also reduce the inhibition of products. At this state, MOFs formed by organic ligands and metal nodes are considered as a novel class of crystalline solid-state matrixes for the immobilization of Abs despite several identified materials having a large specific surface area. Facile functionalization, tunable pore size and structure, high porosity, selectivity, and stability of MOFs help reduce or eliminate the possible leakage of the embedded molecules due to the interactions of host-adsorbed biomolecules and enhanced stability. Additionally, Abs can be immobilized in MOFs through plentiful in situ and post-synthetic procedures such as biofunctionalization, in situ encapsulation, diffusion, surface immobilization, and embedding based on covalent attachment and host–guest interactions.
This study reviewed recent progresses in CEA biomarker detection using immunosensors based on MOF-derived composites, and alongside that, examined the performance of different techniques, which developed according to this concept. At first, various strategies for Ab immobilization by MOF carriers are studied, in particular in situ encapsulation, diffusion into the pores, and surface bioconjugation (e.g., covalent linkage and adsorption). Particularly, the recent technological hotspots are also considered in the field of Ab immobilization by MOFs for Ab immobilization and multi-Ab immobilization. Then, the applications of MOFs as immobilization supports for Abs in the immunosensing of CEA have also been discussed, such as electrochemical, electrochemiluminescence, colorimetric, and other sensing approaches. Finally, the further opportunities and challenges for Ab immobilization in MOFs and their sensing applications are also reviewed.
2. Selection of MOFs for antibody immobilization (MOF/Ab biocomposites)
Abs, naturally produced glycoproteins, are used as biorecognition elements in developing immunosensors towards various antigens including bacteria, antibiotics, and proteins.7 Inspired by the selectivity, sensitivity, and fast response of Ab-antigen reactions, Abs are widely used in sensing applications. Still, one of the key problems hindering the reproducibility and stability performance of the sensors is the immobilization of Abs. In this regard, MOFs as crystalline porous materials with multiple functional groups such as sulfonates, phosphates, amines, nitrates, and carboxylates are ideal matrix materials to immobilize several Abs with high stability and immobilization capacity.8 In biological applications, some unique properties are advantageous for MOFs, including stability in biofluids, non-destructive sensing, biodegradability, and low toxicity, along with environmental matters in their synthesis.9 Using several post-synthetic modification methods, rational design of linkers and nodes, and synthetic conditions, MOFs with these properties and different chemical functions can be faithfully personalized.10 At the first step of porous MOF development, the micro-sized bulk crystalline structure limited the catalytic activity and dispersibility in solutions in the sensing field. Therefore, a decrease in particle size (nano-scaled MOFs) would improve the analyte diffusion and electroactive accessibility leading to enhanced sensitivity of sensors.11 Typically, nano-MOF-based sensors with smaller sizes and a large number of active sites exhibit a low limit of detection (LOD) and enhanced sensitivity.12 Recently, Ab immobilization on MOFs with various topologies has been remarkably stated with the prosperous development and in-depth research of MOFs in sensing fields due to their significant properties.13 At the state of Ab integration, the large specific surface area of MOFs (even up to 6000 m2 g−1) endows them with more accessible active sites, resulting in higher Ab loading capacity and enhanced catalytic activity. Besides, benefitting from the adjustable structure and aperture size of MOF scaffolds, Abs can efficiently match them. In a typical example, Wang and coworkers fabricated MOFs with tunable sizes by altering the solvent composition in the reaction system.12 Such a decrease in the size of MOFs to nanoscale improved not only the electrocatalytic activity but also the dispersibility in solutions. Owing to the above-mentioned advantages of nanoscaled MOFs, the applicability, sensitivity, and assay time of MOF biocomposite-based sensors can be efficiently enhanced.14 Furthermore, the water stability of MOFs is another key factor for MOF–Ab-based sensing applications. Although Abs are soluble in water and destructible in organic solvents, MOFs possess weak metal–ligand bonds in aqueous solutions, which requires further research on the formation of water-stable MOFs. On account of the strong water stability, two types of MOF frameworks have been synthesized.15 The first category is the metal carboxylate framework containing high-valence metal ions such as Zr4+, Cr3+, and Fe3+ possessing high coordination number and charge density. Benefitting from this feature, MOFs with stable coordination bonds between ligands and metal ions can be made along with providing a rigid structure.14,16 The second category is the metal azolate framework consisting of soft nitrogen-donor ligands, including pyrazolates, triazolates, imidazolates, and tetrazolates, which can react with soft divalent metal ions to build strong MOF structures.17 With recent advances in the design of novel and porous MOFs by choosing proper organic ligands, metal ions, and synthesis approaches, a variety of MOFs with different sensing properties have been developed for conjugation with biocomposites such as Abs. Moreover, Ab protection is another effect of the highly ordered MOFs that increases their operability in practical applications by making them steadier in extremely high alkaline or acidic media, organic solvents, and high temperatures. Last but not least, the reusability of Abs can be ensured by immobilization in MOFs. According to the concept of MOFs for Ab immobilization, extensive research has been reported using MOFs as carriers to immobilize Abs through diverse approaches to construct different immunosensors with exceptional specificity and selectivity.
2.1. Surface bioconjugation
Abs were incorporated into MOFs for capturing the target antigens, which produces an optical or electrochemical signal. In this part, the approaches of fabricating MOF–Ab biocomposites are mainly discussed based on the interaction mode between the MOF matrix and Abs. Generally, Ab-conjugated MOFs are synthesized via post-synthetic modification, which comprises chemical or physical immobilization of Abs on pre-prepared MOFs.9 Basically, chemical immobilization of Abs is reached by the chemical reaction between Abs and the unreacted functional groups of MOFs using cross-linkers or coupling agents. In contrast, simple impregnation between Abs and MOFs is involved in the solution phase at the physical modification state. The post-synthetic bioconjugation strategies of Abs–MOFs are usually divided into two types including covalent attachment and surface adsorption.
2.1.1. Adsorption. Generally, surface adsorption is the commonly used method benefiting from the advantages of broad applicability and simple operation. This strategy commonly uses weak interactions such as hydrophobic interactions, π–π stacking, electrostatic, hydrogen bonding, and van der Waals forces to anchor Abs on the MOF surface.18 Generally, Abs are mixed physically with pre-synthesized MOFs under mild conditions without any synthesis requirements. Benefiting from all these advantages, various types of MOFs can be used as matrices to immobilize Abs via surface adsorption. In a typical example of a surface adsorption procedure, an impedimetric sensor was developed based on Ab–MOF biocomposites for the determination of vomitoxin and salbutamol by Liu et al.19 The impedance signal was obviously increased when the biocomposite-modified electrode captured the vomitoxin or salbutamol antigens. Benefitting from the higher Ab loading capacity of MOFs, a lower LOD was obtained for salbutamol (0.4 pg mL−1) and vomitoxin (0.7 pg mL−1). Upon the addition of different interferences, the impedance response represented no change indicating good specificity.
2.1.2. Covalent linkage. In comparison with surface adsorption, the covalent attachment is much stronger and has higher linkage stability. Typically, the covalent linkage results from the formation of peptide bonds via a condensation reaction between amino and carboxyl groups, which originates from Abs and MOFs.20 Additionally, the covalent attachment could be derived from the cross-linking process between amino groups on MOFs and biomolecules using a crosslinking polymer, i.e., glutaraldehyde. As another type of covalent attachment, noble metal NPs can be applied as immobilization supports to anchor Abs benefiting from the electrostatic adsorption between noble metal NPs and Abs. In this regard, Bhardwaj et al.21 developed an Ab–MOF sensor using covalent linkages for the determination of atrazine. To form an immunosensing platform, atrazine Abs were conjugated to the polyaniline-based thin film substrate with a high electrical conductivity. The designed immunosensor demonstrated a low LOD of 0.01 nmol L−1 for the conductometric detection of atrazine. No significant change in electrical signals of the platform in the presence of several other pesticides has been directed toward their high specificity.
2.2. Diffusion into MOFs
Pore diffusion is a strategy in which biomolecules are adsorbed into the porous structure of MOFs instead of their surface as an immobilization matrix.22 In this method, MOF structures are pre-synthesized and then the infiltration of Abs into the MOF cavity can occur under biocompatible conditions. This process profits from several advantages for the fabrication of biocomposites. First, Abs can highly load into the pores of MOFs by virtue of the mesoporous cavity and large pores of MOFs. Second, the numerous supramolecular interactions such as electrostatic interactions, π–π interactions, and hydrophilic interfacial interactions between framework ligands and Abs simplify the rapid diffusion. Third, the MOFs offer a protective layer for immobilized Abs preserving the activity of biomolecules and avoiding the leakage of Abs. Since the crystallization of MOFs is independent of the infiltration process, MOFs with excellent aqueous stabilities, low defects, and highly crystalline structures could be synthesized under hydro/solvothermal conditions expanding their applicability.
2.3. In situ encapsulation
The in situ encapsulation approach introduces biomolecules, e.g. Abs, during the synthesis process of the framework structures to form MOF biocomposites.23 MOFs should be formed under mild conditions considering the fragile nature of biomolecules to avoid destroying the activity of immobilized biomolecules. From the view of the growth mechanism, the in situ encapsulation strategy commonly occurs through two competing strategies of biomimetic mineralization and co-precipitation. In the biomimetic mineralization approach, the biomolecule is directly mixed with the MOF and its surface facilitates the nucleation of MOF precursors to yield biomolecule@MOF. For the co-precipitation method, biological species coated with capping agents such as polyvinyl pyrrolidone are passively encapsulated into the MOF.
Because post-synthetic methods are generally employed for the fabrication of Ab-conjugated MOFs, the specific control of reaction conditions including temperature and pH is essential. During Ab conjugation, various buffers could be utilized to maintain reaction parameters such as pH. Tris(hydroxymethyl)aminomethane, phosphate buffer (PB), phosphate-buffered saline (PBS), and 2-(N-morpholino) ethane sulfonic acid (MES) are the frequently used buffers. Apart from the used post-synthesis modification approaches for the attachment of Abs to MOFs, the activation of pending groups is frequently explored because of easy activation and large availability. In a work conducted by Kumar et al., anti-bovine serum albumin (anti-BSA) was covalently conjugated to a Zn-based MOF in which their pendent group was activated. Pendant group activation was accomplished using 1-ethyl-3-(3-dimethylaminopropyl)carbodiimide (EDC) cross-linkers and MES buffers. To keep the pH of the reaction solution during covalent conjugation, the PBS buffer was used. The interaction of EDC and carboxyl groups forms active intermediates such as O-acylisourea, and this active intermediate could be hydrolyzed to produce a carboxyl group in the absence of an amine group. At this state, the conjugation efficiency was decreased by the hydrolysis process of these intermediates; hence, using N-hydroxysuccinimide (NHS) could be advantageous to avoid hydrolysis. To this end, the formation of NHS esters by the interaction of intermediate components and NHS served as an active site for amines. It is found that NHS ester reacts with the nearby amine group to form an amide bond. The EDC–NHS was exploited further for the conjugation between carboxylic groups of Abs and amine-functionalized MOFs.24 As such, the sensitivity of the sensor is enhanced by the oriented attachment of Abs, which increases the available active sites on the sensing substrate. For investigating the conjugation status of Abs and MOFs, some spectroscopic procedures such as FTIR and UV-vis spectroscopies could be more deliberately used. To this end, the completion of bioconjugation between Abs and MOFs can be confirmed by the appearance of special bonds and also a shift in the band of UV-vis spectra.
3. Immunosensing approaches for MOF-derived nanocomposites
The combination of sensing technology with a specific and sensitive immunoassay is of the immunosensing analysis strategies, which are widely investigated for sensing and monitoring. New ideas are provided for the development of efficient and novel immunoassay strategies due to the remarkable properties of MOF–Ab materials such as (i) high surface area,25 (ii) Ab protection in various media,9 (iii) high reproducibility, recyclability, and stability,26 and (iv) structural flexibility and ease of synthesis.27 Based on the type of detection, various immunosensors such as colorimetric, electrochemical, and electrochemiluminescent (ECL) immunosensors are constructed and their main features are summarized.
3.1. Electrochemical immunosensors
Electrochemical immunoassays have emerged as valuable analytical techniques due to their cost-effectiveness, broad dynamic range, and remarkable sensitivity. Several electrochemical immunoassay platforms have attracted considerable attention in recent years. According to the detection principle, electrochemical immunosensing is divided into voltammetry (square wave, linear sweep, cyclic, and differential pulse), amperometry, and impedance subclasses. In voltammetry, information about the species is obtained by the current, resulting from the applied time-dependent potential to an electrochemical cell. In amperometry, a pulsed or constant potential is typically applied to the working electrode after measuring the resulting current as time-dependent. Moreover, impedance spectroscopy is based on the characterization of frequency-dependent electrical impedance at alternating currents. The integration of MOF-based composites has been demonstrated to enhance the detection capabilities of electrochemical immunoassays. The main focus of these approaches is on two fundamental elements: (i) modification of electrodes using MOF composites and (ii) fabrication of immunoprobes using MOF nanocomposites. The thoughtful design of immunoprobes based on the MOF-derived materials is advantageous in improving the electrochemical sensing performance. On the one hand, MOFs possess a meticulously arranged pore building that affords a large surface area for loading electrochemical active substances, thereby augmenting the sensitivity of the nanoprobes. On the other hand, MOFs have the capability to serve as a protective layer to safeguard materials from external factors, thereby enhancing the stability of the probes.28 In this regard, Biswas et al.29 developed a samarium (Sm)-MOF-based electrochemical label-free immunosensor for the sensitive determination of a colon cancer biomarker. For that, highly stable MOFs were synthesized by a solvothermal process using different carboxylate ligands such as 1,3,6,8-tetra(4-carboxyphenyl)pyrene (TBPy), meso-tetra(4-carboxyphenyl)porphyrin (TCPP), and trimesic acid (TMA). All the rod-shaped Sm–TMA MOFs, spherical-shaped Sm–TBPy MOFs, and cubic-shaped Sm–TCPP MOFs exhibited respective electrocatalytic activity. The immunosensing performances and electrocatalytic activity were significantly affected by the density of Sm(III) unsaturated coordination sites. Under optimal conditions, the designed immunosensors were used for the determination of CEAs with a lower LOD and a wide linear detection range in human serum samples. In another work, Huang et al.30 developed an electrochemical immunosensor based on gold nanoparticle (AuNP)@Cu/Co-MOF nanosheets for the sensitive determination of CEAs and alpha-fetoprotein (AFP). Here, AuNP@Cu-MOF and AuNP@Co-MOF nanosheets as redox probes were used to label different Abs. For blocking the remaining potential active sites, glucose oxidase was used. In the presence of glucose oxidase, glucose was in situ catalyzed to H2O2 using MOF-based materials. To obtain amplified signals, AuNP@Cu-MOF- and AuNP@Co-MOF-derived materials were used for further catalysis of H2O2. The results showed a lower LOD of 0.31 pg mL−1 with a linear range of 0.001–80 ng mL−1 for CEA determination. Remarkably, the prepared electrochemical immunoassay proposed cost-effective, simple, and rapid analysis of CEA in biological samples. To solve the problems of fast decrease in response signal and unstable signal of traditional electroactive substances, Jiang et al.31 developed another MOF-based electrochemical immunosensor using a tetrahydroxy-1,4-benzoquinone (THQ) ligand and Cu2+ (Cu–THQ MOF)-modified AuNPs under green and mild conditions for CEA determination in real serum. For that, a uniform layer of AuNPs was deposited on the surface of a glassy carbon electrode. The electrode was incubated at 4 °C after the addition of Abs. To block the non-specific active sites on Cu–THQ MOF/AuNPs, bovine serum albumin (BSA) was inserted. Then, the as-prepared CEA secondary Ab tag was added to the antigen-immersed electrodes to construct the electrochemical immunosensor. Here, the coordination of Cu2+ with small THQ in MOFs not only improved the sensitivity, stability, and conductivity but also increased the redox activity of THQ in comparison to the traditional electroactive substance. The π–π stacking of the interlayer ligand THQ and Cu2+ improved the stability and conductivity of Cu–THQ MOFs, endowing them with promising electrochemical activity and conductivity. The results revealed good linearity for the CEA determination in the range of 1.0 fg mL−1 to 40 ng mL−1, a low LOD of 0.477 fg mL−1, and a high sensitivity of 14.81 μA (ng mL−1) cm−2.
Despite the immobilization of immune molecules and signal enhancement strategy in the development of different MOF-based nanocomposites for sensitive CEA determination, the improvement of sensitivity is problematic in some cases. For example, the insulating layer created by the immune complex on the surface of the electrode can hinder electron transfer. Additionally, the sensitivity could be affected by the high impedance of the signal probe.32,33 Considering these issues, Liu and coworkers34 employed Cu-MOF–toluidine blue (TB) sealed with a polydopamine (PDA) coating as an immunosensing probe for the quantification of CEAs in human serum. Cu-MOFs were used in this electrochemical immunoassay due to their superior catalytic activity, dense porous structure, unique electric conductivity, and well-defined configuration. PDA-covered TB molecules with active nitrogen atoms were trapped inside Cu-MOFs via electrostatic forces. In the proposed sandwich immunosensor, chitosan and carboxylic multiwall carbon nanotubes (MWCNs) were employed to immobilize anti-CEA (Ab1) and improve the conductivity of the electrode. In the presence of CEA, the immunoreaction between the Ab1–MWCNT-modified electrode and Cu-MOFs–TB/PDA–CEA Abs (Ab2) generated the current response. Here, the sensitivity of this strategy was improved by the destruction of the immune complex and the probe using HCl, making the distance between the sensing electrode and TB closer to produce a higher response current. The results indicated a low LOD of 3.0 fg mL−1 with a wide linear range of 20 fg mL−1 to 200 ng mL−1. This strategy was successfully employed for the construction of a sensitive, stable, and selective electrochemical CEA immunosensor.
More recently, Huang's group has reported a high-performance label-free electrochemical immunosensor using an anti-CEA/Ag-MOF/GO composite for early CEA analysis.35 Study of the morphology and crystal structure of the altered electrode through XRD and FE-SEM indicated that the successful combination of GO nanosheets and Ag-MOF nanoparticles led to a significant surface area suitable for capturing antibodies. Electrochemical tests exploiting the DPV and CV techniques revealed that the immunosensor's selectivity, stability, and sensitivity were enhanced via the anti-CEA/Ag-MOF/GO/GCE. The findings indicated that, using an LOD of 0.005 ng mL−1, the reduction in peak current displayed a negative correlation with the logarithmic amount of CEA within the range of 10–3 to 5000 ng mL−1 (Fig. 1). The applicability of the developed CEA immunosensor in a human serum sample was explored. Diagnostic studies using the standard addition technique for both DPV and ELISA demonstrated good agreement in the results obtained from the two assays. The developed immunosensor provides a label-free and highly efficient approach for early cancer detection, demonstrating promise for application in human fluids and clinical settings in the future.
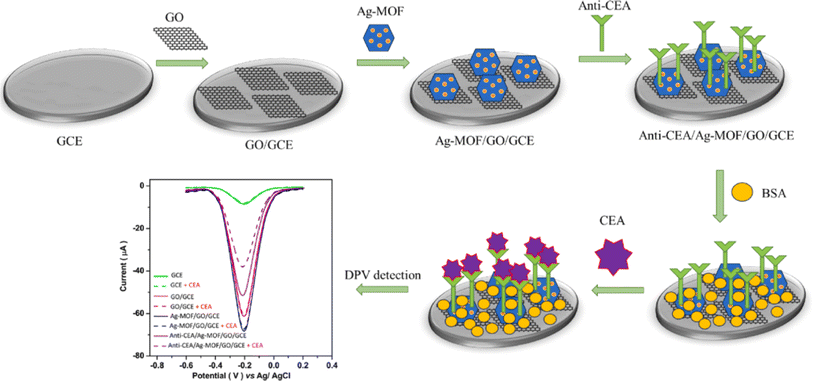 |
| Fig. 1 Diagram illustrating the procedural stages involved in the fabrication of the anti-CEA/Ag-MOF/GO/GCE biosensor and the subsequent sensing of CEA. Reproduced from ref. 35 with permission from [Elsevier], copyright [2023]. | |
In another study, researchers successfully produced a two-dimensional Cu–benzoquinone metal–organic skeleton (Cu–THQ MOF) under mild and green conditions, which is a novel kind of electroactive material with environmental friendliness and low cost.36 The incorporation of an ultra-small THQ linker with Cu2+ serves to enhance the density of redox active sites, as well as to augment both the stability and conductivity. The addition of Cu2+ resulted in an enhancement of the redox activity and stabilization of the electrical signal of THQ, leading to a synergistic amplification of the detection signal. The π–π stacking interaction between the interlayer ligand THQ and Cu contributes to the improved stability and conductivity of Cu–THQ MOF, resulting in enhanced electrochemical activity and conductivity of the material. The utilization of Cu–THQ/Au NPs in the construction of an electrochemical immunosensor for the secondary labeling not only addresses the instability of classical electrochemical immunosensor sensing signals and the susceptibility of test signals to leakage, but also augments the sensitivity of the immunosensor. The experimental outcomes demonstrated that the developed immunosensor displayed a strong linear relationship in detecting CEA within the concentration range of 1 fg mL−1 to 40 ng mL−1, with a sensitivity and an LOD of 14.81 μA (ng−1 mL−1) cm−2 and 0.477 fg mL−1, respectively. This advancement holds promise for potential applications in the detection of real serum samples in the future. Bimetallic metal–organic frameworks, composed of two different metal ions linked by organic ligands, are considered potential precursors for producing metal oxide composites via calcination. These composites display distinctive mesoporous structures, the extensive construction of heterojunction, the tailored heteroatom doping content, and large specific surface area. These characteristics contribute to improving the sensing performance beyond that of metal oxides derived from single-metal MOFs.37 For instance, it was demonstrated that the Cu MOF displayed exceptional peroxidase activity. Furthermore, the catalytic performance of a bimetallic MOF containing both Ni and Cu intercalation was found to be superior to that of the single MOF. Therefore, the bimetallic Cu–Ni MOF shows potential for enhancing signal amplification in the advancement of electrochemical immunoassay sensors. In this context, a screen-printed incorporated electrochemical immunosensor using a Cu–Ni MOF/CPE (carbon printed electrode) was introduced for point-of-care (POC) analysis of CEA, as clarified in Fig. 2.38 Primarily, a triple electrode configuration was achieved through the application of a carbon ink onto a polyethylene terephthalate (PET) platform using screen-printing techniques. An aminated Cu–Ni MOF was synthesized via a usual solvothermal approach and subsequently treated with glutaraldehyde (GA) to activate it. The activated MOF was then applied onto a CPE as the working electrode. The Cu–Ni metal–organic framework (MOF) exhibited significant peroxidase activity, enabling the catalytic oxidation of hydroquinone (HQ) with H2O2, thereby amplifying the peak current signal. In addition, CEA antibodies were chemically linked with the MOF. The MOF possessed a great specific surface area, enabling effective capture of the Ab. Following the creation of antibody–antigen immune complexes, the electrochemical signal reduced due to the suppressed catalytic function of the Cu–Ni MOF. Finally, a novel electrochemical immunosensor was established by integrating a PDMS-based electrolytic cell with the CPE, which facilitated the quantitative determination of CEA content in clinical serum samples with a low LOD of 0.16 pg mL−1 and a broad concentration range of 0.5 pg mL−1 to 500 ng mL−1.
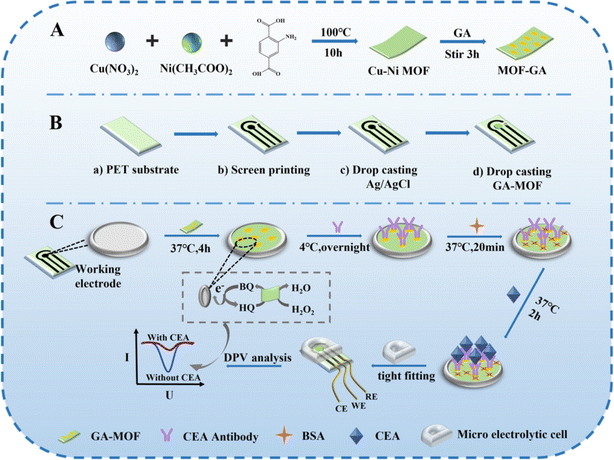 |
| Fig. 2 (A) Illustration of the synthesis procedure of the MOF-GA and Cu–Ni MOF complex. (B) Fabrication diagram of the integrated Cu–Ni MOF-based CPE. (C) Construction procedure of the CEA/BSA/Ab/MOF/CPE electrochemical immunosensor and POC testing. Reproduced from ref. 38 with permission from [Royal Society of Chemistry], copyright [2023]. | |
Thus, MOF-derived electrochemical immunosensors enhance the detection sensitivity as well as the biocompatibility of electrode materials. MOFs with abundant active sites and large surface area could realize high immobilization quantities of Abs. Meantime, the tunable pore size in MOFs enables the encapsulation and doping of biomolecules and noble metal NPs to boost the electrical conductivity of MOF-derived composites. However, MOFs have poor electrical stability and low electrical conductivity. In addition to conjugating with NPs and polymers with good electrical conductivity, enhancing the electrochemical function of MOFs is very imperative.
3.2. ECL immunosensors
In ECL as a branch of electrochemical analysis, a potential is applied on the electrode surface converting electrochemical energy into light. Owing to their high sensitivity, simple design, and low background, ECL techniques have been extensively used in different sensory fields. ECL immunoassays can efficiently avoid sample matrix luminescence and light scattering and do not require a light source. Furthermore, the feasible operation and the requirement of simple equipment make ECL immunosensors one of the advanced labeled immunoassay methods.39,40 Recently, quantum dots (QDs), luminol, Ru(bpy)32+, and other luminescent agents are used as ECL agents to label Abs or antigens improving the applicability of ECL-based methodologies. However, the realization of stable and effective fixation of luminescent agents on transducers is a challenge.41 At this state, the fixation capacity and high adsorption features of MOFs as marker carriers give high encapsulation efficiency and stability with high ECL determination sensitivity. Li et al.42 fabricated an ultrahigh-sensitive sandwich-type immunosensor endowed with these features using an N,B-doped Eu MOF (N,B-Eu MOF) as the signal amplifier and PtNP-decorated SnS2 nanoplates (Pt@SnS2) as the matrix for the selective determination of CEAs. Here, PtNPs in Pt@SnS2 enhance the capability of surface electron transport and ECL performance of SnS2 nanoplates. For synthesizing N,B-Eu MOFs, 5-nitroisophthalic acid and 5-boronoisophthalic acid were used as the dual ligands and Eu3+ as the metal node. Their dual ligand effect in N,B-Eu MOFs greatly amplified the ECL signal at the cathode. In the presence of CEA, the ECL response was weakened due to its capture by anti-CEACAM5 rabbit polyclonal Ab (Ab1). By the addition of B-Eu MOF-goat anti-rabbit IgG (Ab2), the ECL signal is enhanced because of the Ab1 and Ab2 specific recognition. Benefitting from these, the CEA was successfully quantified using an ECL sandwich-type immunosensor with an LOD of 0.06 pg mL−1. Furthermore, the proposed immunosensor showed good practicability, good reproducibility, excellent stability, and high sensitivity and specificity in real human serum.
Iridium(III) complexes represent a growing category of ECL materials known for their high quantum yield (QY), large Stokes shifts, and the ability to tune their emission wavelength across a spectrum from near-infrared to deep blue by modifying ligands.43 However, biosensing in aqueous solutions using Ir(III) complexes has been limited due to challenges such as the precipitation between proteins and organic solvents, the dispersion and poor solubility of Ir(III) complexes in aqueous solutions, and the absence of suitable biological coupling groups of Ir(III) complexes as labels for ECL signals.44 MOFs possess a crystalline porous framework that enables the binding of luminescent groups or substances onto organic ligands or metal centers. This capability allows for the effective modulation of ECL performance. However, as for 3D MOFs, the long diffusion path between co-reactants, electrons, and ions could impede the excitation of ECL emitters. Furthermore, the electrical conductivity of 3D MOFs is poor, which can decrease the ECL response. In order to address these challenges, two primary approaches can be engaged. One of them is to decrease the dimensionality of MOFs to augment their electrical conductivity. Metal–organic layers (MOLs), also known as MOF nanosheets or coordination nanosheets, not only preserve the benefits of MOFs, but also can significantly reduce the migration path of electrons and ions owing to their distinct 2D lamellar structure, further improving the application rate of ECL emitters.45 Moreover, the surface of MOLs exposes highly available active sites that can be easily post modified and functionalized.46 Another approach involves integrating MOFs with other materials to produce nanocomposites such as AuNPs, known for their favorable electrical conductivity properties. In this context, Zhang and colleagues prepared a novel and efficient ECL emitter AuNP@Ir–Zr-MOL and employed it as a signal label to develop an immunosensor for the accurate determination of CEAs.47 The 2D lamellar Zr-MOL was prepared using a solvothermal technique, followed by the coordination of Ir(III) onto the Zr-MOL structure. To enhance the electrical conductivity of Ir–Zr-MOL for potential conjugation with biological antibodies, a nanocomposite known as AuNP@Ir–Zr-MOL was fabricated through the reduction of HAuCl4 on the Ir–Zr-MOL surface using NaBH4. Ultimately, an ECL signal marker, denoted as Ab–AuNP@Ir–Zr-MOL, was developed by linking AuNP@Ir–Zr-MOL with an antibody via an Au–S bond interaction. The as-synthesized AuNP@Ir–Zr-MOL composite exhibited great ECL efficacy and could be applied as an ECL emitter because of the excellent ECL feature of Ir–Zr-MOL. The ECL intensity exhibited a linear decrease correlated with the logarithm of CEA concentration within the range of 1.00 pg mL−1 to 100 ng mL−1, with an LOD of 0.200 pg mL−1. Moreover, the immunosensor prepared in this study performed effectively in human blood samples, demonstrating its potential for practical analysis. In another study, an ECL immunosensor utilizing a “signal on-off” mechanism was developed for the sensitive detection of CEA. This approach is based on the ternary extinction effects of CuFe2O4@PDA–MB, which leads to self-enhancement of the Ru(dcbpy)32+-activated metal–organic layer [(Hf)MOL–Ru–PEI–Pd] (Fig. 3).48 The covalent attachment of PEI and Ru(dcbpy)32+ onto the (Hf)MOL surface effectively inhibits the release of Ru(dcbpy)32+ from (Hf)MOL. Reducing the distance between Ru(dcbpy)32+ and PEI can notably enhance the ECL efficiency of the system. Simultaneously, the incorporation of Pd NPs onto (Hf)MOL–Ru–PEI through Pd–NH2 can not only enhance the electron transmission capacity of (Hf)MOL–Ru–PEI, but also increase the availability of active sites for the reaction of PEI and Ru(dcbpy)32+. A highly effective quenching probe (CuFe2O4@PDA–MB) was developed, demonstrating ternary extinction effects that resulted in a significant reduction in the initial ECL signal. The UV-vis spectra of CuFe2O4@PDA–MB revealed a prominent absorption peak at 660 nm, which exhibited a clear correspondence with the ECL emission spectrum of (Hf)MOL–Ru–PEI–Pd. Consequently, the activation of the IFE (inner filter effect) resulted in the transfer of energy from the (Hf)MOL–Ru–PEI–Pd donors to the CuFe2O4@PDA–MB acceptors. The IFE arises from the radiant energy absorption by the quenching agent. In response to the CEA presence, the composite material CuFe2O4@PDA–MB–Ab2 effectively suppresses the excited states of (Hf)MOL–Ru–PEI–Pd through MB, Cu2+, and PDA by electron and energy transfer, resulting in a reduction of the ECL signal. Under optimal conditions, the proposed immunosensor displayed a broad linear response in a range of CEA concentrations of 0.1 pg mL−1 to 100 ng mL−1 with a low LOD of 20 fg mL−1.
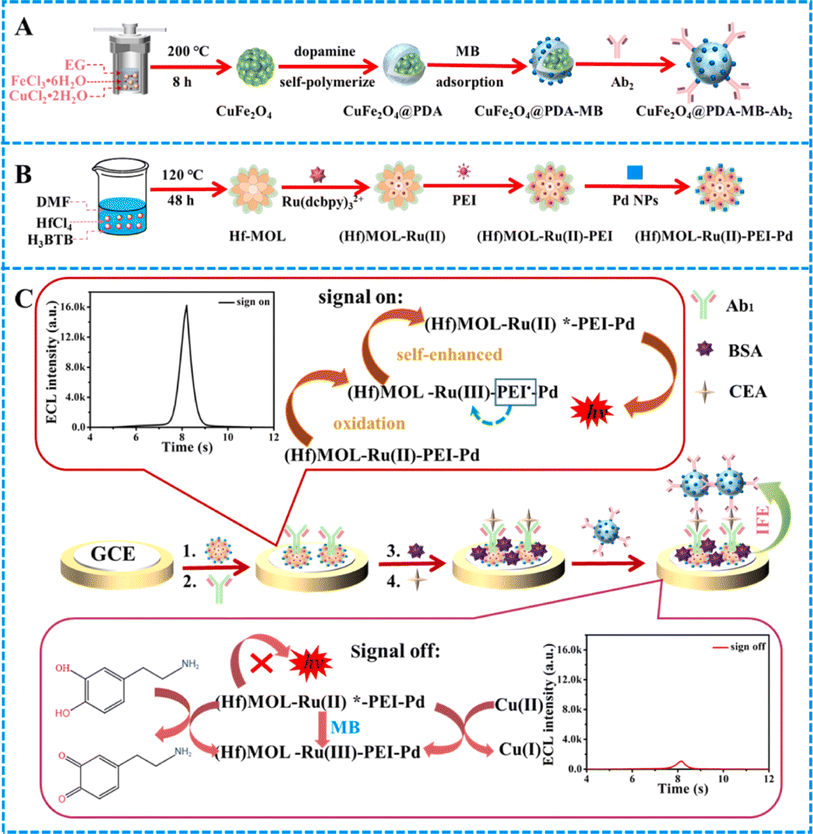 |
| Fig. 3 Diagrams illustrating (A) the sequential processes for the production of CuFe2O4@PDA–MB–Ab2, (B) the synthesis procedures for (Hf)MOL–Ru–PEI–Pd, (C) and the fabrication method for CEA sensors and potential mechanisms for luminescence. Reproduced from ref. 48 with permission from [Elsevier], copyright [2024]. | |
Inspired by the luminescence functionalities of MIL-101 MOFs, CdSe QD-modified MIL-101 nanocomposites were synthesized by Liu et al.49 for ECL detection of CEA. The as-prepared CdSe–MIL-101 nanocomposites experienced not only efficient accumulation and highly selective adsorption endowed with MOFs but also excellent sensing selectivity and high ECL intensity because of QDs. Achieving a low LOD of 0.33 fg mL−1 and a linear range from 10−12 mg mL−1 to 10−4 mg mL−1 exhibited a sensitive response of the designed ECL immunosensor toward CEAs. The high reproducibility, specificity, stability, and sensitivity of the developed immunosensor provide their potential application for the detection of real samples. Furthermore, their rational ECL mechanism related to both band-gap transition and surface states may lead to the fabrication of a designable ECL sensing platform for different analytes with great sensing selectivity and sensitivity. Moreover, in a work conducted by Lai et al.,50 an ECL immunosensor was developed based on donor–acceptor pairs for the ultrasensitive determination of CEAs. In this study, a PtCu/hollow metal polydopamine framework (h-MPF) in a ZIF-67 template as the energy acceptor and manganese-based single-atom nanozyme/polyethyleneimine luminol (Mn/PEI-luminol) in a zeolite structured MOF (ZIF-8) template as the energy donor were constructed. For the assembly of immunosensors according to Fig. 4, the Mn/PEI-luminol nanocomposite was deposited onto the surface of the glassy carbon electrode. In order to increase the electrical conductivity, AuNPs were added, providing a platform for attachment of the CEA primary Ab. After the incubation of Abs, BSA as a blocking agent was inserted. Finally, CEAs were added dropwise following the addition of PtCu/h-MPF–Ab2 composites. Mn nanozymes exhibited significant activation of H2O2 with peroxidase-like activity. By further modification of Mn nanozymes, Mn/PEI-luminol displayed outstanding ECL emission characteristics. The overlap of the UV-vis spectra of PtCu/h-MPF with ECL spectra of Mn/PEI-luminol triggers the ECL resonance energy transfer behavior between the acceptor and the donor, which improves the sensitivity of the immunosensor. The results showed an acceptable linearity of 10−5 to 80 ng mL−1, providing a new technique for the early determination of CEAs in clinical diagnosis.
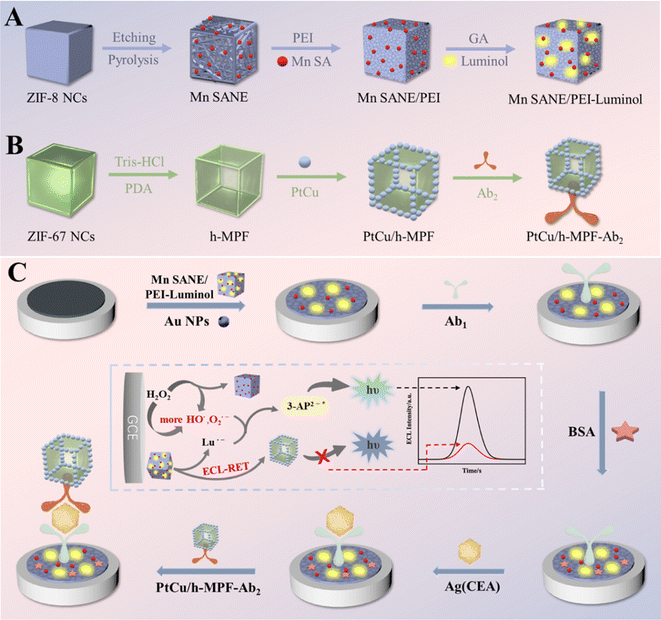 |
| Fig. 4 Schematic representation of (A) Mn/PEI-luminol, (B) PtCu/h-MPF–Ab2, and (C) the final ECL immunosensors. Reproduced from ref. 50 with permission from [American Chemical Society], copyright [2023]. | |
Benefitting from the high thermal/chemical stability and excellent degree of microporosity of ZIF-8 platforms, Huang et al.51 designed an ECL immunosensor using ZIF-8 as an ideal loading template modified with AuNP/nanoporous carbon (NPC)/graphene oxide (GO) for the determination of CEA. NPC was synthesized on GO for the in situ growth of AuNPs because of the π–π interaction between GO and ZIF-8 to form a GO@ZIF-8 composite. Here, the obtained AuNP@NPC/GO was used for immobilizing captured Abs (Ab1) owing to good biocompatibility, structural stability, good immobilization ability, and an enhanced electron transport rate. Furthermore, Ru(phen)32+-doped silica NPs (RuSiNPs) as enhanced ECL signaling units were used to label secondary Abs by a typical emulsion method. Finally, a sandwiched immunoreaction-based immunosensor was fabricated for the determination of CEAs using RuSiNPs as the signal tracer and AuNP@NPC/GO as the ECL substrate. The prepared ECL immunosensor exhibited an LOD of 0.003 ng mL−1 at a concentration of 0.01–80 ng mL −1 for CEAs in human serum samples.
MOFs were also selected to encapsulate Ru(bpy)32+ to improve water solubility, and the developed systems exhibited stable ECL emission in an aqueous solution. NH2-MIL-88(Fe) is characterized by stable octahedral building, small particle size, amino groups richness, and high porosity.52 Hence, the employment of NH2-MIL-88(Fe) as the encapsulating agent facilitates Ru(bpy)32+ to generate reproducible and stable ECL responses. Traditional MOFs are commonly employed as supports for developing ECL immunosensors, and it is challenging to achieve effective ECL responses because of the inadequate Ru(bpy)32+ loading by conventional MOFs. However, NH2-MIL-88(Fe) is characterized by a high presence of amino groups, which serve as carriers. The amino groups present on the surface serve as a catalyst for the co-reactant K2S2O8 to produce SO4˙−, leading to the accumulation of a considerable quantity of SO4˙− in the vicinity of Ru(bpy)32+. This process minimizes energy and material transfer losses between Ru(bpy)32+ and SO4˙−, consequently amplifying the ECL signal significantly.53 Additionally, the substrate could augment the stability of the illuminant. Herein, a sandwich-type ECL immunosensor was designed for the extremely sensitive determination of CEAs via a triple signal enhancement approach using Ru(bpy)32+/MIL-NH2 as the signal probe and Au@MoS2 as the co-reactant promoter (Fig. 5).54 The ECL immunosensor was effectively utilized for the specific and precise identification of CEAs, attaining an LOD of 38.9 fg mL−1.
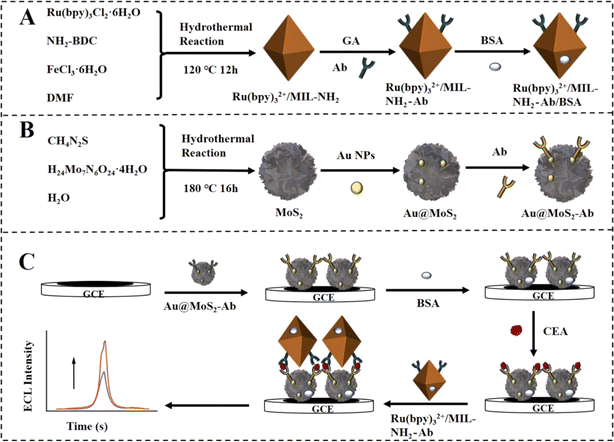 |
| Fig. 5 Synthesis of (A) Ru(bpy)32+/MIL-NH2–Ab bioconjugates and (B) Ab–Au@MoS2, as well as (C) the assembly procedure of the suggested triple improved ECL immunosensor. Reproduced from ref. 54 with permission from [Elsevier], copyright [2024]. | |
More recently, Cheng and coworkers have constructed an ECL immunosensor based on the ECL-RET approach, with Pd@UiO-66-NH2 serving as the acceptor and Ru@TiO2-MXene serving as the donor.55 The Ru@TiO2-MXene luminescent complex serves to decrease the water solubility of Ru(bpy)32+ while augmenting the conductivity of the luminescent complex, thereby boosting its ECL efficiency. The addition of small amounts of Nafion results in a notable enhancement of signal stability in luminescence, leading to consistent and high-luminescence signals even at an excitation voltage of 450 V. The Pd@UiO-66-NH2 material exhibits superior visible-light absorption capabilities and biocompatibility. Additionally, due to their small particle size and exceptional electrical conductivity, these substances not only act as receptors for Ru@TiO2-MXene, but also form direct links with a wide array of biomolecules. The developed ECL sensor demonstrates brilliant function, with a wide concentration range from 1 × 10−5 ng mL−1 to 80 ng mL−1 and a low LOD of 2.65 fg mL−1. Therefore, the fabricated ECL probe could be effectively utilized for the determination of CEAs.
The incorporation of MOFs with antibodies has enabled the utilization of ECL probes for biomedical analysis applications. To illustrate, the detection limits of these detecting strategies ranged from picograms to femtomolars for the identification of cancer and diseases. Hence, ECL immunosensors utilizing MOFs demonstrate heightened sensitivity compared to alternative immunosensor configurations using MOFs. Nevertheless, ECL immunoassays using MOFs still suffer from restricted applicability due to procedural complexity and relatively high costs. In terms of portability, the use of smart gadgets such as microfluidic and smartphone systems can be beneficial for the application of ECL immuno-biosensors.
3.3. Colorimetric immunosensors
Recently, colorimetric immunosensing catch the spotlight due to its functional simplicity and low cost. These immunosensors do not need any decoding instrumentation and typically respond by the color change in the visible range. These features endowed colorimetric immunoassays to be applied in point-of-care diagnosis and environmental mapping.56 Three types of MOF-based colorimetric immunosensors exist by the origins of color manifestation: (i) MOFs with a guest molecule, in which plasmon NPs such as platinum, silver, and gold are typically implemented in MOF-based composites. Moreover, a chromophore such as 3,3′,5,5′-tetramethylbenzidine (TMB) or o-phenylenediamine can be used.57 (ii) MOFs (intrinsic), which contain transition metal ions, where the color change is caused by a redox process, such as Fe2+/Fe3+. The color manifestation may occur by ligands' chromophore groups. (iii) MOFs, as catalysts, are most dynamically and extensively developing using non-enzymatic and enzymatic interactions. In this case, the enzyme–MOF composites could be employed as the recognition elements. It is noted that MOFs and enzymes with inherent mimic enzyme catalytic function can be used to construct colorimetric sensors with a multi-enzyme catalytic cascade. These types of immunosensors provide dual-multi-model systems to test a variety of analytes. Regarding the beneficial aspects of MOFs in catalysis owing to their flexible tailoring ability, high porosity, and tunable crystalline structure, Zeng et al.58 constructed a colorimetric immunosensor using 2D tetrakis(4-carboxyphenyl)porphyrin (Fe) (Fe-TCPP)-MOFs as enzyme mimics for the determination of CEA. In order to enhance the performance, selectivity, and sensitivity of this immunosensor, AuNPs were deposited on the surface of the MOFs to combine with DNA aptamers via Au–S bonds. To realize the colorimetric reaction, nanozyme was reacted with the substrate in the presence of CEA. The designed immunosensor displayed stability as the MOF mimic is free from interference by inhibitors or exogenous enzymes. The results showed good determination performance with an LOD of 0.742 pg mL−1 and a linear range of 1 pg mL−1 to 1000 ng mL−1. Additionally, the great applicability of the proposed immunosensor in clinical supplies provides them to be used in distinguishing the human serum of colorectal cancer patients. Remarkably, the superior advantages of MOFs such as low cost, stability, mass production, and ease of transport along with visual detection ability can make this immunosensor suitable for point-of-care testing in resource-poor or remote areas. In another work, Sha and coworkers59 developed a stable MOF-based colorimetric immunosensor as a horseradish peroxidase mimic for the determination of CEAs in serum samples. To achieve an interface assembly process and good molecular recognition, zirconium (Zr) metal ions were used as ligands. According to Fig. 6, Zr-MOF was functionalized with a DNA tetrahedron nanostructure (TDN) for the successful incorporation of a CEA aptamer for capturing targets (CEAapt-TDN-MOF colloid nanorods denoted as PCN-222 (Fe)-based MOF). By using iron tetrabenzoate porphyria, MOFs proposed strong horseradish peroxidase mimicking activity, which leads to signal amplification. Furthermore, the detection efficacy was greatly improved by TDN, which provides further accessibility toward the target. Thus, a significant colorimetric signal was exhibited when CEA was captured by its Ab. At this state, CEA was specifically detected with an LOD of 3.3 pg mL−1 in the linear range of 0.01–25 ng mL−1 in serum samples. The designed immunosensor provides a great potential for the effective clinical diagnosis and analysis of patients with colon cancer.
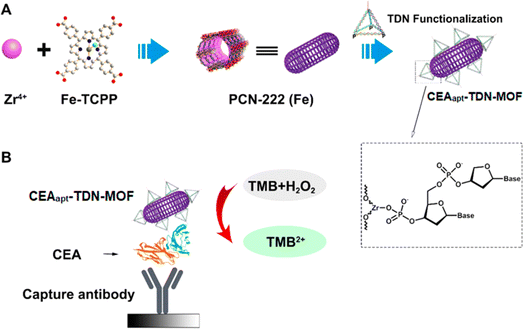 |
| Fig. 6 Schematic representation of (A) CEAapt-TDN-MOF preparation process and (B) CEA detection using the CEAapt-TDN-MOF (TMB was used as the substrate). Reproduced from ref. 59 with permission from [MDPI], copyright [2021]. | |
To illustrate, optical immunosensors utilizing MOFs have the potential to be employed in the detection of cancers and diseases, offering a detection limit ranging from nanograms to picograms. Besides, the majority of advanced immunosensors have been operated according to the colorimetric technique, offering cost-effective and simple identifying tactics. However, by examining the benefits of colorimetric immunosensors using MOFs, their number is less than that of other techniques. There is a significant shortage in the utilization of colorimetric MOF-based immunosensors for detecting CEA biomarkers.
3.4. Photoelectrochemical
The photoelectrochemistry (PEC) immunoassay represents an innovative detection platform that involves the detection of photocurrent generated by photoactive nanomaterials through the specific recognition of Ab and Ag. As a developing technology in recent years, the emerging technology of PEC immunoassay offers numerous appealing benefits for detecting CEA, including high sensitivity, perfect selectivity, user-friendly nature, and low cost. As stated by electrochemical immunosensing, optical signals in PEC are obtained through photoactive material activation using light as the excitation source and then converted into electrical signals. In photoelectric active substances, electron excitation by light energy generates charge transfer and separation, which could be used as detection signals. Although numerous PEC immunosensors have been reported, the development of novel photoelectric active materials with super detection mode and photoelectric conversion efficacy is of great significance. In this regard, the metal oxide clusters of MOFs as photoactive materials can efficiently promote the separation and transfer of electrons followed by improving the visible-light harvesting proficiency by matching well with the bands of sensitizers such as metal hybrids.60 For the development of high-sensitivity PEC immunosensors and avoiding some shortcomings of pristine MOFs, MOF-based composites could offer a hopeful perspective by providing novel points.61 Transition-metal sulfide (MoS2 and ZnS)-functionalized MOFs are such nanocomposites that offer excellent photoactive materials. Inspired by their beneficial aspects, Wei et al.62 constructed an effective sandwich-type PEC immunosensor based on MoS2/C/ZnS-MOF nanocomposites for the determination of CEA. In this study, the solvothermal-synthesized Zn-MOF was used as a template for the synthesis of MoS2/C/ZnS nanocomposites as the porous photoactive element. The formation of MoS2 and ZnS and the carbonization of Zn-MOF enhance the photocurrent response under visible light illumination. To evaluate the PEC performances of MoS2/C/ZnS, Ab1 were immobilized on the functionalized electrode followed by immersion in an alkaline phosphatase-AuNP-labeled CEA Abs (ALP-Au-Ab2) solution. After optimization, the PEC immunosensor displayed outstanding analytical features with an LOD of 1.30 pg mL−1 and a linear range of 2.0 pg mL−1 to 10.0 ng mL−1 for the determination of CEAs in serum samples.
Furthermore, Li and coworkers63 designed an ionic liquid-functionalized MOF for the in situ synthesis of AuNPs for fabricating a near-infrared light-driven PEC immunosensor for CEAs in clinical serum samples. At this state, AuNPs were integrated on the surface of the Yb-MOF to improve the photoelectric conversion efficacy. Compared to the pristine Yb-MOF, the near-infrared-PEC response of the Yb-MOF@AuNPs was enhanced four-fold. After specific assembly of anti-CEA Abs, CEA interacted with the surface of Yb-MOF@Au-NP, blocking the mass transfer of ascorbic acid and photogenerated electron–hole pair transfer to the sensing interface. Thus, the decrease in photocurrent response was used for the quantitative determination of CEAs. The PEC immunosensor exhibited a low LOD of 0.25 pg mL−1 in the linear range of 0.005–15 ng mL−1 for CEAs. These results were very reliable with the values attained from clinical tests, which verifies its applicability in clinical diagnosis.
MOFs with a wider band gap resulted in a lower concentration of photogenerated carriers. The study demonstrated that metal oxide semiconductors, QDs, and hybrid composites have the potential to enhance the transmission of photogenerated electrons and increase PEC signals as a result of their narrow band gaps. Hence, conjugating MOFs and CdTe-QDs modified with active amino and carboxyl groups, which can be covalently interacted with the Ab of CEAs, is a potential solution to enhance the technique sensitivity. The capture of CEA by its corresponding antibody, which has been modified with photogenerated carriers through steric hindrance, can result in a reduction in photocurrent. Herein, a hybrid material consisting of CdTe-QDs and MIL-101(Cr) was formulated, produced, and subsequently linked to the Ab of CEA.64 Organic–inorganic nanocomposites have revealed strong photosensitivity, high charge mobility, and effective band gap matching ability, leading to increased efficiency in separating photogenerated carriers. As the photocurrent intensity of MIL-101(Cr) varies with varying applied working voltage, a framework known as the “anodic photocurrent-CEA concentration-cathode photocurrent” system was developed. The incorporation of composite CdTe-QDs has been shown to enhance the density of photogenerating carriers. Consequently, a linear regression model was developed based on the dual photocurrent intensity of M&C composites and the amount of CEA measured. The PEC probe established in this study can detect both cathodic and anodic photocurrent signals at the same CEA concentrations. Under the optimal condition, the cathodic and anodic LOD values of the PEC immunosensor for CEA were 0.00023 ng mL−1 and 0.00018 ng mL−1, respectively. The designed PEC immunosensor presented suitable sensitivity and stability for the analysis of urine and human serum samples. For future outlook, using 3D-printing and PEC technology, a small PEC detector was deliberate with the potential to identify tumor markers. For given better vision and performance comparison, Table 1 summarizes all the mentioned MOF–Ab-based immunosensors.
Table 1 Classification and summary of MOF–Ab-based immunosensors
MOF |
Technique/detection method |
LOD (ng mL−1) |
Linearity (ng mL−1) |
Ref. |
Sm–TMA MOF |
Electrochemical |
0.001 U mL−1 |
0.01–100 U mL−1 |
29 |
Sm–TBPy MOF |
0.05 |
0.1–30 |
Sm–TCPP MOF |
0.01 |
0.1–37 |
AuNPs@Cu-MOF and AuNPs@Co-MOF |
Electrochemical |
0.31 × 10−3 |
0.001–80 |
30 |
Cu–THQ MOF/AuNPs |
Electrochemical |
0.477 × 10−6 |
10 −6 to 40 |
31 |
Cu-MOFs–TB/PDA–Ab2@CEA@Ab1–MWCNTs/GCE |
Electrochemical |
3.0 × 10−6 |
20 × 10 −6 to 200 |
34 |
MoS2/C/ZnS (Ab1)–Zn-MOF |
PEC |
1.30 × 10−3 |
2.0 × 10−3 to 10.0 |
62 |
Yb-MOF@AuNPs |
PEC |
0.25 × 10−3 |
0.005–15 |
63 |
N,B-Eu MOFs |
ECL |
0.06 × 10−3 |
— |
42 |
PtCu/h-MPF@ZIF-67 Mn/PEI-luminol@ZIF-8 |
ECL |
1.35 × 10−6 |
10−5 to 80 |
50 |
AuNPs@NPC/GO–Ab1 |
ECL |
0.003 |
0.01–80 |
51 |
RuSiNPs |
MIL-101–CdSe |
ECL |
0.33 × 10−6 |
10−9 to 10−1 |
49 |
Cu–TCCP MOF |
Colorimetry |
0.742 × 10−3 |
1 × 10−3 to 1000 |
58 |
CEAapt-TDN-MOF |
Colorimetry |
3.3 × 10−3 |
0.01–25 |
59 |
4. Conclusion and perspective
Paying continuous attention to various biomarkers in the human body facilitates the development of relevant diagnostic strategies for their efficient identification. As one of the common tumor markers, CEAs possess an imperative clinical value in early screening, the therapeutic assessment of malignant tumors, disease monitoring, and differential diagnosis. In the past few decades, the immunosensing strategies using MOF-derived nanocomposites have the properties of rapid detection, high sensitivity, and high selectivity and are extensively employed for CEA monitoring. Thereinto, current techniques for the synthesis of MOFs and MOF-derived nanocomposites and their employment as detecting platforms in the immunosensor's structure for the determination of CEAs were comprehensively outlined.
Even though MOF-based nanomaterials have shown enormous potential for biomarker diagnosis, the performance of MOF-based immunosensors needs to be enhanced with regard to stability and sensitivity. Since the building of MOFs is of great importance for sensor function, accurately controlling the size and shape of MOFs remains a challenge. To design an immunosensor probe with good sensitivity and accuracy, emerging nanoscale MOFs with uniform structures to enhance detecting active areas and trigger electron/mass transmission will be an effective approach. The accurate and efficient immobilization of antibodies/antigens on the precise location of MOFs is of great significance in the reproducibility of immunosensors. Therefore, the antibody/antigen ratio per MOF, functionalized approaches, and decorated elements have to be carefully selected. In addition, MOF-derived platforms are challenging to work, normally not portable, and not suitable for in situ and real-world application. The rational use of self-assembly techniques is essential for the specific immobilization of antibodies. Conventional methods such as covalent linkage and surface adsorption for antibody immobilization may not guarantee antibody functionality, adequate loading, and precise targeting of immobilization. In order to address this issue, enhancing the targeting efficacy of MOF immunoprobes can be achieved through the deliberate modification and assembly of the Fc region of antibodies with MOFs. This process facilitates the exposure of Fab fragments, thereby promoting interactions between antibodies and antigens. The synthesis of MOFs with a single material can be difficult when aiming for high-performance immunoassays. Therefore, combining multiple materials with distinct nanoscale characteristics to create multifunctional composite probes is crucial for improving sensing capabilities. In favor of portability, in recent years, there has been a growing trend towards incorporating analytical techniques into smartphones and 3D printed microfluidic technology, which holds promise for the advancement of biosensing technologies. Although there are currently only a few microfluidic systems and smartphone-based immunosensors utilizing MOFs in the probe structure, it is anticipated that the prevalence of these sensing platforms will grow in the future. Additionally, some affordable detecting platforms, such as paper-based immunosensors, can facilitate more available diagnostic techniques. Nowadays, multiplex bioassays are mostly used to investigate multiple biomarkers thanks to their efficiency and cost-effectiveness. Easily functionalized MOFs could simultaneously interact with different recognition groups, enabling multitarget immunoassay strategies.
Considered all these, it is important to point out that the immunosensors using MOF-derived composites are relatively immature and their progress is frequently confirmed in a laboratory context. Specifically, ECL, electrochemical, and optical immunoassays that take advantage of MOFs should be established for real samples with minimal user involvement. Therefore, upcoming studies should focus on the construction of immunosensors and data transmission while maintaining efficiency and portability.
Conflicts of interest
The authors declare no conflict of interest.
Acknowledgements
This work was supported by Research Affairs of Tabriz University of Medical Sciences, under grant number 67947.
References
- Z. Liu, Y. Wang, Y. Guo and C. Dong, Label-free electrochemical aptasensor for carcino-embryonic antigen based on ternary nanocomposite of gold nanoparticles, hemin and graphene, Electroanalysis, 2016, 28, 1023 CrossRef CAS
. - Z. Liu, Q. Rong, Z. Ma and H. Han, One-step synthesis of redox-active polymer/AU nanocomposites for electrochemical immunoassay of multiplexed tumor markers, Biosens. Bioelectron., 2015, 65, 307 CrossRef CAS PubMed
. - M. Sakari, A. Laisi and A. T. Pulliainen, Exotoxin-Targeted Drug Modalities as Antibiotic Alternatives, ACS Infect. Dis., 2022, 8, 433 CrossRef CAS PubMed
. - L. Zhang, Y. Li, Y. Ying and Y. Fu, Recent advances in fabrication strategies and protein preservation application of protein-nanomaterial hybrids: Integration and synergy, TrAC, Trends Anal. Chem., 2019, 118, 434 CrossRef CAS
. - A. Butreddy, K. Y. Janga, S. Ajjarapu, S. Sarabu and N. Dudhipala, Instability of therapeutic proteins—An overview of stresses, stabilization mechanisms and analytical techniques involved in Lyophilized proteins, Int. J. Biol. Macromol., 2021, 167, 309 CrossRef CAS PubMed
. - R. Rajan, S. Ahmed, N. Sharma, N. Kumar, A. Debas and K. Matsumura, Review of the current state of protein aggregation inhibition from a materials chemistry perspective: Special focus on polymeric materials, Mater. Adv., 2021, 2, 1139 RSC
. - T. R. Holford, F. Davis and S. P. Higson, Recent trends in antibody based sensors, Biosens. Bioelectron., 2012, 34, 12 CrossRef CAS PubMed
. - W. Liang, H. Xu, F. Carraro, N. K. Maddigan, Q. Li, S. G. Bell, D. M. Huang, A. Tarzia, M. B. Solomon and H. Amenitsch, Enhanced activity of enzymes encapsulated in hydrophilic metal–organic frameworks, J. Am. Chem. Soc., 2019, 141, 2348 CrossRef CAS PubMed
. - Q. Qiu, H. Chen, Y. Wang and Y. Ying, Recent advances in the rational synthesis and sensing applications of metal-organic framework biocomposites, Coord. Chem. Rev., 2019, 387, 60 CrossRef CAS
. - R. J. Drout, L. Robison and O. K. Farha, Catalytic applications of enzymes encapsulated in metal–organic frameworks, Coord. Chem. Rev., 2019, 381, 151 CrossRef CAS
. - C. He, D. Liu and W. Lin, Nanomedicine applications of hybrid nanomaterials built from metal–ligand coordination bonds: nanoscale metal–organic frameworks and nanoscale coordination polymers, Chem. Rev., 2015, 115, 11079 CrossRef CAS PubMed
. - Z. Wang, P. Dong, Z. Sun, C. Sun, H. Bu, J. Han, S. Chen and G. Xie, NH 2-Ni-MOF electrocatalysts with tunable size/morphology for ultrasensitive C-reactive protein detection via an aptamer binding induced DNA walker–antibody sandwich assay, J. Mater. Chem. B, 2018, 6, 2426 RSC
. - G. Chen, X. Kou, S. Huang, L. Tong, Y. Shen, W. Zhu, F. Zhu and G. Ouyang, Modulating the biofunctionality of metal–organic-framework-encapsulated enzymes through controllable embedding patterns, Angew. Chem., Int. Ed., 2020, 59, 2867 CrossRef CAS PubMed
. - M. Chen, N. Gan, Y. Zhou, T. Li, Q. Xu, Y. Cao and Y. Chen, A novel aptamer-metal ions-nanoscale MOF based electrochemical biocodes for multiple antibiotics detection and signal amplification, Sens. Actuators, B, 2017, 242, 1201 CrossRef CAS
. - C. Wang, X. Liu, N. K. Demir, J. P. Chen and K. Li, Applications of water stable metal–organic frameworks, Chem. Soc. Rev., 2016, 45, 5107 RSC
. - N. ul Qadir, S. A. Said and H. M. Bahaidarah, Structural stability of metal organic frameworks in aqueous media–controlling factors and methods to improve hydrostability and hydrothermal cyclic stability, Microporous Mesoporous Mater., 2015, 201, 61 CrossRef
. - J.-P. Zhang, Y.-B. Zhang, J.-B. Lin and X.-M. Chen, Metal azolate frameworks:
from crystal engineering to functional materials, Chem. Rev., 2012, 112, 1001 CrossRef CAS PubMed
. - H.-S. Wang, Metal–organic frameworks for biosensing and bioimaging applications, Coord. Chem. Rev., 2017, 349, 139 CrossRef CAS
. - C.-S. Liu, C.-X. Sun, J.-Y. Tian, Z.-W. Wang, H.-F. Ji, Y.-P. Song, S. Zhang, Z.-H. Zhang, L.-H. He and M. Du, Highly stable aluminum-based metal-organic frameworks as biosensing platforms for assessment of food safety, Biosens. Bioelectron., 2017, 91, 804 CrossRef CAS PubMed
. - J. S. Kahn, L. Freage, N. Enkin, M. A. A. Garcia and I. Willner, Stimuli-Responsive DNA-Functionalized Metal–Organic Frameworks (MOFs), Adv. Mater., 2017, 29, 1602782 CrossRef PubMed
. - S. K. Bhardwaj, N. Bhardwaj, G. C. Mohanta, P. Kumar, A. L. Sharma, K.-H. Kim and A. Deep, Immunosensing of atrazine with antibody-functionalized Cu-MOF conducting thin films, ACS Appl. Mater. Interfaces, 2015, 7, 26124 CrossRef CAS PubMed
. - D. Feng, T.-F. Liu, J. Su, M. Bosch, Z. Wei, W. Wan, D. Yuan, Y.-P. Chen, X. Wang and K. Wang, Stable metal-organic frameworks containing single-molecule traps for enzyme encapsulation, Nat. Commun., 2015, 6, 1 Search PubMed
. - D. Aulakh, J. B. Pyser, X. Zhang, A. A. Yakovenko, K. R. Dunbar and M. Wriedt, Metal–organic frameworks as platforms for the controlled nanostructuring of single-molecule magnets, J. Am. Chem. Soc., 2015, 137, 9254 CrossRef CAS PubMed
. - S. Sam, L. Touahir, J. Salvador Andresa, P. Allongue, J.-N. Chazalviel, A. Gouget-Laemmel, C. Henry de Villeneuve, A. Moraillon, F. Ozanam and N. Gabouze, Semiquantitative study of the EDC/NHS activation of acid terminal groups at modified porous silicon surfaces, Langmuir, 2010, 26, 809 CrossRef CAS PubMed
. - P. Kumar, A. Deep and K.-H. Kim, Metal organic frameworks for sensing applications, TrAC, Trends Anal. Chem., 2015, 73, 39 CrossRef CAS
. - K. Lu, T. Aung, N. Guo, R. Weichselbaum and W. Lin, Nanoscale metal–organic frameworks for therapeutic, imaging, and sensing applications, Adv. Mater., 2018, 30, 1707634 CrossRef PubMed
. - J. Bedia, V. Muelas-Ramos, M. Peñas-Garzón, A. Gómez-Avilés, J. J. Rodríguez and C. Belver, A review on the synthesis and characterization of metal organic frameworks for photocatalytic water purification, Catalysts, 2019, 9, 52 CrossRef
. - C.-S. Liu, J. Li and H. Pang, Metal-organic framework-based materials as an emerging platform for advanced electrochemical sensing, Coord. Chem. Rev., 2020, 410, 213222 CrossRef CAS
. - S. Biswas, Q. Lan, C. Li and X.-H. Xia, Morphologically flex Sm-MOF based electrochemical immunosensor for ultrasensitive detection of a colon cancer biomarker, Anal. Chem., 2022, 94, 3013 CrossRef CAS PubMed
. - X. Huang, X. Deng, Z. Deng, C. Huang, X. Zhu and L. Sun, Simultaneous detection of two tumor markers using electrochemical immunosensor based on ultrathin metal–organic framework derived nanosheets as redox probes, New J. Chem., 2023, 47, 11651 RSC
. - M. Jiang, M. Zhang, X. Qiao and C. Hong, Electrochemical immunosensor based on Cu(II)-tetrahydroxy-1,4-benzoquinone amplifier for carcinoembryonic antigen determination, Microchim. Acta, 2022, 189, 441 CrossRef CAS PubMed
. - E. Arkan, R. Saber, Z. Karimi and M. Shamsipur, A novel antibody–antigen based impedimetric immunosensor for low level detection of HER2 in serum samples of breast cancer patients via modification of a gold nanoparticles decorated multiwall carbon nanotube-ionic liquid electrode, Anal. Chim. Acta, 2015, 874, 66 CrossRef CAS PubMed
. - Y. Wang, H. Ma, X. Wang, X. Pang, D. Wu, B. Du and Q. Wei, Novel signal amplification strategy for ultrasensitive sandwich-type electrochemical immunosensor employing Pd–Fe3O4-GS as the matrix and SiO2 as the label, Biosens. Bioelectron., 2015, 74, 59 CrossRef CAS PubMed
. - J. Liu, Y. Shang, J. Xu, Y. Chen, Y. Jia and J. Zheng, A novel electrochemical immunosensor for carcinoembryonic antigen based on Cu-MOFs-TB/polydopamine nanocarrier, J. Electroanal. Chem., 2020, 877, 114563 CrossRef CAS
. - P. Huang, L. Meng, J. Pang, H. Huang, J. Ma, L. He and P. Amani, Development of a high-performance label-free electrochemical immunosensor for early cancer diagnosis using anti-CEA/Ag-MOF/GO/GCE nanocomposite, Environ. Res., 2023, 238, 117178 CrossRef CAS PubMed
. - M. Jiang, M. Zhang, X. Qiao and C. Hong, Electrochemical immunosensor based on Cu (II)-tetrahydroxy-1, 4-benzoquinone amplifier for carcinoembryonic antigen determination, Microchim. Acta, 2022, 189, 441 CrossRef CAS PubMed
. - L. Kong, Z. Yuan, H. Gao and F. Meng, Recent progress of gas sensors based on metal oxide composites derived from bimetallic metal-organic frameworks, TrAC, Trends Anal. Chem., 2023, 166, 117199 CrossRef CAS
. - Y. Shu, L. Yan, M. Ye, L. Chen, Q. Xu and X. Hu, A bimetallic metal–organic framework with high enzyme-mimicking activity for an integrated electrochemical immunoassay of carcinoembryonic antigen, Analyst, 2023, 148, 4721 RSC
. - B. Mohan, S. Kumar, V. Kumar, T. Jiao, H. K. Sharma and Q. Chen, Electrochemiluminescence metal-organic frameworks biosensing materials for detecting cancer biomarkers, TrAC, Trends Anal. Chem., 2022, 116735 CrossRef CAS
. - J. Zhou, Y. Li, W. Wang, X. Tan, Z. Lu and H. Han, Metal-organic frameworks-based sensitive electrochemiluminescence biosensing, Biosens. Bioelectron., 2020, 164, 112332 CrossRef CAS PubMed
. - B. Babamiri, D. Bahari and A. Salimi, Highly sensitive bioaffinity electrochemiluminescence sensors: recent advances and future directions, Biosens. Bioelectron., 2019, 142, 111530 CrossRef CAS PubMed
. - J. Li, H. Yang, R. Cai and W. Tan, Ultrahighly Sensitive Sandwich-Type Electrochemical Immunosensor for Selective Detection of Tumor Biomarkers, ACS Appl. Mater. Interfaces, 2022, 14, 44222 CrossRef CAS PubMed
. - M. A. Haghighatbin, S. E. Laird and C. F. Hogan, Electrochemiluminescence of cyclometalated iridium (III) complexes, Curr. Opin. Electrochem., 2018, 7, 216 CrossRef CAS
. - B. Tong, P. Ma, Q. Mei and Z. Hua, Synthesis, photophysical properties and electrochemiluminescence performances of a series of cationic iridium (III) complexes, Inorg. Chim. Acta, 2014, 421, 405 CrossRef CAS
. - H. Zhong, M. Wang, G. Chen, R. Dong and X. Feng, Two-dimensional conjugated metal–organic frameworks for electrocatalysis: opportunities and challenges, ACS Nano, 2022, 16, 1759 CrossRef CAS PubMed
. - L. Cao, Z. Lin, W. Shi, Z. Wang, C. Zhang, X. Hu, C. Wang and W. Lin, Exciton migration and amplified quenching on two-dimensional metal–organic layers, J. Am. Chem. Soc., 2017, 139, 7020 CrossRef CAS PubMed
. - W. Zhang, L. Chen, K. Yang, L. Wang, B. Han, S. Sun and J. Wen, An electrochemiluminescence immunosensor based on functionalized metal organic layers as emitters for sensitive detection of carcinoembryonic antigen, Sens. Actuators, B, 2023, 393, 134317 CrossRef CAS
. - X. Ren, Z. Xie, H. Wang, L. Wang, Z. Gao, H. Ma, N. Zhang, D. Fan, Q. Wei and H. Ju, Ternary electrochemiluminescence quenching effects of CuFe2O4@PDA-MB towards self-enhanced Ru(dcbpy)32+ functionalized 2D metal-organic layer and application in carcinoembryonic antigen immunosensing, Anal. Chim. Acta, 2024, 1287, 342091 CrossRef CAS PubMed
. - Q. Liu, Y. Yang, X.-P. Liu, Y.-P. Wei, C.-J. Mao, J.-S. Chen, H.-L. Niu, J.-M. Song, S.-Y. Zhang and B.-K. Jin, A facile in situ synthesis of MIL-101-CdSe nanocomposites for ultrasensitive electrochemiluminescence detection of carcinoembryonic antigen, Sens. Actuators, B, 2017, 242, 1073 CrossRef CAS
. - W. Lai, J. Li, M. Jiang, P. Li, M. Wang, C. Ma, C. Zhao, Y. Qi and C. Hong, Electrochemiluminescence Immunosensors Based on ECL-RET Triggering between Mn SANE/PEI-Luminol and PtCu/h-MPF for Ultrasensitive Detection of CEA, Anal. Chem., 2023, 95, 7109 CrossRef CAS PubMed
. - X. Huang, X. Deng, W. Qi and D. Wu, A metal–organic framework nanomaterial as an ideal loading platform for ultrasensitive electrochemiluminescence immunoassays, New J. Chem., 2018, 42, 13558 RSC
. - N. Hassan, A. Shahat, I. El-Deen, M. El-Afify and M. El-Bindary, Synthesis and characterization of NH2-MIL-88 (Fe) for efficient adsorption of dyes, J. Mol. Struct., 2022, 1258, 132662 CrossRef CAS
. - C. Li, Y. Li, Y. Zhang, G. Zhao, Y. Wang, H. Wang, H. Wang, R. Xu and Q. Wei, Signal-enhanced electrochemiluminescence strategy using iron-based metal-organic frameworks modified with carboxylated Ru (II) complexes for neuron-specific enolase detection, Biosens. Bioelectron., 2022, 215, 114605 CrossRef CAS PubMed
. - D. Zhang, M. Gao, X. Xue, X. Ren, R. Feng, D. Wu, X. Liu and Q. Wei, Triple signal-enhanced electrochemiluminescence strategy using iron-based metal-organic frameworks modified with Ru(II) complexes for carcino-embryonic antigen detection, Talanta, 2024, 267, 125239 CrossRef CAS PubMed
. - Y. Cheng, Y. Li, H. Ren, B. Wen, W. Liang, S. Zhang, B. Cong, M. Jiang and C. Hong, Electrochemiluminescent biosensor based on ECL-RET between Ru@TiO2-MXene and Pd@UiO-66-NH2 for the detection of carcinoembryonic antigens, Sens. Actuators, B, 2024, 405, 135381 CrossRef CAS
. - T. Gong, P. Li, Q. Sui, J. Chen, J. Xu and E.-Q. Gao, A stable electron-deficient metal–organic framework for colorimetric and luminescence sensing of phenols and anilines, J. Mater. Chem. A, 2018, 6, 9236 RSC
. - Y. Xing, H. Si, D. Sun and X. Hou, Magnetic Fe3O4@ NH2-MIL-101 (Fe) nanocomposites with peroxidase-like activity for colorimetric detection of glucose, Microchem. J., 2020, 156, 104929 CrossRef CAS
. - Y. Zeng, M. Wang, Z. Sun, L. Sha, J. Yang and G. Li, Colorimetric immunosensor constructed using 2D metal–organic framework nanosheets as enzyme mimics for the detection of protein biomarkers, J. Mater. Chem. B, 2022, 10, 450 RSC
. - L. Sha, M. Zhu, F. Lin, X. Yu, L. Dong, L. Wu, R. Ding, S. Wu and J. Xu, Stable DNA Aptamer–Metal–Organic Framework as Horseradish Peroxidase Mimic for Ultra-Sensitive Detection of Carcinoembryonic Antigen in Serum, Gels, 2021, 7, 181 CrossRef CAS PubMed
. - T. Yan, T. Wu, S. Wei, H. Wang, M. Sun, L. Yan, Q. Wei and H. Ju, Photoelectrochemical competitive immunosensor for 17β-estradiol detection based on ZnIn2S4@ NH2-MIL-125 (Ti) amplified by PDA NS/Mn: ZnCdS, Biosens. Bioelectron., 2020, 148, 111739 CrossRef CAS PubMed
. - J. Qin, J. Li, H. Zeng, J. Tang and D. Tang, Recent advances in metal-organic frameworks-based photoelectrochemical and electrochemiluminescence biosensors, Analyst, 2023, 148, 2200–2213 RSC
. - Q. Wei, C. Wang, P. Li, T. Wu, N. Yang, X. Wang, Y. Wang and C. Li, ZnS/C/MoS2 Nanocomposite Derived from Metal–Organic Framework for High-Performance Photo-Electrochemical Immunosensing of Carcinoembryonic Antigen, Small, 2019, 15, 1902086 CrossRef CAS PubMed
. - H. Li, Y. Li, X. Zhang, P. Liu, M. He, C. Li and Y. Wang, Near-infrared photoactive Yb-MOF functionalized with a large conjugate ionic liquid: synthesis and application for photoelectrochemical immunosensing of carcinoma embryonic antigen, Nanoscale, 2021, 13, 9757 RSC
. - C. Mao, L. Wu, Y. Wen, X. Tang, Z. Huang and L. Zhao, Photoelectrochemical immunosensor for carcinoembryonic antigen detection-an attempt for early cancer screening, Biosens. Bioelectron., 2023, 220, 114918 CrossRef CAS PubMed
.
|
This journal is © The Royal Society of Chemistry 2024 |