DOI:
10.1039/D3RA01520C
(Paper)
RSC Adv., 2023,
13, 13463-13471
Lignocellulosic residues from bioethanol production: a novel source of biopolymers for laccase immobilization
Received
7th March 2023
, Accepted 24th April 2023
First published on 3rd May 2023
Abstract
The full utilization of the main components in the lignocellulosic biomass is the major goal from a biorefinery point of view, giving not only environmental benefits but also making the process economically viable. In this sense the solid residue obtained in bioethanol production after steam explosion pretreatment, enzymatic hydrolysis, and fermentation of the lignocellulosic biomass, was studied for further valorization. Two different residues were analyzed, one generated by the production of cellulosic ethanol from an energy crop such as switchgrass (Panicum virgatum) and the other, from wood (Eucalyptus globulus). The chemical composition of these by-products showed that they were mainly composed of lignin with a total content range from 70 to 83% (w/w) and small amounts of cellulose and hemicellulose. The present work was focused on devising a new alternative for processing these materials, based on the ability of the ionic liquids (IL) to dissolve lignocellulosic biomass. The resulting mixture of biopolymers and IL constituted the raw material for developing new insoluble biocatalysts. Active hydrogels based on fungal laccase from Dichostereum sordulentum 1488 were attained. A multifactorial analysis of the main variables involved in the immobilization process enabled a more direct approach to improving hydrogel-bound activity. These hydrogels achieved a 97% reduction in the concentration of the estrogen ethinylestradiol, an emerging contaminant of particular concern due to its endocrine activity. The novel biocatalysts based on fungal laccase entrapped on a matrix made from a by-product of second-generation bioethanol production presents great potential for performing heterogeneous catalysis offering extra value to the ethanol biorefinery.
Introduction
The continuous increase in energy consumption and dependence on fossil fuels has resulted in the accumulation of greenhouse gases and, consequently, climate change. Therefore, developing new, cleaner and renewable fuel alternatives continues to be a great challenge.1,2
Lignocellulosic biomass is an abundant, renewable, and sustainable resource to produce biofuels, and in particular to produce bioethanol from cellulose. However, the production of just bioethanol is not viable from an economic point of view. To address this challenge, significant efforts have been made to develop processes and products that utilize all or most of the components of lignocellulosic biomass in an efficient manner.3,4 Pretreatment of the lignocellulosic biomass deconstructs the lignocellulosic matrix, with the aim of making the cellulose available for the next step in the production of bioethanol. Steam explosion pretreatment is one of the options to achieve this objective. In this pretreatment, the material structure is disrupted, producing a solid fraction composed mainly of lignin and cellulose where the cellulose fibers are exposed enabling the enzymes to perform their cellulolytic activity.5 The purpose of the enzymatic hydrolysis is to release the glucose from the cellulose prior to fermentation and bioethanol production. This process leaves a residue rich in lignin, which can be used to add value to the production of bioethanol in a sustainable manner.6 Derived from the steam explosion pretreatment a liquid fraction is obtained, a mixture of pentose and hexose sugars from the hemicellulose of the lignocellulosic material, which can also be used to produce valuable compounds.7 The residue left after enzymatic hydrolysis is usually burnt to generate heat and power, wasting compounds that could be useful in some other applications.8 However, different efforts have been taking place to improve the utilization of this residue, composed mainly of lignin. In some preparations, the predominance of lignin offers a wide range of advantages, from thermal and mechanical stability to antioxidant properties and UV stabilization.9 Different approaches in the utilization of lignin have been studied, using it as a macromolecule or depolymerizing it.6
Different sources of lignocellulosic biomass can be utilized for the production of bioethanol, from agricultural waste to dedicated lignocellulosic feedstocks. Among the latter, switchgrass (Panicum virgatum) is one of the most promising feedstocks due to several competitive advantages, such as high biomass yield, wide adaptability to grow in marginal soils, and low input requirements.3,10 On the other hand, the utilization of wood such as Eucalyptus has its own advantages, for instance availability, the non-seasonal character, the plantations can be run at a relative low cost, high cellulose content and high density among others.11 In addition to its importance given by a productive or economic aspect, the use of these two starting materials has a technological concern due to the difference in the composition of its lignin which could have an effect on the immobilization outcome.12–14
Enzymes are efficient and environmentally friendly catalysts widely used in industrial biotechnological processes. They can catalyze reactions under very mild conditions with a very high degree of substrate specificity. Among the enzymes with the greatest field of applications, laccases (EC 1.10.3.2) stand out, because of their low specificity, which allows them to act on a wide range of substrates, being able to even oxidize complex compounds using mediators in the electronic transference.15–17
In particular, fungal laccases have shown the capacity to degrade endocrine disruptors, including naturally occurring estrogens (estrone; 17β-estradiol; estriol) and the synthetic estrogen 17α-ethinylestradiol (EE2).16,18–25 The effect of hormonally active substances on the environment and human beings is very complex. In humans, they can cause reduction of fertility, breast cancer, prostate cancer and in aquatic animals can lead to the feminization of males and hermaphroditism.24,26 The use of synthetic estrogens in hormonal contraceptives, potentiates these effects by breaking down more slowly than the natural hormone, staying longer in the aquatic environment.27
The industrial application of laccases is limited by their instability, the difficulty to recover the used free enzyme and the impossibility of reusing it.28,29 Enzyme immobilization is one of the most useful strategies to overcome these disadvantages and for this reason, these oxido-reductases have been immobilized by different methods on diverse matrices.16,30,31 One of the simplest immobilization techniques is the entrapment of the biocatalyst in a polymeric network, resulting in a hydrogel.29,32–34 Natural biopolymers have the advantages of being inherently biocompatible, non-toxic, biodegradable, renewable, mechanically flexible. In addition, those biopolymers derived from lignocellulosic materials have wide distribution, are inexpensive and possess hydrophilic and hydrophobic regions capable of interacting with the enzyme.31,35,36 The dissolution of lignocellulosic material is a challenge in the preparation of biopolymer-based hydrogels that can be addressed using ionic liquids (ILs).34,37,38 ILs are organic salts or eutectic mixtures of an organic and inorganic salt, stable, non-flammable and recyclable.29,39
In this work, 1-n-butyl-3-methylimidazolium chloride (BmimCl) and 1-n-butyl-3-methylimidazolium acetate (BmimAc) were used for the dissolution of the by-product generated after second-generation bioethanol production from switchgrass (Panicum virgatum) and Eucalyptus globulus. The resulting mixture of biopolymers and ILs constituted the raw material for developing a novel insoluble biocatalyst based on laccase from Dichostereum sordulentum 1488, a basidiomycete strain isolated from local Eucalyptus plantations.40 The biotechnological potential of this immobilized enzyme was studied by its application in the removal of EE2 to reduce water pollution.
Materials and methods
Chemicals
1-Butyl-3-methylimidazolium acetate (BmimAc) and 1-butyl-3-methylimidazolium chloride (BmimCl), purchased from IoLiTec Ionic Liquids Technologies GmbH Heilbronn, Germany. Ethinylestradiol and 2,6-dimethoxyphenol (DMP) were purchased from Sigma-Aldrich, St. Louis, MO, USA, and dimethylsulfoxide was obtained from Carlo Erba (Milan, Italy).
Laccase production
Laccase was produced under semi-solid-state fermentation conditions. A native strain from Dichostereum sordulentum (1488) which was isolated from Uruguayan forests (Eucalyptus) was used. It was grown in potato dextrose agar (PDA) at 28 °C for 7 days. A preculture was done in cotton-plugged Erlenmeyer flasks (250 mL) containing 100 mL of malt extract 5%, Bactopeptone 1% and Eucalyptus dunnii's bark 0.25%. It was inoculated with five agar plugs (10 mm in diameter) from PDA culture and incubated at 28 °C on a rotary shaker at 150 rpm for 7 days. A suspension (100 mg/3 mL) from this preculture was prepared by homogenization of the fungal biomass. New cotton-plugged Erlenmeyer flasks (250 mL) were prepared, each one containing 17 mL of CuSO4 1 mM, KH2PO4 2 g L−1, MgSO4·7H2O 0.5 g L−1, CaCl2·H2O 0.1 g L−1, glucose 8.5 g L−1 and peptone 12 g L−1, in citrate-phosphate buffer 0.1 M, pH 5.0, and 2.5 g of E. dunnii's bark. Each flask was inoculated with 3 mL of the previously prepared suspension and incubated at 28 °C for 15 days. The crude extract laccase was collected by adding 5 mL of sodium phosphate buffer pH 6.0 0.1 M to each flask, was partially purified, lyophilized, and kept at 4 °C.
Feedstock
Switchgrass (Panicum virgatum) used in this study was provided by the Uruguayan state-owned company ANCAP. The material was harvested at the Agricultural Experimental Station Mario Cassinoni, Department of Paysandú, Uruguay, in September 2016. Eucalyptus globulus chips were provided by the Uruguayan company Chipper. Both feedstocks were dried at 40 °C by forced convection oven until 10% moisture, and then milled to an average particle size of 1 cm. Chemical composition of the raw materials determined according to NREL's standard procedure is shown in Table 1.41
Table 1 Chemical composition of raw biomasses
Raw biomass |
Composition % (w/w) |
Lignin |
Cellulose |
Hemicellulose |
Ash (inorganic) |
Switchgrass |
31.2 ± 0.8 |
31.8 ± 0.8 |
25.0 ± 1.2 |
3.2 ± 0.3 |
Eucalyptus globulus |
26.0 ± 0.8 |
47.9 ± 1.0 |
16.0 ± 0.8 |
0.4 ± 0.1 |
Steam explosion pretreatment
Switchgrass (SWG) and Eucalyptus globulus (EUC) biomasses were pretreated in a semi-continuous pre-pilot reactor installed at the Technological Laboratory of Uruguay. The equipment (Advance Bio Systems LLC, model S1401-D2011) has an approximate capacity of 10 kg h−1 of raw biomass pretreating. The conditions of temperature (200 °C) and residence time (10 min) used for pretreatment were based on previous optimization studies.5 After steam explosion pretreatment, the biomass sludge was pressed and filtrated in order to separate the hemicellulosic liquid fraction from the solid fraction. The resulting solid material, composed mainly by cellulose and lignin, was washed three times with tap water at 60 °C in a ratio 5
:
1 (water
:
dry biomass in kg), using a portable concrete mixer for 5 minutes, and re-pressed. The pH of the third washing water was 5–6.
Saccharification
Enzymatic hydrolysis of the solid fraction was done for both biomasses. The biomass (2% w/v, 100 mL total volume) was mixed with 0.05 M sodium citrate buffer (pH 4.8) in Erlenmeyer flasks. Then, 50 FPU per g biomass of enzyme (Cellic CTec2, Sigma Aldrich code SAE0020) were added to the flasks in order to initiate the reactions. The experiments were maintained in an incubator at 50 °C and 200 rpm, for 72–96 h. At the end of the saccharification, the hydrolysis was stopped by heating at 100 °C and the samples were centrifuged (15
000 rpm, 10 min) and washed two times. The solid residue was analyzed according to the NREL Laboratory Analytical Procedure.41
Pyrolysis-gas chromatography/mass spectrometry (Py-GC/MS)
The samples of switchgrass and Eucalyptus were milled to 40–60 mesh and pelletized (ca. 1 mg) for pyrolysis. A pyrolyzer PYROJECTOR II (SGE) coupled to a Shimadzu QP 5050 GC-MS. The pyrolysis temperature was 450 °C and the products were separated in an OPTIMA 1701 column (Macherey-Nagel, 30 m × 0.25 mm i.d., 0.25 μm film thickness). The chromatography conditions were helium as carrier gas; flow 1 mL min−1; split 1
:
20; an injector temperature of 250 °C, initial temperature 45 °C for 4 minutes, an increase of 4 °C min−1 to 240 °C and then an increase of 25 °C min−1 up to 280 °C maintaining this temperature for 5 min. The transfer line between the GC and the mass spectrometer was maintained at 290 °C. The spectrometer operated in electronic impact mode at 70 eV and a temperature of 180 °C. Pyrolysis products were identified using the mass spectra libraries NIST, Wiley, and literature data.42–45
Thermal stability of laccase in the presence of ILs
Aliquots of free enzyme (1000 ± 30 EU per L) were incubated for 20 hours at different temperatures (17–70 °C) in 0.05 M pH 5.0 acetate buffer, in absence and presence of 10% of each ionic liquid. After returning to room temperature the residual activity was determined.
Active hydrogel formation
Dissolution of both solid residues was carried out in BmimAc and BmimCl. In a typical assay 1 g of each IL was placed in a 20 mL vial and heated up to 100 °C under gentle stirring with DMSO as co-solvent. Then, the lignocellulosic residue (100 mg) was added and stirred until dissolution. The mixture was cooled down to 40 °C, the lyophilized enzyme was added (30 EU), and the vial content was quickly transferred to a plastic syringe and dripped over 0.05 M pH 5.0 acetate buffer, giving pearls of hydrogel. The supernatant was separated by filtration and the hydrogel pearls were washed with the same buffer under gentle stirring conditions. Laccase activity was measured in supernatant, washes, and pearls. The amount of DMSO to be added was analyzed in the range of 188–2000 μL and 600–900 μL for BmimCl and BmimAc respectively.
Laccase activity assay
Free laccase activity was measured by using DMP 2.0 mM in 0.1 M sodium acetate buffer pH 3.8 (activity buffer) as substrate, following the reaction by measurement of the increase in absorbance at 477 nm (ε477 = 14
800 M−1 cm−1) using a UV-1800 Shimadzu Spectrophotometer. The reaction mixture was prepared with 500 μL of DMP solution and 50 μL of laccase sample, prepared in the same buffer. One enzyme unit (EU) was defined as the amount of enzyme that catalyzed the appearance of 1 μmol of product per minute at 25 °C.46
The immobilized enzyme activity was assayed by incubating active hydrogel pearls with substrate under magnetic stirring (100 rpm). Samples from the reaction mixture were withdrawn at 30 seconds intervals, and filtered by suction in less than 2 seconds, which marked the interruption of the immobilized enzyme reaction. Initial velocities were determined by immediately measuring product formation in the filtrates. After this, the filtrates were returned to the reaction mixture, keeping its volume unchanged.
To check enzyme release, once the immobilized activity was measured, the hydrogels pearls were removed, the filtrates incubated at room temperature, and variation in absorbance at 477 nm determined again.
Protein determination
The protein content was determined using the BCA assay.47 Bovine serum albumin was used as a standard. Immobilized protein was estimated as the difference between the amount of protein added to the gel and that recovered in the supernatant and washing fractions.
SEM analysis
Morphological structure of biomaterials used for hydrogel preparation and of the hydrogels beads based on biopolymers from the switchgrass residue was examined by Scanning Electron Microscopy (SEM). Freeze-dried samples were metallized with a gold film and then submitted to an acceleration voltage of 20 kV in a scanning microscope JEOL JSM-5900LV. Images were obtained at magnifications up to 14
000-fold. Samples of one sphere were analyzed.
Study of variables of the immobilization process
A four factor, two-level factorial design was used, therefore, the weight of four independent variables involved in the laccase derivate formation were analyzed by designing a reduced set of experiments leaning on the program Design Expert® version 10.0 by Stat-Ease, Inc. (Suite 480, Minneapolis, MN, USA). These assays were run for the ionic liquid BmimAc, and the lignocellulosic material from Eucalyptus. The variables tested in this experiment were temperature of active derivative formation, amount of biopolymer, enzyme charge, and DMSO (Table 2), and laccase activity measured in the hydrogel (EU) was the response chosen to analyze the result. Analysis of variance (ANOVA) was carried out to determine statistical significance of each model coefficient.
Table 2 Variables studied in factorial design for laccase immobilization
Factor |
Level |
Units |
Low (−) |
Central points (0) |
High (+) |
Enzyme charge (A) |
10 |
20 |
30 |
EU |
Biopolymers (B) |
125 |
150 |
175 |
mg |
DMSO (C) |
0.6 |
0.9 |
1.2 |
mL |
Temperature (D) |
20 |
30 |
40 |
°C |
Ethinylestradiol degradation
Degradation of EE2 was carried out with both free and immobilized laccase. The reaction started with the addition of the enzyme (20 EU per L) to a solution of EE2 (0.01 mg mL−1) in activity buffer, under shaking at 60 rpm and 25 °C. Negative controls without enzyme were performed. After 24 hours, the samples (filtered or not depending on whether they came from the treatment with free or immobilized enzyme) were loaded into previously conditioned SPE columns, CHROMABOND C18 f, 100 μm, 3 mL/500 mg (MACHEREY-NAGEL, Düren, Germany) and eluted with ethyl acetate, which was then evaporated, and samples were reconstituted in MeOH. The removed hydrogels pearls were washed with activity buffer and reused in a second EE2 degradation assay.
HPLC DAD-UV analysis
The samples extracted from SPE were analyzed by HPLC to assess whether there was a reduction in the concentration of EE2. A Shimadzu Prominence system with diode array detector operating at 280 nm and a Zorbax Eclipse XDB-C18 column (Agilent Technologies, Santa Clara, CA, USA) of 250 mm, 4.6 mm i.d., and 5 μm particle size, with a guard cartridge Ultra-C18 of 10 mm and 4 mm i.d. were used. The oven temperature was 30 °C with the flow rate of 0.8 mL min−1 and the injection volume of 40 μL. The mobile phase was 0.1% phosphoric acid in Milli-Q water (solvent A) and 0.1% phosphoric acid in acetonitrile (B), and the following gradient was used: 0–20 min (20–50% B); 20–45 min (50–80% B); 45–60 min (80% B); 60–63 min (80–20% B); 63–65 min (20% B). The quantification of ethinylestradiol degradation was performed by comparison between the EE2 peak area in reactive mixtures and the EE2 control.
Results and discussion
Chemical composition of biomaterials
The chemical composition of the residue obtained after enzymatic hydrolysis in this study was similar to other studies using other lignocellulosic biomasses.8,48 As shown in Table 3 the enzymatic hydrolysis residue is composed mainly of lignin, with small amounts of cellulose and hemicellulose and it can be seen that the analyzed materials differ in their lignin, hemicellulose and ash content. Furthermore, an important difference in the relative composition – within the lignin – of the phenylpropane units syringyl (S), guaiacyl (G), and p-hydroxyphenyl, for both enzymatic hydrolysis residues was observed (Table 4). The switchgrass lignin was constituted mainly of guaiacyl and hydroxyphenyl units, with small quantities of syringyl units, in concordance with this type of biomass and previous studies.12 In the case of the Eucalyptus residue lignin, it was composed mainly of syringyl monolignols, followed by guaiacyl units with no p-hydroxyphenyl, as expected for this biomass.13,14
Table 3 Chemical composition of lignocellulosic residues used for hydrogels formation
Biomass origin |
Composition % (w/w) |
Lignin |
Cellulose |
Hemicellulose |
Ash (inorganic) |
Switchgrass |
70.6 ± 0.3 |
8.8 ± 0.5 |
6.7 ± 0.4 |
8.0 ± 0.1 |
Eucalyptus globulus |
83.5 ± 0.3 |
8.1 ± 0.3 |
2.4 ± 0.1 |
0.4 ± 0.2 |
Table 4 Lignin composition of lignocellulosic residues by Py-GC/MS analysis
Biomass origin |
Composition of lignin % (w/w) |
Syringyl units |
Guaiacyl units |
p-Hydroxyphenyl units |
Switchgrass |
12.9 |
55.2 |
31.9 |
Eucalyptus globulus |
71.8 |
28.2 |
Not detected |
Biopolymers dissolution and active hydrogel formation
It has been reported that for the processing of wood, lignocellulose, and cellulose, imidazolium ILs have been the most commonly used.37 Since lignin is the main component of the assessed feedstocks, and the β-O-4 linkage is the more abundant in lignin structure, this bond is the target to be broken by the ILs allowing lignin solubilisation. Many research has been done on this, showing that the most important bonds formed between lignin and ILs are the H bonds.49,50 The new H bonds are energetically more favourable than those existing between the lignin monomers, so when they are formed, the structure is stretched and eventually breaks. The anion of the IL plays a central role in the lignin–IL interaction since the π–π interactions and therefore the cation effect appeared to be less significant.51,52 When water is added to the lignin–IL system, as H bonds between IL–water are stronger than the ones between IL–lignin, H bonds between the lignin monomers start to re-form and finally lignin precipitates allowing hydrogel formation.49 Imidazolium ILs exhibit acid/base properties, which play an important role in their performance. In this work we selected BmimAc and BmimCl for biopolymers dissolution, because of their capacity to dissolve wood components.53,54 Moreover, BmimAc is significantly more basic than BmimCl because the acetate anion is more basic than chloride.55 Then, the acetate anion can efficiently break hydrogen bonds and therefore allows better wood and lignin dissolution compared to chloride.52
Both solid residues obtained after steam explosion pretreatment and enzymatic hydrolysis of switchgrass (Panicum virgatum) and Eucalyptus globulus, were able to be dissolved in the assayed ILs. As it was reported DMSO addition to the mixture was necessary to accomplish this objective.56 This solvent reduced the viscosity of the ILs and supported the dissolution of wood polymers and the dissociation of the ionic liquid, increasing the concentration of the ions.57 In the initial dissolution trials, we started working with EUC under the conditions previously reported by Fort et al., using a relation BmimCl/DMSO equal to 6
:
1 (w/v).58 Then, it was necessary to add more DMSO, reaching a relation IL/DMSO 1
:
1, in order to achieve a mixture with a viscosity that allowed its drip and pearl hydrogel formation. The influence of the chemical composition of the lignocellulosic residue on the dissolution process was evident since the SWG required twice as much DMSO. Whereas it was possible to obtain hydrogel beads from switchgrass in BmimCl, this IL/biopolymer combination showed some dissolution problems and hydrogel beads could not always be obtained. In the case of BmimAc, which is liquid at room temperature unlike BmimCl, less DMSO was required, managing to solubilize the EUC and SWG with a BmimAc
:
DMSO ratio of 1.7
:
1 and 0.7
:
1, respectively. This process largely occurs through the cleavage of β-O-4 linkages, and this bond is the one that mostly appears in lignin. The quantity of β-O-4 linkages depends on the proportion of syringyl units (S), guaiacyl units (G) and p-hydroxyphenyl units (H). The methoxy groups at the 3rd and 5th position of the aromatic ring in the S unit prevent the formation of β-O-4, giving a less crosslinked lignin.59 Since lignin from EUC and SWG have different amount of S (71.8 and 12.9% w/w, respectively), this could explain that EUC required less DMSO than SWG.
The development of active hydrogels requires not only the solubilization of the waste materials, but also the analysis of laccase thermal stability in presence of IL. As it could be seen in Fig. 1, at 40 °C (temperature of hydrogels synthesis), there was no significant difference between the residual activity achieved with buffer or BmimAc and only a loss of around 10% of the residual activity was observed in the presence of BmimCl.
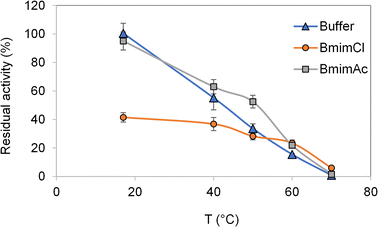 |
| Fig. 1 Thermal stability of laccase in the presence of IL (20 h incubation). | |
The use of lignocellulosic materials solubilized with ionic liquids for entrapping laccases has been little explored.60 Under the assessed conditions, more than 90% of the applied protein could be entrapped and no leakage was observed. Even though the apparent expressed activity was 5 EU per g biopolymer with around 12% of coupling efficiency, the high turnover number of laccase made it possible to have active hydrogels capable of oxidizing substrates such as DMP and EE2.61 These results are very promising, and they are the beginning for developing strategies to increase the activity linked to the hydrogel, like protection of the active site of the enzyme before the entrapping process, addition of other proteins (like BSA) that could react with the ions of the IL increasing laccase stability, etc.
Immobilization variables study
The multifactorial analysis of the main four variables participating in the immobilization process enabled a more direct approach to improving the enzyme activity in the hydrogels. Activity of the laccase derivatives was measured as detailed in the above section, and the results were analyzed with the assistance of the software as shown in Table 5.
Table 5 24 factorial design with four central points matrix and their observed responses for laccase activity
A: Enzyme (EUtotal) |
B: Biopolymers (mg) |
C: DMSO (mL) |
D: Temperature (degrees) |
Activity (EU) |
Central point data excluded for analysis. |
30 |
125 |
1.2 |
40 |
0.551 |
30 |
175 |
0.6 |
40 |
1.287 |
30 |
125 |
1.2 |
20 |
0.128 |
30 |
175 |
1.2 |
40 |
0.549 |
30 |
125 |
0.6 |
40 |
0.801 |
30 |
125 |
0.6 |
20 |
0.411 |
30 |
175 |
0.6 |
20 |
0.466 |
30 |
175 |
1.2 |
20 |
0.177 |
20 |
150 |
0.9 |
30 |
0.479 |
20 |
150 |
0.9 |
30 |
0.791a |
20 |
150 |
0.9 |
30 |
0.497 |
20 |
150 |
0.9 |
30 |
0.351 |
10 |
175 |
1.2 |
20 |
0.084 |
10 |
175 |
1.2 |
40 |
0.212 |
10 |
125 |
1.2 |
20 |
0.047 |
10 |
175 |
0.6 |
40 |
0.330 |
10 |
125 |
0.6 |
20 |
0.348 |
10 |
175 |
0.6 |
20 |
0.539 |
10 |
125 |
0.6 |
40 |
0.322 |
10 |
125 |
1.2 |
40 |
0.125 |
One of the central data points was identified as an outlier and was removed (see in Table 5), and a square root transformation was done to the data for the analysis. The significance of all the terms in the method was statistically evaluated and computed by the prob > F at a confidence level of <0.0500. The model F-value of 28.10 implies the model is significant (Table 6). The “Lack of Fit F-value” of 1.59 implies the lack of fit is not significant relative to the pure error. Enzymatic charge (A), amount of DMSO (C), temperature (D), the interaction enzyme–temperature (AD) and biopolymers (B) to a lesser extent, were significant model terms.
Table 6 ANOVA results for selected factorial model. Analysis of variance table [partial sum of squares – type III]
Source |
Sum of squares |
df |
Mean square |
F value |
p-Value prob > F |
Model |
0.80 |
5 |
0.16 |
28.10 |
<0.0001 |
A-Enzyme |
0.21 |
1 |
0.21 |
36.61 |
<0.0001 |
B-Biopolymers |
0.028 |
1 |
0.028 |
4.95 |
0.0444 |
C-DMSO |
0.31 |
1 |
0.31 |
54.94 |
<0.0001 |
D-Temperature |
0.15 |
1 |
0.15 |
25.89 |
0.0002 |
AD |
0.10 |
1 |
0.10 |
18.12 |
0.0009 |
Residual |
0.074 |
13 |
5.703 × 10−3 |
|
|
Lack of fit |
0.067 |
11 |
6.047 × 10−3 |
1.59 |
0.4496 |
Pure error |
7.629 × 10−3 |
2 |
3.814 × 10−3 |
|
|
Cor total |
0.88 |
18 |
|
|
|
The Pareto chart (Fig. 2) reveals those factors and interactions which have positive and negative effects in the activity, being A, D, AD and B in the first group, and C in the second one. The negative effect of DMSO on the response and the positive one of biopolymers are depicted in Fig. 2, and a three-dimensional response surface plot was drawn to better illustrate the effect of the enzyme charge and temperature, and the interaction between them. The negative effect of DMSO concentration on gel-bound activity could be expected, since it has been reported that for certain proteins, the increase of DMSO concentration is closely associated with the start of protein unfolding.62 This process could expose the non-polar surfaces of the proteins, in the case of laccase, its active site, driving towards a decrease in enzyme activity.63
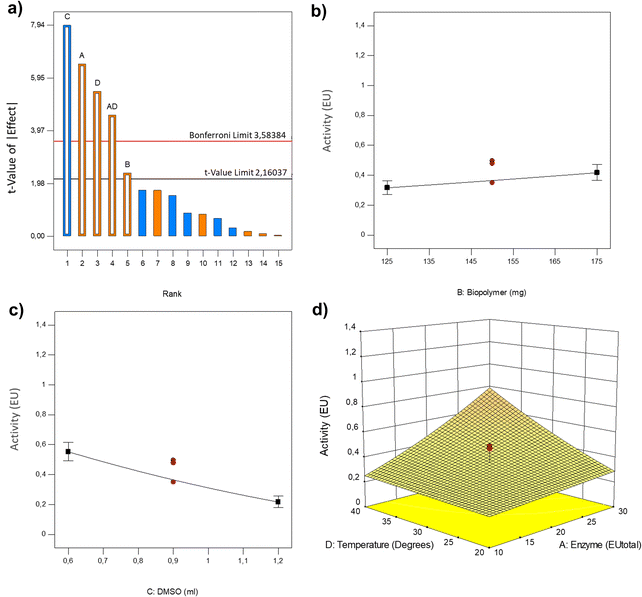 |
| Fig. 2 Factorial design analysis. (a) Pareto chart ((A) enzyme; (B) biopolymers; (C) DMSO; (D) temperature; in orange factors with positive effects; in blue negative effects); (b) effect of the factor biopolymers (mg); (c) effect of the factor DMSO (mL); (d) effect of the interaction between temperature and enzyme charge. | |
The following final equation in terms of actual factors was obtained:
Sqrt (EU) = 0.73265 − 0.012685 × enzyme + 1.68064 × 10−3 × biopolymers − 0.46647 × DMSO − 6.46441 × 10−3 × temperature + 8.03600 × 10−4 × enzyme × temperature |
As can be seen in Fig. 3, the hydrogels pearls turned out to be porous with a diameter around of 3 mm (Fig. 3d). There was a morphological change in the hydrogel based on switchgrass biomaterial with entrapped enzyme since a decrease in the porosity of the pearl surface was observed. In the magnification of the cross-section of the bead with entrapped enzyme (Fig. 3e and f) it could be seen spherical-shaped structures that could correspond to deposits or accumulations of the biocatalyst.
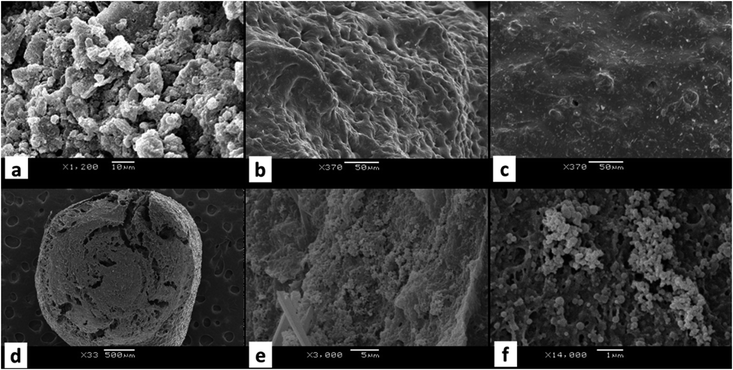 |
| Fig. 3 Scanning electron micrograph of (a) starting biomaterial used to form the hydrogel beads from the treatment of switchgrass, (b) surface of freeze-dried hydrogels bead based on switchgrass biomaterial, (c) surface of hydrogel based on switchgrass with entrapped laccase, (d) a cross-section of the bead of hydrogel with entrapped laccase, (e and f) magnification of the cross section shown in (d). | |
Ethinylestradiol degradation
The fungal laccase used in this study proved to be effective in degrading EE2, since the residual estrogen concentration in a solution treated with free enzyme (0.1 EU) was 7.5% of the initial value (10 mg L−1). Moreover, the active hydrogel beads obtained from switchgrass and Eucalyptus biopolymers also proved to be useful for the removal of the contaminant, achieving a 97% reduction in both cases.
It is important to consider the real estrogen concentrations that the immobilized enzyme would have to deal with. For instance, Auriol et al. reported a concentration of 6.2 ng L−1 in municipal wastewater, while Adeyeye and Laub found a median concentration of 5 ng L−1 EE2 in surface water receiving treated effluents discharged from wastewater treatment plants in Cibolo Creek, a stream near San Antonio (TX) and 11.4 ng L−1 of EE2 in the effluent.23,64 Likewise, in the effluent of a municipal wastewater treatment plant of Calo-Milladoiro (Ames, Spain) analyzed by Lloret et al. (2013) the estrogens were present at concentrations of 0.29–1.52 ng L−1.19 In Uruguay, Griffero et al. published a study about the Atlantic coastal lagoons, Laguna de Castillos and Laguna de Rocha, where EE2 was detected in a range of concentrations between 0.13 and 45.51 μg L−1.65
It becomes evident that active hydrogel beads obtained in this work would be capable of removing EE2 in a real sample, since they demonstrated their efficiency in reducing higher concentrations of this estrogen. This result is in agreement with the best findings reported for other laccases, either in free or immobilized form.19,21,23–25,66,67 However, it should be noted that in these works a lower concentration of EE2 was used, ranging from 0.1 to 5.0 mg L−1 in most cases. Under the conditions assayed in the present study (EE2 concentration equal to 10 mg mL−1 and 24 h of reaction time) Garcia et al. attained to remove 80% of the estrogen compound with laccase from Pycnoporus sanguineus immobilized on calcium and copper alginate–chitosan beads, while Zdarta et al. achieved only a 50% reduction in the estrogen concentration, using immobilized laccase from Trametes versicolor in PAN/PES electrospun fibers.21,68 In addition, in the present work, the removal of EE2 was performed using a very low amount of enzyme compared to that reported by other authors, which confirms the advantage of using these hydrogels over other immobilized laccases.
Finally, after one use and one reuse, the active hydrogel pearls kept unchanged their EE2 removal capacity. This result is of great significance, demonstrating the possibility of reusing the insoluble biocatalyst, one of the most important goals to be achieved by an immobilization process.
Conclusions and future work
In the present work the lignin-rich enzymatic hydrolysis residue from the production of bioethanol was used for the first time for an enzyme immobilization purpose. This material was the source of biopolymers that generate the net where the enzyme laccase was entrapped. Despite their different chemical composition, especially in terms of lignin constituents, both assayed biomasses were adequate as starting materials.
The resulting insoluble biocatalysts showed great efficiency for the elimination of the emerging contaminant ethinylestradiol. Based on this, it could be useful in the environmental area for the development of a clean technology for wastewater treatment.
Thus, this study is the basis for a sustainable procedure that also contributes to the viability of the bioethanol production process. In the future, new strategies should be investigated to improve the expressed activity in the hydrogel, like exploring immobilization methods different from entrapping or enzyme pretreatment before performing the immobilization process. Likewise, it is of great importance to verify the reduction of estrogenic activity in the treated solution as well as to determine the chemical structure of the products obtained after water treatment.
Author contributions
Valeria Vázquez: conceptualization, investigation, formal analysis, visualization, writing – original draft. Victoria Giorgi: conceptualization, investigation, formal analysis, validation, visualization, writing – review & editing. Fernando Bonfiglio: conceptualization, investigation, formal analysis, validation, visualization, writing – original draft. Pilar Menéndez: conceptualization, writing – original draft, writing – review & editing, project administration, funding acquisition. Larissa Gioia: conceptualization, methodology, formal analysis, visualization, supervision, writing – original draft, writing – review & editing. Karen Ovsejevi: conceptualization, methology, validation, supervision, writing – original draft, writing – review & editing, project administration, funding acquisition.
Conflicts of interest
There are no conflicts to declare.
Acknowledgements
This study has been supported by Agencia Nacional de Investigación e Innovación (ANII), project FCE_1_2019_1_156567, and Programa de Desarrollo de las Ciencias Básicas (PEDECIBA), Uruguay. The authors thank Dr Vittorio Vinciguerra (Department for Innovation in Biological, Agro-Food and Forest System, Univesità della Tuscia, Viterbo, Italy) for the structural analysis of lignin by pyrolysis-gas chromatography/mass spectrometry.
References
- Y. Liu, P. Cruz-Morales, A. Zargar, M. S. Belcher, B. Pang, E. Englund, Q. Dan, K. Yin and J. D. Keasling, Cell, 2021, 184, 1636–1647 CrossRef CAS PubMed.
- K. Kupryaniuk, A. Wójtowicz, J. Mazurkiewicz, T. Słowik and A. Matwijczuk, Energies, 2021, 14, 6997 CrossRef CAS.
- V. K. Gupta, A. Pandey, M. Koffas, S. I. Mussatto and S. Khare, Renewable Sustainable Energy Rev., 2022, 167, 112683 CrossRef.
- M. Broda, D. J. Yelle and K. Serwańska, Molecules, 2022, 27, 8717 CrossRef CAS PubMed.
- F. Bonfiglio, M. Cagno, F. Rey, M. Torres, S. Böthig, P. Menéndez and S. I. Mussatto, Biomass Bioenergy, 2019, 121, 41–47 CrossRef CAS.
- L. A. Zevallos Torres, A. Lorenci Woiciechowski, V. O. de Andrade Tanobe, S. G. Karp, L. C. Guimarães Lorenci, C. Faulds and C. R. Soccol, J. Cleaner Prod., 2020, 263, 121499 CrossRef CAS.
- F. Bonfiglio, M. Cagno, C. K. Yamakawa and S. I. Mussatto, Ind. Crops Prod., 2021, 170, 113800 CrossRef CAS.
- R. Xu, H. Du, H. Wang, M. Zhang, M. Wu, C. Liu, G. Yu, X. Zhang, C. Si, S. E. Choi and B. Li, Front. Bioeng. Biotechnol., 2021, 9, 1–10 Search PubMed.
- Y. Peng, S. S. Nair, H. Chen, N. Yan and J. Cao, ACS Sustainable Chem. Eng., 2018, 6, 11078–11086 CrossRef CAS.
- V. Larnaudie, M. D. Ferrari and C. Lareo, Renewable Sustainable Energy Rev., 2022, 158, 112115 CrossRef CAS.
- L. Penín, M. López, V. Santos, J. L. Alonso and J. C. Parajó, Bioresour. Technol., 2020, 311, 123528 CrossRef PubMed.
- J. Yan, Z. Hu, E. C. B. Yunqiao Pu and A. J. Ragauskas, Biomass Bioenergy, 2010, 34, 48–53 CrossRef CAS.
- C. A. Nunes, C. F. Lima, L. C. A. Barbosa, J. L. Colodette, A. F. G. Gouveia and F. O. Silvério, Bioresour. Technol., 2010, 101, 4056–4061 CrossRef CAS PubMed.
- P. Ramadevi, D. V. Hegde, M. Varghese, R. Kamalakannan, S. P. Ganapathy and D. S. Gurumurthy, J. Near Infrared Spectrosc., 2016, 24, 529–536 CrossRef CAS.
- A. Kunamneni, F. J. Plou, A. Ballesteros and M. Alcalde, Recent Pat. Biotechnol., 2008, 2, 10–24 CrossRef CAS PubMed.
- K. Sun, S. Li, Y. Si and Q. Huang, Crit. Rev. Biotechnol., 2021, 41, 969–993 CrossRef CAS PubMed.
- T. Senthivelan, J. Kanagaraj and R. C. Panda, Biotechnol. Bioprocess Eng., 2016, 21, 19–38 CrossRef CAS.
- G. Macellaro, C. Pezzella, P. Cicatiello, G. Sannia and A. Piscitelli, BioMed Res. Int., 2014, 2014, 614038 Search PubMed.
- L. Lloret, G. Eibes, M. T. Moreira, G. Feijoo and J. M. Lema, Environ. Sci. Technol., 2013, 47, 4536–4543 CrossRef CAS PubMed.
- L. Lloret, G. Eibes, G. Feijoo, M. T. Moreira and J. M. Lema, J. Hazard. Mater., 2012, 213–214, 175–183 CrossRef CAS PubMed.
- J. Zdarta, K. Jankowska, U. Strybel, Ł. Marczak, L. N. Nguyen, P. Oleskowicz-Popiel and T. Jesionowski, J. Hazard. Mater., 2022, 432, 128688 CrossRef CAS PubMed.
- S. Nicotra, A. Intra, G. Ottolina, S. Riva and B. Danieli, Tetrahedron: Asymmetry, 2004, 15, 2927–2931 CrossRef CAS.
- M. Auriol, Y. Filali-Meknassi, R. D. Tyagi and C. D. Adams, Water Res., 2007, 41, 3281–3288 CrossRef CAS PubMed.
- M. Bilal and H. M. N. Iqbal, Sci. Total Environ., 2019, 690, 447–459 CrossRef CAS PubMed.
- J. Zdarta, L. N. Nguyen, K. Jankowska, T. Jesionowski and L. D. Nghiem, Crit. Rev. Environ. Sci. Technol., 2021, 1–30 Search PubMed.
- M. Adeel, X. Song, Y. Wang, D. Francis and Y. Yang, Environ. Int., 2017, 99, 107–119 CrossRef CAS PubMed.
- D. G. J. Larsson, Philos. Trans. R. Soc., B, 2014, 369, 20130571 CrossRef PubMed.
- R. A. Sheldon, Adv. Synth. Catal., 2007, 349, 1289–1307 CrossRef CAS.
- R. A. Sheldon and J. M. Woodley, Chem. Rev., 2018, 118, 801–838 CrossRef CAS PubMed.
- L. Gioia, K. Ovsejevi, C. Manta, D. Míguez and P. Menéndez, Environ. Sci.: Water Res. Technol., 2018, 4, 2125–2135 RSC.
- N. A. Daronch, M. Kelbert, C. S. Pereira, P. H. H. de Araújo and D. de Oliveira, Chem. Eng. J., 2020, 397, 125506 CrossRef CAS.
- Y. Liu and J. Y. Chen, J. Bioact. Compat. Polym., 2016, 31, 553–567 CrossRef CAS.
- P. B. Poulsen, Biotechnol. Genet. Eng. Rev., 1984, 1, 121–140 CrossRef CAS.
- M. H. Kim, S. An, K. Won, H. J. Kim and S. H. Lee, J. Mol. Catal. B: Enzym., 2012, 75, 68–72 CrossRef CAS.
- J. Meyer, L. E. Meyer and S. Kara, Eng. Life Sci., 2022, 22, 165–177 CrossRef CAS PubMed.
- H. T. Imam, P. C. Marr and A. C. Marr, Green Chem., 2021, 23, 4980–5005 RSC.
- P. Mäki-Arvela, I. Anugwom, P. Virtanen, R. Sjöholm and J. P. Mikkola, Ind. Crops Prod., 2010, 32, 175–201 CrossRef.
- J. B. Ocreto, W. H. Chen, A. P. Rollon, H. Chyuan Ong, A. Pétrissans, M. Pétrissans and M. D. G. De Luna, Chem. Eng. J., 2022, 445, 136733 CrossRef CAS.
- H. Wang, G. Gurau and R. D. Rogers, Chem. Soc. Rev., 2012, 41, 1519–1537 RSC.
- S. Martínez and K. K. Nakasone, Check List, 2014, 10, 1237–1242 CrossRef.
- A. Sluiter, B. Hames, R. Ruiz, C. Scarlata, J. Sluiter D. Templeton and D. Crocker, NREL/TP-510-42618 analytical procedure - Determination of structural carbohydrates and lignin in Biomass, 2012 Search PubMed.
- O. Faix, D. Meier and I. Fortmann, Holz Roh- Werkst., 1990, 48, 351–354 CrossRef CAS.
- O. Faix, D. Meier and I. Fortmann, Holz Roh- Werkst., 1990, 48, 281–285 CrossRef CAS.
- O. Faix, I. Fortmann, J. Bremer and D. Meier, Holz Roh- Werkst., 1991, 49, 299–304 CrossRef CAS.
- O. Faix, I. Fortmann, J. Bremer and D. Meier, Holz Roh- Werkst., 1991, 49, 213–219 CrossRef CAS.
- S. Galai, A. P. De Los Ríos, F. J. Hernández-Fernández, S. H. Kacem and F. Tomas-Alonso, RSC Adv., 2015, 5, 16173–16189 RSC.
- P. K. Smith, R. I. Krohn, G. T. Hermanson, A. K. Mallia, F. H. Gartner, M. D. Provenzano, E. K. Fujimoto, N. M. Goeke, B. J. Olson and D. C. Klenk, Anal. Biochem., 1985, 150, 76–85 CrossRef CAS PubMed.
- L. An, C. Si, G. Wang, W. Sui and Z. Tao, Ind. Crops Prod., 2019, 128, 177–185 CrossRef CAS.
- W. Ji, Z. Ding, J. Liu, Q. Song, X. Xia, H. Gao, H. Wang and W. Gu, Energy Fuels, 2012, 26, 6393–6403 CrossRef CAS.
- E. C. Achinivu, Int. J. Mol. Sci., 2018, 19, 428 CrossRef PubMed.
- Z. Zhang, J. Song and B. Han, Chem. Rev., 2017, 117, 6834–6880 CrossRef CAS PubMed.
- V. Strehmel, D. Strunk, H. Wetzel and N. Strehmel, Sustainable Chem. Pharm., 2017, 6, 107–113 CrossRef.
- N. Muhammad, Z. Man, M. I. A. Mutalib, M. A. Bustam, C. D. Wilfred, A. S. Khan, Z. Ullah, G. Gonfa and A. Nasrullah, ChemBioEng Rev., 2015, 2, 257–278 CrossRef.
- A. V. Ladesov, A. V. Belesov, M. V. Kuznetsova, A. S. Pochtovalova, A. V. Malkov, S. L. Shestakov and D. S. Kosyakov, Russ. J. Appl. Chem., 2018, 91, 663–670 CrossRef CAS.
- C. Liu, Y. Li and Y. Hou, Int. J. Chem. Eng., 2018, 2018, 1–8 Search PubMed.
- T. G. Paiva, M. Zanatta, E. J. Cabrita, C. E. S. Bernardes and M. C. Corvo, J. Mol. Liq., 2022, 345, 117810 CrossRef CAS.
- H. Abushammala and J. Mao, Polymers, 2020, 12, 195 CrossRef CAS PubMed.
- D. A. Fort, R. C. Remsing, R. P. Swatloski, P. Moyna, G. Moyna and R. D. Rogers, Green Chem., 2007, 9, 63–69 RSC.
- N. D. Patil, N. R. Tanguy and N. Yan, in Lignin in Polymer Composites, Elsevier Inc., 2016, pp. 27–47 Search PubMed.
- M. B. Turner, S. K. Spear, J. D. Holbrey and R. D. Rogers, Biomacromolecules, 2004, 5, 1379–1384 CrossRef CAS PubMed.
- S. M. Jones and E. I. Solomon, Cell. Mol. Life Sci., 2015, 72, 869–883 CrossRef CAS PubMed.
- T. Arakawa, Y. Kita and S. N. Timasheff, Biophys. Chem., 2007, 131, 62–70 CrossRef CAS PubMed.
- R. Mehra, J. Muschiol, A. S. Meyer and K. P. Kepp, Sci. Rep., 2018, 8, 1–16 CAS.
- A. O. Adeyeye and B. G. Laub, Environ. Monit. Assess., 2020, 192, 426 CrossRef CAS PubMed.
- L. Griffero, J. Alcántara-Durán, C. Alonso, L. Rodríguez-Gallego, D. Moreno-González, J. F. García-Reyes, A. Molina-Díaz and A. Pérez-Parada, Sci. Total Environ., 2019, 697, 134058 CrossRef CAS PubMed.
- L. Lloret, G. Eibes, T. A. Lú-Chau, M. T. Moreira, G. Feijoo and J. M. Lema, Biochem. Eng. J., 2010, 51, 124–131 CrossRef CAS.
- K. Sun, D. Hong, J. Liu, A. Latif, S. Li, G. Chu, W. Qin and Y. Si, Sci. Total Environ., 2021, 782, 146917 CrossRef CAS.
- L. F. Garcia, M. F. A. R. Lacerda, D. V. Thomaz, J. C. de Souza Golveia, M. d. G. C. Pereira, E. de Souza Gil, F. Schimidt and M. F. Santiago, Prep. Biochem. Biotechnol., 2019, 49, 375–383 CrossRef CAS PubMed.
|
This journal is © The Royal Society of Chemistry 2023 |