DOI:
10.1039/C5RA03408F
(Paper)
RSC Adv., 2015,
5, 32198-32204
A strong charge-transfer effect in surface-enhanced Raman scattering induced by valence electrons of actinide elements†
Received
24th February 2015
, Accepted 31st March 2015
First published on 31st March 2015
Abstract
Surface-enhanced Raman scattering (SERS) is a powerful spectroscopic technique for highly sensitive molecular detection. As the great radial extent of 5f/6d orbitals is enough to support surface plasmons, SERS substrate materials containing actinide elements might bring a novel enhancement mechanism and even significantly enhanced magnitude. Here we study the SERS of a pyridine molecule on the typical actinide embedded structure Ac@Au7 and on a similar structure of pure gold Au8, respectively, using the time-dependent density functional theory (TDDFT) method. The calculated results show that the absorption spectrum of pyridine-Ac@Au7 at 456 nm presents a strong peak belonging to charge-transfer (CT) transition from the metal to the pyridine molecule. Surprisingly, the corresponding CT-SERS enhancement can reach an order of magnitude of 105, which could hardly be achieved in the pure gold systems (about 103–104). Furthermore, electronic structure analysis reveals that the initial orbitals of the CT transition contain a non-ignorable contribution from the 6d electrons of the Ac atom. Meanwhile, the contribution of the 6d electrons can be tuned by changing the conformation of the pyridine-Ac@Au7 complex. The current findings provide a theoretical basis for exploring and synthesizing SERS materials based on actinide elements, which might subsequently facilitate applications of such structures in nanostructural characterization, single-molecule SERS signal detections and biological molecular recognitions etc.
Introduction
It is well known that an appropriate metal substrate is the prerequisite for observing surface-enhanced Raman scattering (SERS), and its composition directly determines the intensity of SERS signals, so how to select components has been a core issue in this analytical technique.1,2 In fact, all metals can enhance the local electromagnetic field more or less, and the intensity of the enhancement mainly depends on the ability of the metals to support the high resonance quality of surface plasmons, i.e., the optical properties of substrate materials directly involved with their electron structures.2 Among them, because the valence shell electrons (…3d104s1 for copper, …4d105s1 for silver, …5d106s1 for gold) are very active chemically, coinage metals have been well designed and fabricated as particularly promising SERS substrate materials.1 Meanwhile, other metal substrate materials have also been widely developed in this field, such as Li, Na, K, Co, Ni, Pt, Rh, Pd etc.2,3 Obviously, for the above mentioned metals, their isoelectronic shells all contain similar unsaturated electrons which make them obtain great SERS enhancement. Thus, actinide elements would have great advantage in supporting surface plasmons due to their chemically hyperactive valence orbital electrons.
Currently, the SERS experiments have found that the valence electrons (5f, 6d) of actinide elements can induce intense charge-transfer (CT) transition between metals and molecules.4 However, because the optical resonance excitation of the system (containing 5f/6d orbitals) is severely affected by the inherent complexity which might arise from the metal nanoparticles, the probe molecule and the metal–adsorbate system, to get a full understanding of SERS enhancement mechanism remain facing enormous challenge. Therefore, a comprehensive theoretical prediction is essential for further exploration and synthesis of multifunctional SERS substrate materials. In single-molecule SERS (SM-SERS) signal detections, the multicomponent substrates not only can control the optical properties of the metal–molecule complexes, but also can facilitate the selection of excitation lights to match the experimental requirements.5–13 Furthermore, our reported works have also pointed out the binary coinage metal clusters have great advantages in tuning the excitation light for SERS spectra, compared with the pure element clusters.12,13 Moreover, although silver is more plasmonically active than gold in SM-SERS and ultrasensitive SERS experiments, gold is more chemically inert, high biocompatible and hypo-toxic, so gold is preferred in medical applications. This means the chemical stability of substrate materials is also more important than maximum enhancement.14 With the successful application of a series of heavy elements (Ac, U, Gd),15–19 the designed mixed-metal of gold–actinides has great applying prospect in biosystem.
Nowadays, the observed huge SERS signal in experiments have two contribution origins:20,21 one is a long-range electromagnetic (EM) effect and the other one is a short-range CT effect also called a chemical (CHEM) enhancement effect. The former is due to the localized surface plasmon resonance at the surface of metal nanoparticles, and the latter is based on a photoinduced transfer of an electron from the Fermi level of the metal to an unoccupied molecular orbital of the adsorbate (metal → molecule) or vice versa. It should be noted that the CT process is closely related to the coupling of the complicated metal surface, adsorbed molecules and other external sources. Until now, the CT enhancement mechanism still cannot be fully explained under certain conditions, despite the existence of the direct or indirect CT effect in some models, such as metal–semiconductor–molecule model and Au/Ag nanoparticles–molecule model etc.22–28 As a consequence, we will focus on the CT enhancement mechanism when the incident light is resonant with a molecular or charge-transfer excitation of the system.
Time-dependent density functional theory (TDDFT) as a useful first-principles method has been widely-used to describe SERS enhancement mechanism, and their theoretical data can also match well with the experimental results.25,29–34 Moreover, pyridine molecule has become one of the most commonly used adsorbates due to its sensitive SERS-active effect.29–32 In this work, a theoretical study of the enhanced Raman scattering of pyridine–actinides model systems is presented by employing the TDDFT method. We anticipate this study will provide a theoretical basis for future study of spectroscopy properties of actinide elements doped gold nanoparticles.
Models and computational methods
It is common knowledge that actinide elements have distinctive optical and magnetic properties, and can present a series of electron transitions in a wide range of energy.35 In addition, the optical and magnetism properties of actinide elements vary with their coordination environment.36 In this case, to explore a stable SERS substrate based on actinide elements is a very challenging, important and interesting work in biomedical applications. With the development of nano-science and technology, planar structures are recently found to be a new class of nanoparticles with highly tunable optical properties, which depend on their own size and composition. These nanoparticles can be designed to either absorb or scatter light in the visible and infrared region where the penetration of light through tissue is maximal.37–40 Based on the above considerations, a typical closed-shell planar structure is selected as SERS substrate material, Ac@Au7, i.e. an actinium atom encapsulated in the center of Au7 ring. When pyridine is adsorbed on metal surface in parallel and vertical directions respectively, the properties of seven gold atoms are equivalent due to Ac@Au7 has a D7h-like symmetry. Moreover, as a comparison, we also selected an existent Au8 planer structure41 as SERS substrate, which is the most similar model to Ac@Au7 and has two different adsorption configurations as shown in Fig. 1.
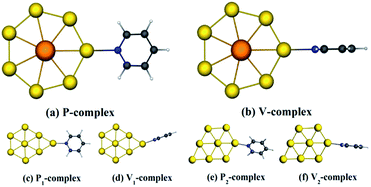 |
| Fig. 1 Stabilized structures of six complexes. P-complex and V-complex represent two Ac@Au7 planar structure with a pyridine molecule absorbed on its surface in parallel and vertical directions, respectively. The subscripts 1 and 2 represent the different adsorption sites of Au8 structure (Ac: orange, Au: luminous yellow, N: deep blue, C: deep grey, H: white). | |
The TDDFT method, which is based on a short-time approximation to the Raman scattering cross section, has been widely used in the theoretical study of SERS. This method not only can deal with the molecule and the metal at the same level of theory, but also can describe the different SERS enhancement mechanisms and their relation to other optical properties in a uniform way, thereby providing accurate microscopic insights into SERS. And its theoretical results for some closed-shell molecular systems are consistent with the experimental data.42,43 All calculations in this study have been done by employing the Amsterdam density functional (ADF) program.44 Considerable researches have shown that the exchange correlation functional BP86 (ref. 45 and 46) is appropriate for describing electronic and optical properties of metal–molecule coupled systems, whether it is pure theory or the combination of the theoretical and experimental work.30,33,42,43 And BP86 functional usually gives harmonic frequencies close to experimental results without the use of scaling factors,47 thereby it is used in this work. Whereas we also used the statistical averaging of orbital potential (SAOP)48,49 to further provide more evidence for the validity of this method. The potential SAOP which displays the correct asymptotic behavior has been specially designed for calculating optical properties.50–52 It has yielded very good results for response properties on gold dimer.50 Relativistic corrections of heavy elements (Au, Ac) have been taken into account through zeroth order regular approximation (ZORA).53–56 A triple-ζ with polarization functions (TZP)57 uncontracted Slater-type orbital basis set have been used, with a [1s2–4d10] frozen core for Au and Ac, and with full electrons for N, C, and H. The geometry optimizations have been performed without imposing any symmetry constraint. All obtained structures were analyzed with vibration frequency calculations at the same level to avoid imaginary frequencies.
Absolute Raman intensities are presented as the differential Raman scattering cross-section (DRSC). For Stokes scattering with an experimental setup of a 90° scattering angle and perpendicular plan-polarized light, the cross-section is given:58
here,
ω0 and
ωi respectively denote the frequencies of the incident light and the
ith vibrational mode;

and

respectively denote the derivatives of the isotropic and anisotropic polarizability of the
ith vibrational mode; and
h (Planck constant),
c (light speed),
kB (Boltzmann constant), and
T (Kelvin temperature) also respectively denote corresponding physical quantities. The finite lifetime is included phenomenologically using a damping parameter
Γ ≈ 0.004 a.u., which is reasonable for metal clusters and resonant Raman intensities.
29
Results and discussion
UV-visible absorption spectra
The absorption spectra of optimized complexes are obtained by TDDFT calculations in the range of ultraviolet visible (UV-vis) light, because the excitation lights are often used in SERS experiments, especially in SM-SERS detections.3,59–61 And the specific changes in the absorption spectra of the six complexes are shown in ESI Part 1.† For Ac@Au7 planar structure, the UV-vis spectrum is principally composed of intracluster excitation (IE). However, when pyridine molecule is adsorbed on it in the form of parallel and vertical two types, in addition to intracluster transition contribution to UV-vis spectrum, the interaction between pyridine and Ac@Au7 nanoparticle can also result in a new CT excitation transitions from the metal to the molecule. As mentioned in the introduction, the CT resonant mechanism is very important when the incident light is resonant with a molecular or CT excitation of the system. Therefore, to further understand the electron transitions behavior of the adsorption systems, we listed the typical CT excitation energies in Table 1 (the additional CT excitation energies are shown in ESI Part 2†). Among them, the CT excitation energy of V-complex is about 0.63 eV lower than that of P-complex, which is similar to the corresponding energy difference between surface-complex and vertex-complex for Ag20 cluster that is about 0.50 eV.29 The difference of CT excited energy is easily understood if one consider the orbitals involved in the transitions. From Table 1 it is apparent that the electron transitions all from occupied MOs to third lowest unoccupied MO (LUMO+2), while the second highest unoccupied MO (HOMO−1) of V-complex is more active than the HOMO−5 of P-complex.
Table 1 Calculated excitation energies (E in eV), excitation wavelengths (λ in nm), oscillator strengths (f) and orbital transitions of CT excitations for six complexes, respectively
Geometry |
E/λ |
f |
Transitions |
P-complex |
3.35/370 |
0.0058 |
HOMO−5 → LUMO+2 |
V-complex |
2.72/456 |
0.0976 |
HOMO−1 → LUMO+2 |
P1-complex |
3.21/387 |
0.0231 |
HOMO−10 → LUMO+2 |
V1-complex |
2.46/505 |
0.0151 |
HOMO−1 → LUMO+2 |
P2-complex |
2.75/450 |
0.0588 |
HOMO−6 → LUMO+2 |
V2-complex |
2.78/446 |
0.0440 |
HOMO−5 → LUMO+2 |
In most cases, the intensity of resonance Raman scattering is approximately proportional to the square of oscillator strength. By comparison, we found that V-complex reveals the larger oscillator strength than any other complex, which might induce a strong resonance-enhanced Raman signal. To confirm the accuracy of CT excited energy (2.72 eV) for V-complex, we performed the calculation of absorption spectrum at SAOP/TZP level, and found that the typical CT excitation energy increased by only 0.10 eV comparing with that at BP86/TZP level. In addition, the typical IEs excitation energies are listed in ESI Part 3.† First, for pyridine-Ac@Au7 complexes in Table S2,† the orbitals involved in excited transitions are all from HOMOs of Ac@Au7 to unoccupied MOs (above LUMO) of pyridine. In contrary, for the pyridine–Au8 complexes, the electron transitions are from occupied MOs (below HOMO) of Au8 to LUMO or LUMO+1 of pyridine. Thus, whether in the CT or IEs excited transitions, there are many obvious differences among these six complexes, although they are all formed by pyridine adsorption on gold atoms.
Enhanced Raman scattering
In order to explore how the interaction between the molecule and the metal affect the intensity of the Raman scattering, we firstly analyzed the well-known six vibrational modes of the pyridine molecule, i.e. ring deformation (598 cm−1), ring breathing (978 cm−1 and 1021 cm−1) and ring stretch vibrational modes (1205 cm−1, 1468 cm−1, 1568/1574 cm−1), which have very obvious signals strength in SERS experiment.3,62 Fig. 2a shows that the static DRSC is of the order of 10−31 cm2 sr−1 for pyridine alone, which is consistent with that of previous reported theoretical data.30 The static Raman scattering spectra of pyridine is mainly dominated by ring breathing and stretch modes, and the relative Raman intensity of these vibrational modes is closely related to the adsorption site of pyridine. For P-complex (Fig. 2b), the maximum Raman peak corresponds to ring breathing vibrational modes. Its intensity enhances by a factor of about 4 than that of pyridine alone, and several new vibration peaks appear in the SERS spectrum. For V-complex (Fig. 2c), the maximum Raman peak corresponds to ring stretch vibrational mode, and its intensity increases by 16 times. The relative intensities might be attributed to sensitive to adsorption orientation of pyridine molecule. Next, for the other four complexes (see ESI Part 4†), their maximum Raman peaks are also correspond to ring stretch vibrational modes, and their enhancements range from 10 to 40 times. Besides the enhancement of intensity, when pyridine is adsorbed on metal surface, the typical Raman peaks will show a blue shift about 1–40 cm−1. This results are consistent with the recent theoretical calculation for a pyridine adsorbed on Ag20 surface.29 All these changes are induced owing to the polarization effect caused by charge redistribution of complex.
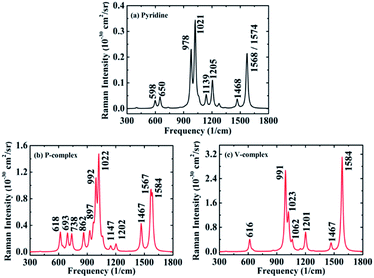 |
| Fig. 2 Calculated static Raman spectra of pyridine alone (a), P-complex (b), V-complex (c). Differential cross-section is measured in the unit of 10−30 cm2 sr−1. Spectra have been broadened by a Lorentzian having a width of 20 cm−1. | |
Next, the SERS spectra based on typical CT resonance excitations are shown in Fig. 3 in the range of 300 to 1800 cm−1. By comparison, we found that the CT resonance-enhanced Raman spectra are mainly dominated by ring breathing and stretch vibrational modes, and other weaker vibrational modes (see Fig. 3). This relative Raman intensity is closely related to the adsorption site of pyridine and the composition of SERS substrate materials. For P-complex, the typical absorption peaks located at 370 nm is denoted as SERS (370 nm) which is presented in Fig. 3a. The Raman scattering intensity is in the order of 10−28 cm2 sr−1, presenting an enhancement of the order of 103 compared to the 10−31 cm2 sr−1 of pyridine. For V-complex, the typical absorption peaks located at 456 nm is denoted as SERS (456 nm) which is shown in Fig. 3b. The intensity of the order of 10−26 cm2 sr−1 corresponds to an enhancement of the order of 105. Next, we also simulated the CT resonance-enhanced Raman spectra for pyridine-Au@Au7 complexes under their own corresponding excited light. Among them, although the CT enhancement factor is of the order of 104 for P2-complex and V2-complex, it is still 1 order of magnitude lower than that of V-complex, and these complexes are distorted seriously. In addition to list the typical CT excitation energies in main text, some additional CT excitation energies have been considered (see ESI Part 2†). However, due to the intensity of resonance Raman scattering is approximately proportional to the square of oscillator strength, the corresponding CT-SERS largest enhancement only can reach the order of magnitude of 103.
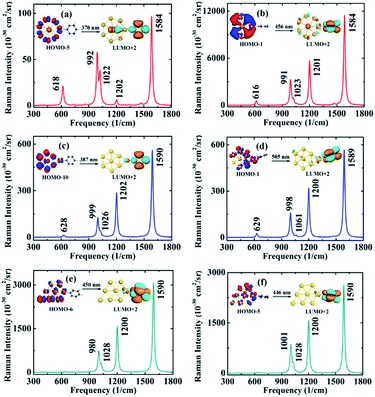 |
| Fig. 3 Calculated CT resonance-enhanced Raman spectra for six complexes at the corresponding incident wavelength. (a) P-complex, (b) V-complex, (c) P1-complex, (d) V1-complex, (e) P2-complex, (f) V2-complex. | |
Therefore, from the above comparison, we found that the SERS enhancement intensity has a significant difference between P-complex and V-complex, even though they all have the same metal substrate. That might result from the difference of the initial orbitals involved in electron transitions. Furthermore, molecular orbital analysis shows that the SERS (456 nm) electron transitions from the 6d–5d6s (6d orbital of Ac, 5d6s hybrid orbital of Au7 ring) hybrid bonding orbital to the π orbital of pyridine and slight charge transfers to the metal (HOMO−1 → LUMO+2). For other five complexes, the electrons involved in excited transitions are all from 5d or 5d6s hybrid orbitals of Au7 ring. Through the above analysis, it is clear that the originate of electron transition (HOMO−1) in V-complex contain non-ignorable contribution from 6d electrons of the Ac atom. However, it is not contained in the originate in other five complexes. Therefore, the 6d electron involved in excited transitions is more likely to lead to a strong CT enhancement.
The deformation densities analysis can reveal visually photoinduced charge transfer, which has been used in SERS studies.29,34 From Fig. 4 we can clearly see that a majority of the electron exchanges between Ac@Au7 and pyridine take place in the junction of molecule–metal complexes (within black frame). However, for pyridine–Au8 complexes, the electron exchange occurs in overall systems (Fig. 4). Zayak et al. have confirmed that the intense electron exchange in interface is beneficial to chemical enhancement.63 Therefore, Ac@Au7 is more suitable to be used as SERS metal substrate material compared with pure gold system. However, for P-complex and V-complex, although they all have the same metal substrate, their Raman enhancement intensities are obviously different. To explore how the adsorption structural difference affect the Raman scattering intensity, we further analysis the process of electron exchange for P-complex and V-complex. In V-complex, the process is accomplished just in Au–N bonding region (Fig. 4b). However, in P-complex, with the exception of the Au–N bonding region, we also saw enhanced electron density in the Au–H bonding region (Fig. 4a). When the incident light is resonant with CT excitation of system, the electron involved in excited transition is delocalized on the molecular N–C ring, not on the H atoms. This means, the flow of electron density from the H atoms to Au atoms occurs in the Au–H bonding region, (Fig. 4a, within black frame), which is enough to weaken the intensity of Au–N bonding, and then reduce the CT-SERS enhancement of P-complex. Therefore, all these facts explain why the Raman enhancement intensity of V-complex is greater than that of P-complex.
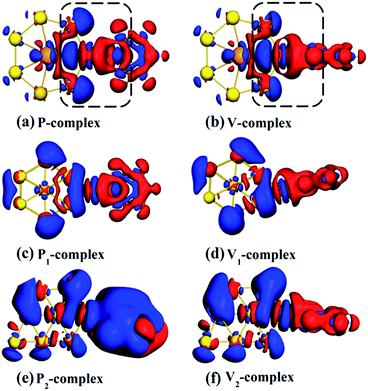 |
| Fig. 4 Calculated deformation density (Δρ = ρAc@Au7+pyridine − ρAc@Au7 − ρpyridine) isosurface for the six complexes. Red and blue respectively denote an enhanced and a depleted electron density (isosurface value = 0.0004 a.u.). | |
The previous SERS experiment about actinide elements has shown that 6d electron involves in electron transitions and leads to greater intensity in the CT transition.4 Through the above comparative analysis, the CT-SERS enhancement of V-complex is the strongest, and from Fig. 3 it can be seen clearly that 6d electron of Ac atom did participate in CT excited transition of system. Inversely, although 6d electron also involved in the electron transitions in P-complex, due to pyridine molecule is adsorbed on Ac@Au7 in a parallel direction, the contribution of 6d electron to excited transition is weakened. As a whole, it can be confirmed the 6d electron of actinide element induces a strong CT enhancement effect.
Furthermore, to get a better understanding of the bonding characteristics between metal and molecule, we performed energy decomposition analysis (EDA) for P-complex and V-complex (see Table 2). Basis set superposition errors (BSSE) have been accounted for using the counterpoise method64 by calculating the bonding energies with respect to the isolated fragments. It should be noted that, if the stabilized complex has a good symmetry, it could be seen as a typical model system. Since the conformations of pyridine-Au@Au7 complexes are distorted seriously, we would not perform EDA for them. In Table 2, the Au–N bondings of the two complexes are mainly dominated by electrostatic interaction (70.51/71.34%). And despite the proportion of orbital interaction term (29.49/28.66%) in attractive term is small, it suggests an important polarization of the electron density on the surface of the cluster. The total binding energy between Ac@Au7 and pyridine nicely agrees with previous reported results of another complex which is also with pyridine but adsorption on Ag20 nanoparticles.29 In addition, because of the increase of electron density in Au–H bonding region (see Fig. 4), the total binding energy of P-complex is a little lower than that of V-complex. To quantify the charge density redistribution occurring upon pyridine bonding, atomic charges have been computed within the VDD scheme. We found that the electron exchange of V-complex is indeed more drastic than that of P-complex in Au–N bonding region, and the Au–N bond length is also shorter.
Table 2 Calculated energy decomposition analysis (kcal mol−1), Au–N bond length (Å) and Voronoi deformation density (VDD)44 charge (e) transferred for the two complexes. Values in parentheses give the percentage of each attractive term with respect to the sum of the attractive termsa
|
P-complex |
V-complex |
ΔEelectrostatic is the classical electrostatic interaction term; ΔEPauli is the Pauli repulsion term; ΔEorbital is the orbitals interaction term. Within this energy decomposition scheme the attractive and repulsive terms are negative and positive, respectively. |
ΔEelectrostatic |
−45.97 (70.51%) |
−46.17 (71.34%) |
ΔEPauli |
56.62 |
57.12 |
ΔEorbital |
−19.23 (29.49%) |
−18.55 (28.66%) |
Etotal binding energy |
−8.58 |
−7.60 |
Etotal binding energy (BEES corrected) |
−4.23 |
−3.94 |
Au–N bond length |
2.43 |
2.42 |
VDD charge (molecule → metal) |
0.16 |
0.18 |
The binding properties of Ac@Au7
In addition, to more demonstrate the reliability of our selected model, we analyzed the binding properties of Ac@Au7 planar structure. The bonding in the Ac@Au7 planar structure can be viewed as an Au7 ring interacting with an Ac atom, which possesses a s1, d3 valence configuration for Au, Ac, respectively, (i.e., the 7s electrons are promoted to the 6d orbitals). The 10 valence electrons in this planar structure represent a closed-shell electronic structure (Fig. 5), which is consistent with previous reported structures, such as Ti@Au6, Sc@Cu7.65,66 Among them, the two in-plane 6d orbitals (dxy and dx2−y2) of Ac atom play a positive role in the bonding. The average total binding energy per atom and HOMO–LUMO gap for Ac@Au7 are 2.70 eV and 2.42 eV, respectively. In general, nanocluster structures with binding energy greater than 2.40 eV and HOMO–LUMO gap larger than 1.70 eV are commonly regarded to be stable.67 Therefore, our current results suggested that Ac@Au7 belongs to stable structures similar to those previously reported, such as W@Au12, U@Au14, Sc@Au15 etc.68–70
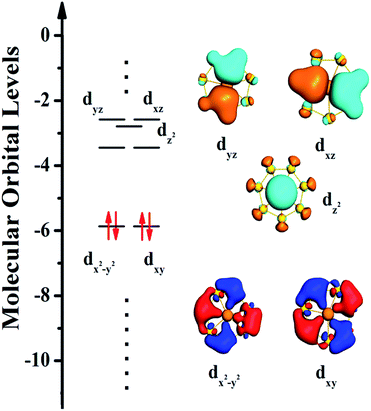 |
| Fig. 5 Molecular orbitals (MOs) and MO energy level correlation diagram for Ac@Au7 planar structure. | |
Conclusions
In this work, we have researched the SERS enhancement mechanism of pyridine-Ac@Au7 and pyridine–Au8 model systems using TDDFT method. By calculation and comparison of the CT-SERS enhancement of these six complexes, we found that the SERS enhancement of V-complex is the strongest and can reach the order of magnitude of 105 at an incident wavelength of 456 nm, which could hardly be achieved in the pure gold system (about 103–104). Together with a detailed molecular orbital analysis, we surprisingly found that the initial occupied molecular orbital involved in CT excited transition in V-complex contains non-ignorable contribution from 6d electron which is not found in other five complexes. Furthermore, just as the visually deformation density isosurface shows, although the electron exchanges are drastic in Au–N bonding regions of both P-complex and V-complex, the electron exchange process occur in the Au–H bonding region is very strong enough to weaken the intensity of electron exchange of Au–N bonding. This weakening phenomenon is more specifically reflected in the charge transfer and interaction energy between the metal cluster and the molecule. Besides, the electronic structure analysis indicates that, Ac@Au7 planar structure is stabilized by covalent interaction.
Spectroscopic studies of these systems that focus on molecular excited-state properties (e.g. charge-transfer states) promise a further understanding of actinide electronic structure and actinide-molecular bonding schemes. In recent years, the SERS experiment about U atom has found that the 6d orbital involved in electronic transition have greater radial extent and might lead to greater intensity in the CT transition compared with 5f orbital.4 However, the SERS enhancement mechanism of mixed-metal substrate materials, which is consisted by actinide elements and gold nanoparticles, is still unclear. Based on a comprehensive prediction for the enhanced Raman scattering of the gold-ring structure with embedded Ac atom, we hope this study will contribute to providing a theoretical basis for the research and synthesis of new SERS substrate materials.
Acknowledgements
We would like to thank the support of the National Science Foundation of China (11374004, 11034003) and the Science and Technology Development Program of Jilin Province of China (20150519021JH). Z.W. also acknowledges the assistance of the Fok Ying Tung Education Foundation (142001) and the High Performance Computing Center (HPCC) of Jilin University.
Notes and references
- L. Jensen, C. M. Aikens and G. C. Schatz, Chem. Soc. Rev., 2008, 37, 1061–1073 RSC.
- D. Y. Wu, J. F. Li, B. Ren and Z. Q. Tian, Chem. Soc. Rev., 2008, 37, 1025–1041 RSC.
- Z. Q. Tian, B. Ren and D. Y. Wu, J. Phys. Chem. B, 2002, 106, 9463–9483 CrossRef CAS.
- R. E. D. Re, K. C. Jantunen, J. T. Golden, J. L. Kiplinger and D. E. Morris, J. Am. Chem. Soc., 2005, 127, 682–689 CrossRef PubMed.
- R. Olivares-Amaya, D. Rappoport, P. A. Munoz, P. Peng, E. Mazur and A. Aspuru-Guzik, J. Phys. Chem. C, 2012, 116, 15568–15575 CAS.
- P. P. Patra and G. V. P. Kumar, J. Phys. Chem. Lett., 2013, 4, 1167–1171 CrossRef CAS.
- A. Sivanesan, E. Witkowska, W. Adamkiewicz, L. Dziewit, A. Kaminska and J. Waluk, Analyst, 2014, 139, 1037–1043 RSC.
- J. Suntivich, Z. Xu, C. E. Carlton, J. Kim, B. Han, S. W. Lee, N. Bonnet, N. Marzari, L. F. Allard, H. A. Gasteiger, K. Hamad-Schifferli and Y. Shao-Horn, J. Am. Chem. Soc., 2013, 135, 7985–7991 CrossRef CAS PubMed.
- Y. Wang, L. Chen and P. Liu, Chem.–Eur. J., 2012, 18, 5935–5943 CrossRef CAS PubMed.
- Y. Yin, T. Qiu, L. Ma, X. Lang, Y. Zhang, G. Huang, Y. Mei and O. G. Schmidt, J. Phys. Chem. C, 2013, 116, 25504–25508 Search PubMed.
- Y. Zhou, C. Lee, J. Zhang and P. Zhang, J. Mater. Chem. C, 2013, 1, 3695–3699 RSC.
- L. Chen, Z. Li, Y. Meng, M. Lu, Z. Wang and R. Q. Zhang, J. Phys. Chem. C, 2013, 117, 12544–12551 CAS.
- L. Chen, Y. Gao, H. Xu, Z. Wang, Z. Li and R. Q. Zhang, Phys. Chem. Chem. Phys., 2014, 16, 20665–20671 RSC.
- S. Schlucker, Angew. Chem., Int. Ed., 2014, 53, 4756–4795 CrossRef PubMed.
- G. Henriksen, O. S. Bruland and R. H. Larsen, Anticancer Res., 2004, 24, 101–106 CAS.
- S. N. Nahar, A. K. Pradhan and S. Lim, Can. J. Phys., 2011, 89, 483–494 CrossRef CAS.
- S. G. Kang, G. Zhou, P. Yang, Y. Liu, B. Sun, T. Huynh, H. Meng, L. Zhao, G. Xing, C. Chen, Y. Zhao and R. Zhou, Proc. Natl. Acad. Sci. U. S. A., 2012, 109, 15431–15436 CrossRef CAS PubMed.
- S. G. Kang, T. Huynh and R. Zhou, Nanoscale, 2013, 5, 2703–2712 RSC.
- S. G. Kang, T. Huynh and R. Zhou, Sci. Rep., 2012, 2, 957 Search PubMed.
- H. Xu, E. J. Bjerneld, M. Käll and L. Börjesson, Phys. Rev. Lett., 1999, 83, 4357 CrossRef CAS.
- M. Moskovits, Rev. Mod. Phys., 1985, 57, 783–826 CrossRef CAS.
- B. Nikoobakht, J. Wang and M. A. El-Sayed, Chem. Phys. Lett., 2002, 366, 17–23 CrossRef CAS.
- E. Hao and G. C. Schatz, J. Chem. Phys., 2004, 120, 357–366 CrossRef CAS PubMed.
- D. P. Fromm, A. Sundaramurthy, P. J. Schuck, G. Kino and W. E. Moerner, Nano Lett., 2004, 4, 957–961 CrossRef CAS.
- L. Zhao, L. Jensen and G. C. Schatz, Nano Lett., 2006, 6, 1229–1234 CrossRef CAS PubMed.
- Z. Sun, C. Wang, J. Yang, B. Zhao and J. R. Lombardi, J. Phys. Chem. C, 2008, 112, 6093–6098 CAS.
- L. Peyser-Capadona, J. Zheng, J. I. Gonzalez, T. H. Lee, S. A. Patel and R. M. Dickson, Phys. Rev. Lett., 2005, 94, 058301 CrossRef.
- C. H. Xiao, B. X. Xiao, Y. D. Wang, J. Zhang, S. M. Wang, P. Wang, T. Yang, R. Zhao, H. Yu, Z. F. Li and M. Z. Zhang, RSC Adv., 2015, 5, 17945–17952 RSC.
- L. Zhao, L. Jensen and G. C. Schalz, J. Am. Chem. Soc., 2006, 128, 2911–2919 CrossRef CAS PubMed.
- L. Jensen, L. L. Zhao and G. C. Schatz, J. Phys. Chem. C, 2007, 111, 4756–4764 CAS.
- D. Y. Wu, M. Hayashi, C. H. Chang, K. K. Liang and S. H. Lin, J. Chem. Phys., 2003, 118, 4073 CrossRef CAS PubMed.
- D. Y. Wu, M. Hayashi, S. H. Lin and Z. Q. Tian, Spectrochim. Acta, Part A, 2004, 60, 137–146 CrossRef CAS.
- N. Valley, N. Greeneltch, R. P. Van Duyne and G. C. Schatz, J. Phys. Chem. Lett., 2013, 4, 2599–2604 CrossRef CAS.
- L. Xia, M. Chen, X. Zhao, Z. Zhang, J. Xia, H. Xu and M. Sun, J. Raman Spectrosc., 2014, 45, 533–540 CrossRef CAS.
- J. J. Katz, G. T. Seaborg and L. R. Morss, The Chemistry of the Actinide Elements, Chapman and Hall, New York, 2nd edn, 1986 Search PubMed.
- G. Tian, J. Xu and L. Rao, Angew. Chem., 2005, 117, 6356–6359 CrossRef.
- L. R. Hirsch, A. M. Gobin, A. R. Lowery, F. Tam, R. A. Drezek, N. J. Halas and J. L. West, Ann. Biomed. Eng., 2006, 34, 15–22 CrossRef PubMed.
- Z. Li, Z. Liu, J. Zhang, B. Han, J. Du, Y. Gao and T. Jiang, J. Phys. Chem. B, 2005, 109, 14445–14448 CrossRef CAS PubMed.
- C. Zhu, G. Meng, Q. Huang, Z. Zhang, Q. Xu, G. Liu, Z. Huang and Z. Chu, Chem. Commun., 2011, 47, 2709–2711 RSC.
- P. Xu, B. Zhang, N. H. Mack, S. K. Doorn, X. Han and H.-L. Wang, J. Mater. Chem., 2010, 20, 7222–7226 RSC.
- H. Hakkinen, B. Yoon, U. Landman, X. Li, H. Zhai and L.-S. Wang, J. Phys. Chem. A, 2003, 107, 6168–6175 CrossRef.
- A. Mohammed, W. Hu, P. O. Andersson, M. Lundquist, L. Landström, Y. Luo and H. Ågren, Chem. Phys. Lett., 2013, 581, 70–73 CrossRef CAS PubMed.
- X. Zhao and M. Chen, J. Raman Spectrosc., 2014, 45, 62–67 CrossRef CAS.
- G. Te Velde, F. M. Bickelhaupt, E. J. Baerends, C. Fonseca Guerra, S. J. A. Van Gisbergen, J. G. Snijders and T. Ziegler, J. Comput. Chem., 2001, 22, 931–967 CrossRef CAS.
- A. D. Becke, Phys. Rev. A, 1988, 38, 3098–3100 CrossRef CAS.
- J. Perdew, Phys. Rev. B: Condens. Matter Mater. Phys., 1986, 33, 8822–8824 CrossRef.
- J. Neugebauer and B. A. Hess, J. Chem. Phys., 2003, 118, 7215–7225 CrossRef CAS PubMed.
- O. V. Gritsenko, P. R. T. Schipper and E. J. Baerends, Chem. Phys. Lett., 1999, 302, 199–207 CrossRef CAS.
- P. R. T. Schipper, O. V. Gritsenko, S. J. A. van Gisbergen and E. J. Baerends, J. Chem. Phys., 2000, 112, 1344 CrossRef CAS PubMed.
- K. R. Geethalakshmi, F. Ruiperez, S. Knecht, J. M. Ugalde, M. D. Morse and I. Infante, Phys. Chem. Chem. Phys., 2012, 14, 8732–8741 RSC.
- F. Rabilloud, J. Chem. Phys., 2014, 141, 144302 CrossRef PubMed.
- B. Anak, M. Bencharif and F. Rabilloud, RSC Adv., 2014, 4, 13001 RSC.
- E. V. Lenthe, A. Ehlers and E. J. Baerends, J. Chem. Phys., 1999, 110, 8943 CrossRef PubMed.
- E. V. Lenthe, E. J. Baerends and J. G. Snijders, J. Chem. Phys., 1993, 99, 4597–4610 CrossRef PubMed.
- E. V. Lenthe, J. G. Snijders and E. J. Baerends, J. Chem. Phys., 1996, 105, 6505–6516 CrossRef PubMed.
- E. V. Lenthe, E. J. Baerends and J. G. Snijders, J. Chem. Phys., 1994, 101, 9783–9792 CrossRef PubMed.
- E. V. Lenthe and E. J. Baerends, J. Comput. Chem., 2003, 24, 1142–1156 CrossRef PubMed.
- J. Neugebauer, M. Reiher, C. Kind and B. A. Hess, J. Comput. Chem., 2002, 23, 895–910 CrossRef CAS PubMed.
- M. G. Albrecht and J. A. Creighton, J. Am. Chem. Soc., 1977, 99, 5215–5217 CrossRef CAS.
- P. R. Carey, J. Biol. Chem., 1999, 274, 26625–26628 CrossRef CAS PubMed.
- M. Moskovits, J. Raman Spectrosc., 2005, 36, 485–496 CrossRef CAS.
- D. Y. Wu, X. M. Liu, S. Duan, X. Xu, B. Bin, S. H. Lin and Z. Q. Tian, J. Phys. Chem. C, 2008, 112, 4195–4204 CAS.
- A. T. Zayak, Y. S. Hu, H. Choo, J. Bokor, S. Cabrini, P. J. Schuck and J. B. Neaton, Phys. Rev. Lett., 2011, 106, 083003 CrossRef CAS.
- S. F. Boys and F. Bernardi, Mol. Phys., 1970, 19, 553–566 CrossRef CAS.
- X. Li, B. Kiran, L.-F. Cui and L.-S. Wang, Phys. Rev. Lett., 2005, 95, 253401 CrossRef.
- T. Holtzl, E. Janssens, N. Veldeman, T. Veszpremi, P. Lievens and M. T. Nguyen, ChemPhysChem, 2008, 9, 833–838 CrossRef CAS PubMed.
- Y. Gao, S. Bulusu and X. C. Zeng, ChemPhysChem, 2006, 7, 2275–2278 CrossRef CAS PubMed.
- P. Pyykko and N. Runeberg, Angew. Chem., Int. Ed., 2002, 114, 2278–2280 CrossRef.
- Y. Gao, S. Bulusu and X. C. Zeng, J. Am. Chem. Soc., 2005, 127, 15680–15681 CrossRef CAS PubMed.
- Y. Gao, X. Dai, S. G. Kang, C. A. Jimenez-Cruz, M. Xin, Y. Meng, J. Han, Z. Wang and R. Zhou, Sci. Rep., 2014, 4, 5862 CAS.
Footnote |
† Electronic supplementary information (ESI) available: The absorption spectra of six complexes. The additional CT excitation energies of six complexes. The IEs of pyridine-Ac@Au7 and pyridine–Au8 complexes. Calculated static Raman spectra of pyridine–Au8 complexes. See DOI: 10.1039/c5ra03408f |
|
This journal is © The Royal Society of Chemistry 2015 |
Click here to see how this site uses Cookies. View our privacy policy here.