DOI:
10.1039/C3RA46076B
(Paper)
RSC Adv., 2014,
4, 10060-10066
Aggregation-induced emission dye based luminescent silica nanoparticles: facile preparation, biocompatibility evaluation and cell imaging applications†
Received
24th October 2013
, Accepted 11th December 2013
First published on 11th December 2013
Abstract
In this work, we reported for the first time that luminescent silica nanoparticles could be easily fabricated by the encapsulation of an aggregation-induced emission dye (named An18) via a modified Stöber method. In this method, octadecyltrimethoxysilane (C18-Si) and An18 were first self assembled and served as the core of the silica nanoparticles. Then another silicate precursor, tetraethoxysilane, was further coated on the luminescent core, thus forming luminescent silica nanoparticles. The properties of the thus obtained luminescent silica nanoparticles (named An18-SiO2 NPs) were investigated by fluorescence spectroscopy, transmission electron microscopy and Fourier transform infrared spectroscopy. The biocompatibility and cell uptake behavior of the An18-SiO2 NPs were further investigated to evaluate their potential for biomedical applications. Our results demonstrated that the An18-SiO2 NPs have a uniform spherical morphology (with diameter of 70–80 nm), high water dispersibility, remarkable fluorescent properties and excellent biocompatibility, making them a promising candidate material for various biomedical applications.
1. Introduction
Since Alivisatos et al. first reported the utilization of semiconductor quantum dots as biological probes in 1998, the biomedical applications of fluorescent inorganic nanomaterials (FINs) have become one of the most dynamic research fields.1 To date, a variety of FINs including semiconductor quantum dots, fluorescent carbon nanoparticles, metal nanoclusters, lanthanide (Ln) ion doped materials and fluorescent silica nanoparticles, have been prepared and widely explored for different biomedical applications.2–12 As compared with conventional organic dyes, FINs exhibited numerous advantages for biomedical applications, which include ease of synthesis, controllable morphology and better fluorescent properties.13 More importantly, nanosized FINs provide a robust framework in which two or more components can be incorporated to give multifunctional capabilities.14 Despite the significant progress that has been made in the biomedical applications of FINs, the practical biomedical applications of FINs are limited by their intrinsic drawbacks, which include poor water dispersibility, high toxicity to living organisms, low fluorescence quantum yield and high cost.15–17 Therefore, the development of novel fluorescent nanoprobes which could overcome these drawbacks is still highly desirable.
Given their high water dispersibility, excellent biocompatibility, facile surface functionalization and cellular membrane-penetrating capacity, luminescent silica nanoparticles have emerged as one of the most promising FINs for various biomedical applications.18–27 A general strategy for the fabrication of luminescent silica nanoparticles is immobilization or encapsulation of inorganic fluorophores (such as semiconductor quantum dots) or small organic dyes (such as fluorescein or rhodamine) into silica nanoparticles.28,29 After these fluorophores are incorporated into silica nanoparticles, the thus obtained luminescent silica nanoparticles are expected to possess better photostability as the fluorophores are protected by the silica shell. However, it is still difficult to obtain luminescent silica nanoparticles with high fluorescence intensity via encapsulation of conventional organic dyes or inorganic nanoparticles, due to the notorious aggregation caused quenching (ACQ) effect. Therefore, it will inevitably restrict their performance for biomedical applications.
Significantly different from the conventional organic dyes, some organic dyes emit much stronger fluorescence in the aggregation state than in the dispersion state, which is called aggregation-induced emission (AIE).30 Since Tang et al. reported the “abnormal” phenomenon in 2001, a variety of dyes with AIE properties such as tetraphenylethene,31–34 siloles,35,36 triphenylethene,37–40 distyrylanthracene41–44 and cyano-substituted diarylethene,45–52 have been discovered and utilized for the fabrication of fluorescent nanoprobes. In recent years, Tang et al. also demonstrated that highly luminescent silica nanoparticles can be obtained via immobilization of the tetraphenylethene and silole derivatives into silica nanoparticles, via a modified Stöber method.53,54 In this procedure, the AIE dyes should be first linked with silocanes via different conjugation reactions, and then encapsulated into silica via sol–gel reactions. In Tang's work, a covalent conjugation reaction was needed to prepare the AIE dye containing silica precursors, and therefore the AIE derivatives had to bear specific functional groups, which made the procedure for the preparation of luminescent silica nanoparticles rather limited and complex. Therefore, development of a more simple and effective method for the preparation of AIE dye based luminescent silica nanoparticles is still of great research interest.
In this contribution, we report a one-pot facile encapsulation of AIE dye An18 (derivatized from 9,10-distyrylanthracene with an alkoxyl endgroup) into silica nanoparticles via the modified Stöber method. Octadecyltrimethoxysilane (C18-Si) and tetraethyl silicate (TEOS) were introduced as the collaborative sources of the silica nanoparticles. As shown in Scheme 1, An18 and C18-Si were first dispersed in THF to self-assemble by hydrophobic interaction, which could further serve as a structure directing template for the preparation of the fluorescent silica nanoparticles (An18-SiO2NPs) with TEOS and ammonia water. Such obtained fluorescent nanoparticles were investigated by fluorescent (FL) spectroscopy, transmission electron microscopy (TEM) and Fourier transform infrared (FT-IR) spectroscopy. The surface morphology and biocompatibility of the An18-SiO2 NPs, as well as their cell uptake behavior, were further investigated to evaluate their potential biomedical applications.
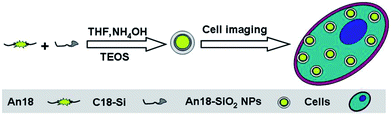 |
| Scheme 1 Schematic showing the preparation of luminescent silica nanoparticles (An18-SiO2 NPs) and their utilization for cell imaging applications. | |
2. Experimental procedure
2.1 Materials and characterization
Octadecyltrimethoxysilane purchased from Aladdin, 9,10-bis(chloromethyl)anthracene purchased from TCI and 4-hydroxybenzaldehyde, 1-bromooctadecane purchased from Alfa Aesar were used as received. All other agents and solvents were purchased from commercial sources and used directly without further purification. Tetrahydrofuran (THF) was distilled from sodium/benzophenone. Ultra-pure water was used in the experiments. FL spectra were measured on a PE LS-55 spectrometer with a slit width of 3 nm for both excitation and emission. The FT-IR spectra were obtained in a transmission mode on a Perkin-Elmer Spectrum 100 spectrometer (Waltham, MA, USA). Typically, 8 scans at a resolution of 1 cm−1 were accumulated to obtain one spectrum. TEM images were recorded on a JEM-1200EX microscope operated at 100 kV. The TEM specimens were made by placing a drop of the nanoparticle suspension on a carbon-coated copper grid. The zeta-potential and size distribution of the An18-SiO2 NPs in water were determined using a Zeta Plus apparatus (ZetaPlus, Brookhaven Instruments, Holtsville, NY).
2.2 Preparation of the An18-SiO2 NPs via the Stöber method
The An18 was prepared according to our previous literature.44,55 The chemical structures of An18 and C18-Si are shown in Scheme 2. The luminescent An18-SiO2 NPs were prepared according to the modified Stöber method.54 In brief, 40 mg of An18 was first dispersed in 10 mL THF, and then the An18 dispersion was slowly added into a C18-Si solution (0.8 mL C18-Si in 100 mL of ethanol) under ultrasound irradiation. After removal of the THF using a rotary evaporator at 40 °C, 1.49 mL of distilled water and 2.42 mL of ammonium hydroxide (25%) were added into the mixture. The solution was stirred at room temperature for about 30 min and then 1.6 mL of TEOS in 5 mL ethanol solution was added dropwise into the mixture and stirred at room temperature for another 5 h. Finally, the mixture was centrifuged and washed with water and THF three times to remove the unencapsulated An18. The thus obtained nanoparticles were characterized by a series of techniques and utilized for cell imaging applications.
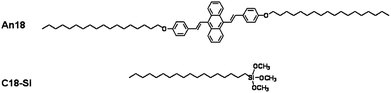 |
| Scheme 2 The chemical structure of An18 (derivatized from 9,10-distyrylanthracene with an alkoxyl endgroup) and octadecyltrimethoxysilane (C18-Si). | |
2.3 Cytotoxicity of the An18-SiO2 NPs
Cell morphology was observed to examine the effects of the An18-SiO2 NPs on human alveolar basal epithelial (A549) adenocarcinoma cells.56–60 Briefly, cells were seeded in 6-well microplates at a density of 1 × 105 cells per mL in 2 mL of respective media containing 10% fetal bovine serum (FBS). After cell attachment, plates were washed with PBS and the cells were treated with complete cell culture medium, or different concentrations of the An18-SiO2 NPs prepared in 10% FBS containing media for 8 and 24 h. Then all the samples were washed with PBS three times to remove the uninternalized An18-SiO2 NPs. The morphology of the cells was observed by using an optical microscope (Leica, Germany), the overall magnification was ×100.
The cell viability of the An18-SiO2 NPs on A549 cells was evaluated by using a cell counting kit-8 (CCK-8) assay based on our previous reports.61–66 Briefly, cells were seeded in 96-well microplates at a density of 5 × 104 cells per mL in 160 μL of respective media containing 10% FBS. After 24 h of cell attachment, the cells were incubated with 10, 20, 40, 80, or 120 μg mL−1 An18-SiO2 NPs for 8 and 24 h. Then the nanoparticles were removed and the cells were washed with PBS three times. 10 μL of CCK-8 dye and 100 μL of DMEM cell culture medium were added to each well and incubated for 2 h at 37 °C. Plates were then analyzed with a microplate reader (VictorIII, Perkin-Elmer). Measurements of formazan dye absorbance were carried out at 450 nm, with the reference wavelength at 620 nm. The values were proportional to the number of live cells. The percent reduction of CCK-8 dye was compared to controls (cells not exposed to the An18-SiO2 NPs), which represented 100% CCK-8 reduction. Three replicate wells were used per microplate, and the experiment was repeated three times. Cell survival was expressed as absorbance relative to that of the untreated controls. Results are presented as mean ± standard deviation (SD). The half maximal inhibitory concentration 50% (IC50) values of the An18-SiO2 NPs were calculated using SPSS 15.0 and used to compare their cytotoxicity.
2.4 Confocal microscopy imaging of cells using An18-SiO2 NPs
The cell uptake of An18-SiO2 NPs was evaluated by confocal microscopy imaging.67–69 Briefly, cells were seeded in a glass bottom dish with a density of 1 × 105 cells per dish. On the day of treatment, the cells were incubated with the An18-SiO2 NPs at a final concentration of 10 μg mL−1 for 3 h at 37 °C. Afterward, the cells were washed three times with PBS to remove the An18-SiO2 NPs and then fixed with 4% paraformaldehyde for 10 min at room temperature. Cell images were taken with a Zeiss 710 3-channel (Zeiss, Germany) confocal laser scanning microscope (CLSM) with the excitation wavelength of 488 nm.
3. Results and discussion
3.1 Characterization of the An18-SiO2 NPs
3.1.1 FT-IR spectra. The successful formation of the An18-SiO2 NPs was confirmed by FT-IR spectroscopy. As shown in Fig. 1, strong absorbance signals with peaks at 2920 and 2845 cm−1 can be observed in the An18 IR spectrum, which can be ascribed to the C–H stretching vibrations of –CH2 and –CH3 groups of the alkyl chain in An18.70,71 On the other hand, a series of peaks located between 1700 and 800 cm−1 can be assigned to the IR signals of aromatic rings, C–O and double bonds in the An18 sample. As compared with the An18 spectrum, the An18 signals can almost not be seen in the sample of the An18-SiO2 NPs.72,73 More importantly, a new peak located at 1072 cm−1 emerged in the sample of the An18-SiO2 NPs, implying the successful encapsulation of An18 in the silica nanoparticles. Based on the experimental procedure, the fabrication of the An18-SiO2 NPs could be divided into two steps. As shown in Scheme 1, the hydrophobic AIE dye (An18) was first self-assembled with C18-Si in an ethanol solution, and then C18-Si was hydrolyzed to form the An18 containing silica core. After that, TEOS was added and coated on the core to form a hydrophilic silica shell. As the An18 was encapsulated in the core of the An18-SiO2 NPs in an aggregated state, the thus obtained silica nanoparticles (An18-SiO2 NPs) are expected to emit strong fluorescence due to their AIE properties.55 On the other hand, because the surface of the An18-SiO2 NPs was covered with the hydrophilic shell, the thus obtained silica nanoparticles are expected to be well dispersed in aqueous solution.
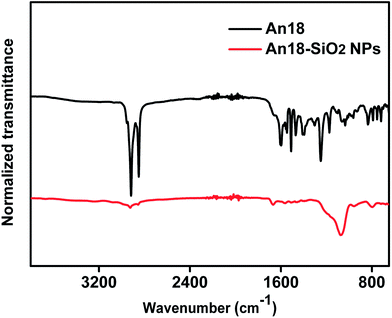 |
| Fig. 1 FT-IR spectra of the An18 and the An18-SiO2 NPs. The disappearance of An18 signals and emergence of Si–O–Si band signals in the sample of the An18-SiO2 NPs demonstrated that An18 was successfully encapsulated in the silica nanoparticles. | |
3.1.2 Optical properties. The optical properties of An18 (in TFH) and the An18-SiO2 NPs (in water) are shown in Fig. 2. As expected, almost no fluorescence can be observed when An18 was dispersed in THF. However, strong yellow fluorescence was emitted when the An18-SiO2 NPs were dispersed in water (inset of Fig. 2). Because the silica nanoparticles were thoroughly washed with water and THF, we believed that the free AIE dye had been completely removed during the washing procedure. Therefore, the luminescence emitted from the An18-SiO2 NPs should be due to the An18, which was encapsulated in the core of silica nanoparticles because free AIE dye had been removed. These results further confirmed the successful encapsulation of An18 in the core of the An18-SiO2 NPs. FL spectra were further employed to examine the fluorescence properties of An18 and the An18-SiO2 NPs and were consistent with the optical images. As shown in Fig. 2, the fluorescence signal of An18 is very weak with an emission peak at 506 nm. After An18 was encapsulated into the silica nanoparticles, the emission peak of the An18-SiO2 NPs was shifted to 522 nm, the red-shift of fluorescence implying the different aggregation states of An18 (in THF) and the silica nanoparticles. On the other hand, the fluorescence intensity of 60 a.u. for An18 in THF, was increased to 620 a.u. for the An18-SiO2 NPs in water, with an approximate 10-fold increase. These results further implied that An18 was successfully encapsulated in the silica nanoparticles. The fluorescence quantum yield of the An18-SiO2 NPs in water was measured using Rhodamine 6G in ethanol as the standard (ΦF = 95%) and the absorbance of the solutions was kept around 0.05 to avoid the internal filter effect. We demonstrated that the ΦF of the An18-SiO2 NPs in pure water is about 5.52%. On the other hand, the photostability of the An18-SiO2 NPs was also measured. We demonstrated that almost no fluorescence intensity decrease was observed after they were irradiated by a UV lamp (λ = 365 nm) for 2 h (Fig. S1†). More important, due to the hydrophilicity of the silica nanoparticles, the thus obtained luminescent nanoparticles showed good dispersion in water (inset of Fig. 2). The size distribution characterized by dynamic laser scattering (DLS) analysis demonstrated that the average size and size distribution of the An18-SiO2 NPs in water is 107.6 ± 3.7 nm with a narrow polydispersity index down to 0.005, evidencing the monodispersity of the An18-SiO2 NPs.
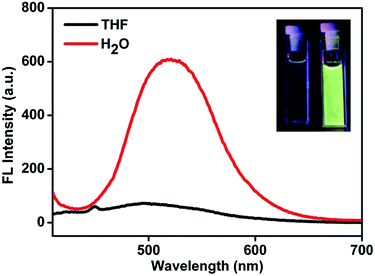 |
| Fig. 2 FL spectra of An18 in THF (black line) and An18-SiO2 NPs in H2O (red line). The inset shows a photograph of the An18 in THF (left cuvette) and An18-SiO2 NPs in H2O (right cuvette). The concentrations of An18 and the An18-SiO2 NPs are 10 and 50 μg mL−1, respectively. | |
3.1.3 Morphology and size characterized by TEM. The morphology and size of the An18-SiO2 NPs were further characterized by TEM. Consistent with the DLS analysis, uniform spherical nanoparticles can clearly be identified by TEM observation at a low magnification (Fig. 3A). Based on the high magnification TEM image, the diameter of most An18-SiO2 NPs is about 70–80 nm (Fig. 3B). On the other hand, the size distribution of the An18-SiO2 NPs was also calculated based on TEM images with more than 100 particles. We demonstrated that over 85% of the nanoparticles have diameters in the range of 70–80 nm (Fig. S2†). As compared with the DLS analysis results, the size determined by TEM is relatively small. This is likely due to the shrinking of silica nanoparticles during the preparation of the TEM samples. The TEM observations further confirmed that luminescent silica nanoparticles were successfully prepared via the modified Stöber method. Because of the controllability of the Stöber method, different AIE dye based silica nanoparticles with different sizes have been previously prepared by Tang et al. They demonstrated that the diameter of silica nanoparticles can be tuned from tens to a few hundred nanometers.54 Therefore, AIE dye based luminescent silica nanoparticles should be of great research interest for the fabrication of highly luminescent nanoprobes, due to their unique AIE properties, facile and controllable synthetic procedure, good water dispersibility and high fluorescence intensity. However, in Tang's work, AIE dyes needed to first be linked with silocanes via a covalent conjugation reaction to obtain an AIE dye contained silica precursor. Therefore, it was relatively complex and specific functional groups were needed on the AIE dye for subsequent conjugation reactions. In the present work, we chose C18-Si as the silica precursor for preparation of the core of luminescent silica nanoparticles via the self assembly of An18 and C18-Si. Then, TEOS was further coated on the luminescent core to fabricate the luminescent core–shell silica nanoparticles (An18-SiO2 NPs). As compared with previous reports, the method described in this work is relative simple. Combined with superior advantages such as controllable morphology, good water dispersibility and high luminescence, the thus obtained luminescent silica nanoparticles are expected to be very promising for biomedical applications.
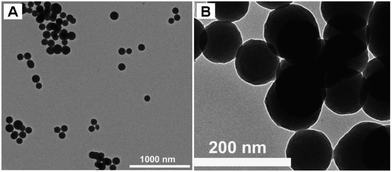 |
| Fig. 3 TEM images of the An18-SiO2 NPs at different magnifications. | |
3.2 Biocompatibility of the An18-SiO2 NPs
To evaluate their potential biomedical applications, the biocompatibility of the An18-SiO2 NPs was determined by optical microscopy observations and a cell viability assay. As shown in Fig. 4A–C, the cells are still strongly attached to the cell culture plate after they were incubated with different concentration of the An18-SiO2 NPs for 24 h. Compared with the control group (cells without An18-SiO2 NPs), all the cells still kept their normal morphology even when the concentration of An18-SiO2 NPs was as high as 120 μg mL−1. No obvious cell number decrease was observed based on the optical microscopy observations (Fig. 4C). These results demonstrated that the An18-SiO2 NPs are very biocompatible with A549 cells. Cell viability results from the CCK-8 assay further demonstrated the excellent biocompatibility of the An18-SiO2 NPs. As shown in Fig. 4D, no obvious cell viability decrease was observed via cell viability examinations. Even when the concentration of An18-SiO2 NPs was as high as 120 μg mL−1, the cell viability of the An18-SiO2 NPs was still greater than 95%. Based on the cell viability results, the IC50 values of the An18-SiO2 NPs are 2099.5 and 659.5 μg mL−1 for 8 h and 24 h, respectively. More importantly, a strong fluorescence signal could be obtained when cells were incubated with only 10 μg mL−1 for 3 h. If we further optimized the synthesis parameters or surface modifications of the An18-SiO2 NPs with targeting agents, the dosage used for cell imaging could be further decreased. Therefore, we believe that the An18-SiO2 NPs are biocompatible enough for biomedical applications.
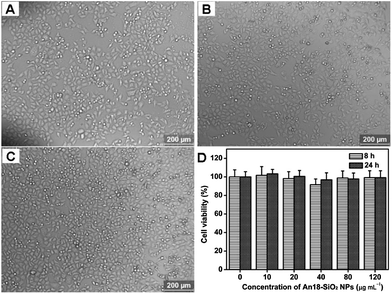 |
| Fig. 4 Biocompatibility evaluation of the An18-SiO2 NPs. (A–C) Optical microscopy images of A549 cells incubated with different concentrations of An18-SiO2 NPs for 24 h: (A) control cells, (B) 20 μg mL−1, (C) 120 μg mL−1. (D) Cell viability of the An18-SiO2 NPs with A549 cells for 8 and 24 h, respectively. Calculated from the CCK-8 results, the IC50 values of the An18-SiO2 NPs are 2099.5 and 659.5 μg mL−1 for 8 h and 24 h incubation, respectively. | |
3.3 Cell imaging applications of An18-SiO2 NPs
The cell imaging applications of the An18-SiO2 NPs were further explored. The CLSM images of cells incubated with 10 μg mL−1 of the An18-SiO2 NPs for 3 h are shown in Fig. 5. The bright field images (Fig. 5A) indicated that cells still kept their normal morphology, further evidencing the good biocompatibility of the An18-SiO2 NPs. When cells were excited with a 488 nm laser, the cell uptake of An18-SiO2 NPs can be clearly distinguished due to the successful staining by the An18-SiO2 NPs. Furthermore many dark areas, which were surrounded by the An18-SiO2 NP areas, could also be found in the CLSM images, which are likely to be the locations of the nuclei of the cells. Given the combination of advantages such as the facile and controllable Stöber method for preparation of luminescent silica nanoparticles, strong fluorescence, good water solubility, excellent biocompatibility and the biodegradable potential of the An18-SiO2 NPs, the luminescent silica nanoparticles obtained in this work, should be highly desirable for bioimaging applications and fabrication of multi-functional theranostic systems.
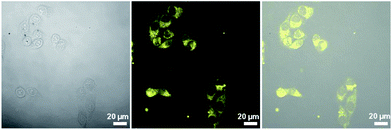 |
| Fig. 5 Confocal imaging of A549 cells. Cells were incubated with 10 μg mL−1 of An18-SiO2 NPs for 3 h. The laser excitation wavelength was 488 nm. | |
4. Conclusions
In summary, a facile method for the preparation of AIE dye (An18) based highly luminescent silica nanoparticles (An18-SiO2 NPs) via a one-pot modified Stöber method was developed for the first time. The thus obtained luminescent nanoparticles exhibited uniform morphology (with diameters about 70–80 nm), strong fluorescence, good water dispersibility and excellent biocompatibility, making the An18-SiO2 NPs promising for cell imaging applications. More importantly, as the An18 was encapsulated in the silica nanoparticles via hydrophobic interaction between An18 and C18-Si, many other AIE dyes might also be encapsulated in silica nanoparticles by the modified Stöber method. Thus numerous luminescent silica nanoparticles with different fluorescent properties might be easily fabricated. Finally, the luminescent silica nanoparticles can be further integrated with other functional components such as magnetic nanoparticles, therapeutic constituents and targeting agents. Thus multifunctional theranostic systems can be also fabricated based on the luminescent silica nanoparticles. Therefore, we believe that the luminescent silica nanoparticles should have a high potential for various biomedical applications.
Acknowledgements
This research was supported by the National Science Foundation of China (no. 21134004, 21201108, 51363016), the National 973 Project (no. 2011CB935700), and the China Postdoctoral Science Foundation (no. 2012M520388, 2012M520243, 2013T60100, 2013T60178).
References
- M. Bruchez, M. Moronne, P. Gin, S. Weiss and A. P. Alivisatos, Science, 1998, 281, 2013–2016 CAS.
- X. Gao, Y. Cui, R. M. Levenson, L. W. K. Chung and S. Nie, Nat. Biotechnol., 2004, 22, 969–976 CAS.
- J. E. Riggs, Z. Guo, D. L. Carroll and Y. P. Sun, J. Am. Chem. Soc., 2000, 122, 5879–5880 CAS.
- Z. Kang, Y. Liu, C. H. A. Tsang, D. D. D. Ma, X. Fan, N. B. Wong and S. T. Lee, Adv. Mater., 2008, 21, 661–664 Search PubMed.
- J. Zheng, J. T. Petty and R. M. Dickson, J. Am. Chem. Soc., 2003, 125, 7780–7781 CAS.
- L. Shang, R. M. Dörlich, S. Brandholt, R. Schneider, V. Trouillet, M. Bruns, D. Gerthsen and G. U. Nienhaus, Nanoscale, 2011, 3, 2009–2014 CAS.
- J. C. Zhou, L. D. Sun, J. Shen, J. Q. Gu and C. H. Yan, Nanoscale, 2011, 3, 1977–1983 CAS.
- D. Yang, X. Kang, M. Shang, G. Li, C. Li and J. Lin, Nanoscale, 2011, 3, 2589–2595 CAS.
- X. Zhang, X. Zhang, S. Wang, M. Liu, Y. Zhang, L. Tao and Y. Wei, ACS Appl. Mater. Interfaces, 2013, 5, 1943–1947 CAS.
- X. Zhang, S. Wang, C. Zhu, M. Liu, Y. Ji, L. Feng, L. Tao and Y. Wei, J. Colloid Interface Sci., 2013, 397, 39–44 CAS.
- X. Zhang, S. Wang, M. Liu, B. Yang, L. Feng, Y. Ji, L. Tao and Y. Wei, Phys. Chem. Chem. Phys., 2013, 15, 19013–19018 CAS.
- X. Zhang, J. Hui, B. Yang, Y. Yang, D. Fan, M. Liu, L. Tao and Y. Wei, Polym. Chem., 2013, 4, 4120–4125 CAS.
- X. Michalet, F. Pinaud, L. Bentolila, J. Tsay, S. Doose, J. Li, G. Sundaresan, A. Wu, S. Gambhir and S. Weiss, Science, 2005, 307, 538–544 CAS.
- P. Couleaud, V. Morosini, C. Frochot, S. Richeter, L. Raehm and J. O. Durand, Nanoscale, 2010, 2, 1083–1095 CAS.
- H. S. Choi, W. Liu, P. Misra, E. Tanaka, J. P. Zimmer, B. I. Ipe, M. G. Bawendi and J. V. Frangioni, Nat. Biotechnol., 2007, 25, 1165–1170 CAS.
- B. Dubertret, P. Skourides, D. J. Norris, V. Noireaux, A. H. Brivanlou and A. Libchaber, Science, 2002, 298, 1759–1762 CAS.
- J. Hui, X. Zhang, Z. Zhang, S. Wang, L. Tao, Y. Wei and X. Wang, Nanoscale, 2012, 4, 6967–6970 CAS.
- K. Patel, S. Angelos, W. R. Dichtel, A. Coskun, Y. W. Yang, J. I. Zink and J. F. Stoddart, J. Am. Chem. Soc., 2008, 130, 2382–2383 CAS.
- X. He, L. Hai, J. Su, K. Wang and X. Wu, Nanoscale, 2011, 3, 2936–2942 CAS.
- Z. Li, J. C. Barnes, A. Bosoy, J. F. Stoddart and J. I. Zink, Chem. Soc. Rev., 2012, 41, 2590–2605 CAS.
- A. Popat, S. B. Hartono, F. Stahr, J. Liu, S. Z. Qiao and G. Q. M. Lu, Nanoscale, 2011, 3, 2801–2818 CAS.
- M. W. Ambrogio, C. R. Thomas, Y. L. Zhao, J. I. Zink and J. F. Stoddart, Acc. Chem. Res., 2010, 44, 903–913 Search PubMed.
- J. M. Rosenholm, C. Sahlgren and M. Lindén, Nanoscale, 2010, 2, 1870–1883 CAS.
- C. R. Thomas, D. P. Ferris, J. H. Lee, E. Choi, M. H. Cho, E. S. Kim, J. F. Stoddart, J. S. Shin, J. Cheon and J. I. Zink, J. Am. Chem. Soc., 2010, 132, 10623–10625 CAS.
- T. D. Nguyen, Y. Liu, S. Saha, K. C. F. Leung, J. F. Stoddart and J. I. Zink, J. Am. Chem. Soc., 2007, 129, 626–634 CrossRef CAS PubMed.
- D. Zhao, J. Feng, Q. Huo, N. Melosh, G. H. Fredrickson, B. F. Chmelka and G. D. Stucky, Science, 1998, 279, 548–552 CrossRef CAS.
- D. Zhao, Q. Huo, J. Feng, B. F. Chmelka and G. D. Stucky, J. Am. Chem. Soc., 1998, 120, 6024–6036 CrossRef CAS.
- Z. Cai, Z. Ye, X. Yang, Y. Chang, H. Wang, Y. Liu and A. Cao, Nanoscale, 2011, 3, 1974–1976 RSC.
- D. Gerion, F. Pinaud, S. C. Williams, W. J. Parak, D. Zanchet, S. Weiss and A. P. Alivisatos, J. Phys. Chem. B, 2001, 105, 8861–8871 CrossRef CAS.
- J. Luo, Z. Xie, J. W. Y. Lam, L. Cheng, H. Chen, C. Qiu, H. S. Kwok, X. Zhan, Y. Liu, D. Zhu and B. Z. Tang, Chem. Commun., 2001, 1740–1741 RSC.
- X. Zhang, Z. Chi, H. Li, B. Xu, X. Li, W. Zhou, S. Liu, Y. Zhang and J. Xu, Chem.–Asian J., 2011, 6, 808–811 CAS.
- X. Zhang, Z. Chi, H. Li, B. Xu, X. Li, S. Liu, Y. Zhang and J. Xu, J. Mater. Chem., 2011, 21, 1788–1796 CAS.
- X. Zhang, Z. Chi, Y. Zhang, S. Liu and J. Xu, J. Mater. Chem. C, 2013, 1, 3376–3390 CAS.
- X. Zhang, M. Liu, B. Yang, X. Zhang and Y. Wei, Colloids Surf., B, 2013, 112, 81–86 CAS.
- Y. Yu, C. Feng, Y. Hong, J. Liu, S. Chen, K. M. Ng, K. Q. Luo and B. Z. Tang, Adv. Mater., 2011, 23, 3298–3302 CrossRef CAS PubMed.
- Z. Li, Y. Q. Dong, J. W. Lam, J. Sun, A. Qin, M. Häußler, Y. P. Dong, H. H. Sung, I. D. Williams and H. S. Kwok, Adv. Funct. Mater., 2009, 19, 905–917 CAS.
- X. Zhang, Z. Yang, Z. Chi, M. Chen, B. Xu, C. Wang, S. Liu, Y. Zhang and J. Xu, J. Mater. Chem., 2010, 20, 292–298 CAS.
- X. Zhang, Z. Chi, B. Xu, H. Li, Z. Yang, X. Li, S. Liu, Y. Zhang and J. Xu, Dyes Pigm., 2011, 89, 56–62 CAS.
- X. Zhang, Z. Chi, B. Xu, H. Li, W. Zhou, X. Li, Y. Zhang, S. Liu and J. Xu, J. Fluoresc., 2011, 21, 133–140 CAS.
- X. Zhang, Z. Chi, B. Xu, C. Chen, X. Zhou, Y. Zhang, S. Liu and J. Xu, J. Mater. Chem., 2012, 22, 18505–18513 CAS.
- X. Zhang, Z. Chi, J. Zhang, H. Li, B. Xu, X. Li, S. Liu, Y. Zhang and J. Xu, J. Phys. Chem. B, 2011, 115, 7606–7611 CAS.
- X. Zhang, Z. Chi, X. Zhou, S. Liu, Y. Zhang and J. Xu, J. Phys. Chem. C, 2012, 116, 23629–23638 CAS.
- X. Zhang, Z. Chi, B. Xu, L. Jiang, X. Zhou, Y. Zhang, S. Liu and J. Xu, Chem. Commun., 2012, 48, 10895–10897 CAS.
- X. Zhang, X. Zhang, B. Yang, S. Wang, M. Liu, Y. Zhang and L. Tao, RSC Adv., 2013, 3, 9633–9636 CAS.
- B. K. An, S. K. Kwon, S. D. Jung and S. Y. Park, J. Am. Chem. Soc., 2002, 124, 14410–14415 CAS.
- Z. Chi, X. Zhang, B. Xu, X. Zhou, C. Ma, Y. Zhang, S. Liu and J. Xu, Chem. Soc. Rev., 2012, 41, 3878–3896 CAS.
- X. Zhang, X. Zhang, B. Yang, Y. Zhang, M. Liu, W. Liu, Y. Chen and Y. Wei, Colloids Surf., B, 2014, 113, 435–441 CAS.
- X. Zhang, X. Zhang, B. Yang, M. Liu, W. Liu, Y. Chen and Y. Wei, Polym. Chem., 2014, 5, 356–360 RSC.
- X. Zhang, X. Zhang, B. Yang, M. Liu, W. Liu, Y. Chen and Y. Wei, Polym. Chem., 2013, 4, 4317–4321 CAS.
- X. Zhang, M. Liu, B. Yang, X. Zhang, Z. Chi, S. Liu, J. Xu and Y. Wei, Polym. Chem., 2013, 4, 5060–5064 CAS.
- X. Zhang, X. Zhang, B. Yang, J. Hui, M. Liu, Z. Chi, S. Liu, J. Xu and Y. Wei, Polym. Chem., 2014, 5, 318–322 CAS.
- X. Zhang, X. Zhang, B. Yang, J. Hui, M. Liu, W. Liu, Y. Chen and Y. Wei, Polym. Chem., 2014, 5, 689–693 RSC.
- M. Faisal, Y. Hong, J. Liu, Y. Yu, J. W. Lam, A. Qin, P. Lu and B. Z. Tang, Chem.–Eur. J., 2010, 16, 4266–4272 CAS.
- F. Mahtab, J. W. Lam, Y. Yu, J. Liu, W. Yuan, P. Lu and B. Z. Tang, Small, 2011, 7, 1448–1455 CAS.
- X. Zhang, X. Zhang, S. Wang, M. Liu, L. Tao and Y. Wei, Nanoscale, 2013, 5, 147–150 CAS.
- L. Xu, X. Zhang, C. Zhu, Y. Zhang, C. Fu, B. Yang, L. Tao and Y. Wei, J. Biomater. Sci., Polym. Ed., 2013, 24, 1564–1574 CAS.
- X. Cai, J. Hao, X. Zhang, B. Yu, J. Ren, C. Luo, Q. Li, Q. Huang, X. Shi and W. Li, Toxicol. Appl. Pharmacol., 2010, 243, 27–34 CAS.
- X. Zhang, J. Yin, C. Kang, J. Li, Y. Zhu, W. Li, Q. Huang and Z. Zhu, Toxicol. Lett., 2010, 198, 237–243 CAS.
- X. Zhang, J. Yin, C. Peng, W. Hu, Z. Zhu, W. Li, C. Fan and Q. Huang, Carbon, 2011, 49, 986–995 CAS.
- Y. Zhu, W. Li, Q. Li, Y. Li, Y. Li, X. Zhang and Q. Huang, Carbon, 2009, 47, 1351–1358 CAS.
- H. Qi, M. Liu, L. Xu, L. Feng, l. Tao, Y. Ji, X. Zhang and Y. Wei, Toxicol. Res., 2013, 2, 427–433 RSC.
- X. Zhang, W. Hu, J. Li, L. Tao and Y. Wei, Toxicol. Res., 2012, 1, 62–68 CAS.
- X. Zhang, H. Qi, S. Wang, L. Feng, Y. Ji, L. Tao, S. Li and Y. Wei, Toxicol. Res., 2012, 1, 201–205 CAS.
- X. Zhang, S. Wang, M. Liu, J. Hui, B. Yang, L. Tao and Y. Wei, Toxicol. Res., 2013, 2, 335–346 CAS.
- X. Zhang, Y. Zhu, J. Li, Z. Zhu, J. Li, W. Li and Q. Huang, J. Nanopart. Res., 2011, 13, 6941–6952 CAS.
- J. Li, Y. Zhu, W. Li, X. Zhang, Y. Peng and Q. Huang, Biomaterials, 2010, 31, 8410–8418 CAS.
- X. Zhang, S. Wang, L. Xu, Y. Ji, L. Feng, L. Tao, S. Li and Y. Wei, Nanoscale, 2012, 4, 5581–5584 CAS.
- B. Yang, Y. Zhang, X. Zhang, L. Tao, S. Li and Y. Wei, Polym. Chem., 2012, 3, 3235–3238 CAS.
- X. Zhang, S. Wang, C. Fu, L. Feng, Y. Ji, L. Tao, S. Li and Y. Wei, Polym. Chem., 2012, 3, 2716–2719 CAS.
- X. Zhang, M. Liu, Y. Zhang, B. Yang, Y. Ji, L. Feng, L. Tao, S. Li and Y. Wei, RSC Adv., 2012, 2, 12153–12155 CAS.
- Y. Cao, X. Zhang, L. Tao, K. Li, Z. Xue, L. Feng and Y. Wei, ACS Appl. Mater. Interfaces, 2013, 5, 4438–4442 CAS.
- X. Zhang, J. Ji, X. Zhang, B. Yang, M. Liu, W. Liu, L. Tao, Y. Chen and Y. WeI, RSC Adv., 2013, 3, 21817–21823 CAS.
- X. Zhang, C. Fu, L. Feng, Y. Ji, L. Tao, Q. Huang, S. Li and Y. Wei, Polymer, 2012, 53, 3178–3184 CAS.
Footnotes |
† Electronic supplementary information (ESI) available. See DOI: 10.1039/c3ra46076b |
‡ These authors contributed equally to this work. |
|
This journal is © The Royal Society of Chemistry 2014 |