Effect of emerging contaminants on soil microbial community composition, soil enzyme activity, and strawberry plant growth in polyethylene microplastic-containing soils†
Received
26th September 2022
, Accepted 15th February 2023
First published on 24th February 2023
Abstract
Microplastic pollution is commonly found in terrestrial ecosystems, yet few studies have investigated its impact on agricultural soils. Herein, we examine the effects of emerging contaminants and high-density polyethylene (HDPE) microplastics (<1 mm) on soil microbial community composition, soil enzyme activity, and strawberry plant growth. The study was conducted outdoors over the span of two years near Montreal, Canada. We found that soils containing emerging contaminants had a significant weighted UniFrac distance from control soils (q = 0.0076). However, when the emerging contaminants were in the presence of 100 mg kg−1 HDPE microplastics, the distance from the control was no longer significant (q = 0.1862). This suggests that HDPE microplastics may mitigate the impact of the emerging contaminants on the soil microbial community. Conversely, no significant differences among treatments were observed in the Shannon's diversity index of the soil microbial community throughout the study. Significant differences in the soil enzyme activity among treatments were rare. At the end of each year's growing season, there were no significant differences in the dry plant biomass, strawberry yield, and the number of leaves and flower stalks between treatments. Many of the endpoints measured showed no significant differences between treatments. Nonetheless, sharing the results of this two-year study, performed outdoors using environmentally relevant concentrations, is important to prevent biased literature and the repetition of resource intensive experiments.
Environmental significance
Many plastics are released into the environment upon disposal where they break down over time into microplastics. The widespread use of pharmaceutically active compounds has led to their detection in wastewater. During the wastewater treatment process these microplastics and compounds can enter the sewage sludge, which can then be converted into biosolids for use in agricultural soils as fertilizer. Therefore, it is important to understand their effects on agricultural soils. In our study performed outdoors over two growing seasons we found that the pharmaceutically active compounds were able to alter the soil microbial community but were unable to do so when HDPE microplastics were present, indicating that the microplastics may play a role in mitigating the impact of these compounds.
|
1 Introduction
The occurrence of microplastics (plastic particles less than 5 mm in size) in our terrestrial and aquatic systems is an environmental problem that is expected to persist even if global plastic use were to end.1 A survey of the literature found that less than 10% of scientific publications on microplastic pollution focused on terrestrial systems whereby most studies focused on aquatic systems.2 Meanwhile, in Europe, it is estimated that 4–23 times as much plastic waste is released into continental environments when compared to oceans.3 Microplastics have even been found in remote catchments of the Pyrenees mountain range and have been shown to travel up to 95 km via atmospheric transport.4 A survey of soils from an industrial area in Sydney, Australia, found that microplastic concentrations varied from 300 to 67
500 mg kg−1.5 Moreover, besides landfills, urban areas, and beaches, agroecosystems are suspected to be the most plastic-contaminated terrestrial system.6 For instance, Piehl et al. measured the quantity of microplastics found in agricultural soils in which microplastic-containing fertilizers and plastic applications were never used and found 0.34 microplastic particles per kg dry soil, with polyethylene being the most common plastic.7 In general, microplastic concentrations in the environment vary greatly. A study measuring microplastic concentrations in floodplain soils from 29 locations across Switzerland determined that the average microplastic concentration in the soils was 5 mg kg−1, but could reach as high as 55.5 mg kg−1.8 Despite increasing evidence that microplastics are ubiquitous in terrestrial environments, there is little work being done to determine their environmental and socioeconomic consequences.9
Agricultural soils can be contaminated with microplastics through a variety of routes. For instance, plastic mulch films with a thickness of ∼10 μm are used to cover agricultural fields to help regulate soil temperatures, retain soil moisture, and control the growth of weeds.6 While these plastic mulch films are present on agricultural fields, they may degrade into microplastics through abrasion and erosion.9 Earthworms have also been shown to break down microplastics into even smaller microplastics, likely through ingestion.10 UV irradiation is also known to physically and chemically degrade certain plastics into microplastics.9 Microplastics also have the potential to interact with other compounds. For instance, polyethylene microplastics have been shown to sorb polychlorinated biphenyls and polycyclic aromatic hydrocarbons.11 Potential human pathogens, such as Vibrio parahaemolyticus, have also been observed on various types of microplastics found in the North Sea and Baltic Sea.12
Biosolids application and the use of wastewater for irrigation of agricultural soils are also becoming increasingly common.13 Microplastics that enter wastewater treatment plants generally end up in the settled sludge which may then be applied to agricultural soils as a fertilizer in the form of biosolids.14,15 Nizzetto et al. estimated that 107
000 to 730
000 tonnes of microplastics make their way into agricultural soils through biosolids every year in North America and Europe.16 Similarly, several emerging chemical contaminants are often present in wastewater and end up in biosolids.13 The behaviour of these contaminants may differ in the presence of plastic particles. For example, Liu et al. found that polystyrene nanoplastics were able to enhance the transport of nonpolar and weakly polar compounds in soil, while polar compound transport was unaffected.17 Similarly in our work, we chose chemical contaminants that cover a wide range of polarities to investigate their ability to impact the soil ecosystem in the absence or presence of high-density polyethylene (HDPE) microplastics. Fu et al. published a comprehensive literature review on the adsorption of various organic pollutants to microplastics, which also includes several of the compounds examined in our study.18
Microorganisms, such as bacteria and fungi, are an important source of soil enzymes.19 Therefore, it is important to measure changes to the soil microbial community, in conjunction with soil enzyme activity, to determine whether there are any links with changes in plant growth. Soil enzyme activity has long been used as a measure of soil health due to the importance of extracellular soil enzymes in the carbon, nitrogen, sulfur, and phosphorus cycles.19 For instance, β-glucosidase (EC 3.2.1.21) is responsible for the degradation of organic matter and plant residues; in particular, it degrades cellulose, providing the soil microbial community with simple sugars.20 Likewise, chitinase (EC 3.2.1.14) (alternative name: β-N-acetylglucosaminidase) degrades chitin.21 Xylan 1,4-β-xylosidase (EC 3.2.1.37) is involved in hemicellulose degradation.22 Alkaline (EC 3.1.3.1) and acid (EC 3.1.3.2) phosphatase are responsible for the degradation of organophosphates.23 Monitoring changes in the soil microbial community composition and soil enzyme activity over time will allow us to gain a deeper understanding of how selected emerging, pharmaceutically-active contaminants can affect the soil ecosystem and strawberry plant growth in the presence and absence of HDPE microplastics.
As previously discussed, microplastics have been shown to interact with chemical compounds11 and bacteria.12 Our goal is to determine if such interactions can have an impact in an agricultural setting. Herein, we report our findings on the effects of eight common wastewater chemical contaminants on soil microbial community composition, soil enzyme activity, and strawberry plant growth in HDPE microplastic-contaminated soil in a two-year outdoor study. The chemical contaminants covered a wide range of octanol–water partition coefficients, allowing them to interact, to varying degrees, with the components of the soil ecosystem, water, and microplastics. A unique aspect of our study is that the experiments were performed outdoors, and the soil was reused in the second year after being exposed to winter conditions near Montreal, Canada. Moreover, we measured the impact of these chemical contaminants on the soil ecosystem, in the presence and absence of HDPE microplastics, to determine if the microplastics can influence any effects of the chemical contaminants. We used microplastic concentrations that are typically found in non-urban soils as these are more representative of the concentrations one may expect to find in agricultural soils. Strawberry plants were chosen because it is a high-value agricultural product. The global strawberry market had a compound annual growth rate of 5% from 2007 to 2016.24 The inherent variability of outdoor conditions (e.g., changes in temperature, light, precipitation, the presence of animals, etc.) may make it difficult to characterize certain system responses which would otherwise have been detected in a controlled laboratory environment.
2 Experimental
2.1 Production of high-density polyethylene microplastics
High-density white polyethylene sheets (48′′ × 96′′ × 1/16′′, McMaster-Carr 8619K112) were cut into strips of approximately 15 cm × 3 cm and washed with 70 v/v% ethanol and rinsed with reverse osmosis water (Type II, >1 MΩ) (Mar Cor Purification reverse osmosis water purifier). The ethanol wash was performed to remove any dust, oil, or bacteria that may have accumulated on the surface of the HDPE sheets during transportation, storage, or handling. In a single batch, three strips were placed in a 500 W glass blender (T-fal Blendforce) and the blender was filled with water until the HDPE strips were approximately half submerged. The blender was then operated in pulse mode for up to 10 min or until there was no further visible degradation of the HDPE strips. The contents of the blender were then passed through a ∼1 mm stainless steel mesh into a clean glass tray. The HDPE microplastics floated as a thin layer on the water in the glass tray. To retrieve the HDPE microplastics, a scoop made from the same HDPE sheet was used to skim the surface layer of HDPE microplastics off the water. An aluminum weighing dish was then used to scrape any HDPE microplastics off the scoop where they were left to dry in the dark at room temperature for several days until there was no more weight change so that the dry mass of the HDPE microplastics could be measured before application to soils. The HDPE microplastics were also imaged using an Olympus SZX16 microscope to visualize the variety of microplastic shapes produced by the blending process.
To determine the mass-weighted size distribution in each year of the experiment, the dried HDPE microplastics were passed through a series of 500 μm, 250 μm, and 125 μm sieves. The mass of HDPE microplastics retained by each sieve was recorded.
2.2 Preparation of contaminated soils
Soil (sand: 30%, silt: 31%, clay: 38%) was collected from an agricultural site at the Macdonald campus of McGill University (Sainte-Anne-de-Bellevue, Canada) and had a pH of 6.9 based on the pH of 8 g of soil in 40 mL of 0.01 M CaCl2 solution.25 Eight chemical contaminants known to be found in wastewater and covering a range of octanol–water partition coefficients (KOW) were examined; namely, sulfanilamide (log
KOW = −0.62),26 caffeine (log
KOW = −0.07),27 acetaminophen (log
KOW = 0.46),27 sulfamethoxazole (log
KOW = 0.89),27 carbamazepine (log
KOW = 2.45),28 ibuprofen (log
KOW = 3.97),29 gemfibrozil (log
KOW = 4.24),30 and triclosan (log
KOW = 4.76).31 To ensure the homogenization of these chemical contaminants in soil, 4.2 mL of a chemical contaminants stock solution (Table S1†) containing these mixed contaminants in acetone was added to 220 g of soil in a glass beaker. For treatments without chemical contaminants, the stock solution consisted of only acetone. Additional acetone was then added until the soil was completely submerged and was then shaken to allow for mixing of the contaminants in the suspended soil. The mixture was left to dry overnight in a fume hood after which the acetone had evaporated, resulting in 220 g of contaminated dry soil. From this batch, 40 g of chemically contaminated (or control) dry soil would be added to ∼18 kg of fresh uncontaminated soil as described in the following section.
2.3 Strawberry field experiment
Plant pots (L × W × H: 81 cm × 22 cm × 17 cm) were wrapped in aluminum with holes at the bottom for drainage. Pots were positioned in 6 rows and 5 columns in a randomized design. The treatments and controls were randomly distributed such that each column contained one pot from each treatment, and two control pots. In early June 2018, 40 g of the dry soil as prepared in section 2.2 (with or without chemical contaminants) and 0, 180, or 1800 mg of HDPE microplastics were mixed with ∼18 kg of fresh soil in each pot. The five treatments were: control (CTRL), 10 mg HDPE microplastic kg−1 soil (P10), 100 mg HDPE microplastic kg−1 soil (P100), chemical contaminants (C), and chemical contaminants + 100 mg HDPE microplastic kg−1 soil (CP100). Each column contained 2 CTRL pots, and 1 P10, P100, C, and CP100 pot for a total of 6 pots per column. Considering there were 5 columns, the experiment therefore contained 10 CTRL pots and 5 P10, P100, C, and CP100 pots for a total of 30 pots. For some treatments at certain time points, a subset of the pots was used due to occasional sample losses or resource constraints. Therefore, the actual sample size for each experiment, treatment, and time point is mentioned in their respective sections. The concentration, log
KOW, molar mass, and chemical structure of the contaminants in C and CP100 soils are listed in Table S2.† The soil was then transferred into an aluminum wrapped pot and these pots were placed ∼1.5 m apart from each other. Each pot was elevated on a wooden platform that allowed for the collection of soil leachate from the bottom of each pot into a metal container. Four strawberry bare root plants (Fragaria x ananassa cv. Seascape) (Production Lareault Inc.) were planted into each pot with two drip irrigation sources. Irrigation was applied on days with no rain, and fertilizers (Table S3†) were applied twice a week through the irrigation system. In September 2018, the end of the first year's growing season, the strawberry plants were removed from the pots. The pots were then covered with aluminum foil and left outdoors. In June 2019, the start of the second year's growing season, the pots were uncovered, and the same treatments were reapplied to the same pots containing the same soil from the first year by removing the soil from the pot into a wheelbarrow and mixing in the respective amount of HDPE microplastics and/or chemical contaminants. Strawberry bare root plants were once again planted in the same manner as the previous year. A schematic diagram of the field layout is shown in Fig. S1.† The placement of strawberry plants, drip irrigation, and soil sampling locations in each pot is shown in Fig. S2.†
2.4 Soil sampling
Soil was sampled vertically at three locations in each pot (Fig. S2†) while ensuring that ∼24 h had passed since the last rainfall or irrigation. The three soil cores (diameter: 1.5 cm, height: 12 cm) were homogenized in an aluminum tray to create a single sample (e.g., N = 1). Soil samples were then dried in an aluminum weighing tray overnight at room temperature. The soil was then passed through a 2 mm stainless steel sieve to remove any large materials such as rocks or plant roots. Soil for enzyme activity assays were used immediately. Soil for microbial community assays were stored at −80 °C until further use. Soil used for the detection of chemical contaminants was stored at −20 °C until further use. In each year, the soil was sampled just before the application (or reapplication for year 2) of treatments (i.e., day 0), and after approximately 1 week, 1 month, 2 months, and 3 months. For contaminant concentration analysis in soil samples, the soil was also sampled just after the application of the chemical contaminants at the start of each growing season to establish a starting point for C and CP100 samples relative to the CTRL.
2.5 Chemical contaminants analysis
Chemical contaminants were measured over time in two types of samples: (1) excess water that was released from holes at the bottom of the pot and subsequently captured (referred to as leachate samples), and (2) contaminants measured in soil samples.
The extraction of contaminants from leachate samples consisted of sampling directly in the field with a syringe, followed by filtration through a 0.22 μm PTFE filter (polytetrafluoroethylene, Chrom4; Thuringen, Germany) into HPLC amber glass vials (Agilent Technologies). The sample size on days 3, 8, 51, and 391 was N = 3, whereas the sample size on days 366, 369, 372, 377 and 384 was N = 5.
The extraction of contaminants from soil samples consisted of shaking 1 g of soil in 2 mL of acetonitrile for 30 min, followed by centrifugation (2240g, 5 min, 20 °C) and filtration of the supernatant through a 0.22 μm PTFE filter into HPLC glass vials. The sample size was N = 3.
Samples were analyzed using a 1290 Infinity II LC System coupled with a 6545 Q-TOF mass spectrometer (Agilent Technologies) operating once in positive (ESI+) and again in negative (ESI−) electrospray ionization modes. The liquid chromatography separation was conducted on an InfinityLab Poroshell 120 (Phenyl-Hexyl, particle size: 2.7 μm, inner diameter: 3 mm, length: 100 mm, Agilent) fitted with an InfinityLab Poroshell 120 (EC-C18, particle size: 2.7 μm, inner diameter: 3 mm, length: 100 mm, Agilent) guard column. The mobile phase (0.4 mL min−1) consisted of a mixture of water (solvent A) and acetonitrile
:
methanol (1
:
1 volume ratio, solvent B). Both solvents contained 5 mM ammonium acetate. In positive mode, 0–0.5 min: 99% A; 0.5–2 min: B increased from 1 to 50%; 2–4 min: B increased from 50 to 100%; 4–8 min: 100% B; 8–9 min: 99% A. In negative mode, 0–0.5 min: 95% A; 0.5–3 min: B increased from 5 to 100%; 3–7 min: 100% B, 7–9 min: 95% A. The injection volume was 4 μL in ESI+ mode and 10 μL in ESI− mode. The column temperature was maintained at 30 °C. Nitrogen was used as the drying gas (positive mode: 150 °C, 11 L min−1; negative mode: 175 °C, 10 L min−1). The fragmentor voltage was 125 V. Mass spectrometry data was acquired in the 50–750 mass-to-charge ratio (m/z) range in the full scan mode. Any values recorded below the instrument detection limit (IDL) were converted to IDL/2. Detection parameters of the individual chemical contaminants (e.g., m/z, retention time, etc.) are listed in Table S4.†
2.6 Soil microbial community composition
For each time point and treatment for which soil microbial community composition was measured, soil samples from three different pots were used (N = 3). Microbial genomic DNA was extracted from soil using a DNeasy PowerSoil Pro Kit (QIAGEN). Double stranded DNA concentration was then quantified using a Quant-iT PicoGreen dsDNA assay kit (Invitrogen) to ensure that the concentration of the samples met the submission requirements of Genome Quebec (Montreal, Canada). Polymerase chain reaction (PCR) was performed to amplify the extracted DNA and was sequenced using Illumina MiSeq (PE250) at Genome Quebec using the 515 F (5′-GTGCCAGCMGCCGCGGTAA-3′) and 806R (5′-GGACTACHVGGGTWTCTAAT-3′) primers to target the V4 region of the 16S rRNA. Data from Genome Quebec was provided in FASTQ format. Analysis of FASTQ data was performed using Qiime2.32 There were 39221–225826 sequences per sample with a median of 119503 sequences per sample. After denoising this was reduced to 12463–70304 sequences per sample with a median of 36989 sequences per sample. To ensure that all samples had an equal number of sequences, a sampling depth of 12462 was used (i.e., one lower than the sample with the least number of denoised sequences). The sequence of commands used in Qiime2 to process the data and calculate the weighted UniFrac distances (and its subsequent principal coordinate analysis (PCoA)), phylum-level taxonomy, and Shannon's diversity index can be found in the ESI† – Qiime2 commands.
In addition to the previously mentioned treatments, a pseudo-treatment was formed from a composite sample of the uncontaminated soils (day 0), since they were sampled before any treatments were applied. This is referred to as pre-treatment (PRE) and was only used for the microbial community composition analysis.
2.7 Soil enzyme activity measurements
Soil enzyme activities were measured according to previously reported methods,33,34 with some modifications. Dry soil (∼1.6 g) was mixed into 50 mM sodium acetate buffer (pH 6.9, ∼100 mL) in a glass jar such that the final concentration of soil in buffer was 16 g L−1. The exact volume of buffer was based on the measured mass of the dry soil to ensure a consistent concentration throughout experiments. The resulting soil slurry was placed on an orbital shaker at 240 rpm for 30 min and was then centrifuged at 4000g for 2 min. This soil extract (i.e., the supernatant) was then used for further analysis.
Soil extracts, containing the natural enzymes found in the soil, were mixed with 4-methylumbelliferone (MUB) or 4-methylumbeliferyl-linked substrates (MUB-substrates) (Table S5†). Stock solutions of 5 mM MUB and 30 mM MUB-substrate were prepared in dimethyl sulfoxide and stored at 4 °C. The stock solutions were diluted in 50 mM sodium acetate buffer (pH 6.9) (hereafter referred to simply as “buffer”) to a concentration of 10 μM MUB and 200 μM MUB-substrate which was then used in the enzyme activity assay. In a 96-well black microplate (Corning Costar), 200 μL of soil extract was mixed with 50 μL of MUB, MUB-substrate, or buffer solution. To account for the fluorescence and fluorescence quenching effects of the soil extract, similar wells containing 200 μL buffer in place of soil extract were also prepared. The prepared plate was kept in darkness at room temperature for 2 h (while the soil enzymes catalyze their respective MUB-substrates), after which 10 μL of a 1 M NaOH solution was added to each well to enhance fluorescence. The plate was then immediately analysed in a Tecan Infinite m200 Pro microplate reader at excitation/emission wavelengths of 365/450 nm. The concentration of MUB in buffer at the time of measurement (i.e., 1.92 μM) was shown to be within its linear fluorescence range (Fig. S3†). CTRL samples had a sample size of N ≤ 10, whereas P10, P100, C, and CP100 treatments had a sample size of N ≤ 5. A more detailed description of the enzyme activity calculation procedure can be found in the ESI† – calculation of enzyme activity.
2.8 Strawberry plant growth
Data was recorded from one plant in each pot (chosen from the middle). Plant parts were wrapped individually with aluminum foil and placed in a zippered storage bag. The samples were stored at −20 °C or lower. The fresh mass (marketable and non-marketable) of strawberry fruit produced by the plant over the course of the growing season was measured. The remaining plant biomass was dried, and the dry biomass was recorded. The number of leaves and flower stalks were counted every week. For the measurement of dry biomass (both years), strawberry yield (year 1) and the number of leaves (year 1) and flower stalks (year 1), CTRL samples had a sample size of N = 6, whereas P10, P100, C, and CP100 treatments had a sample size of N = 3. For the measurement of strawberry yield (year 2) and the number of leaves (year 2) and flower stalks (year 2), CTRL samples had a sample size of N = 10, whereas P10, P100, C, and CP100 treatments had a sample size of N = 5.
2.9 Statistical analysis
Data is reported as the mean ± 2 standard errors of the mean unless stated otherwise. When samples are taken from pots, a sample size of N refers to the number of pots being used unless stated otherwise. In other words, even if a single pot was sampled multiple times, it would still count as a single sample (N = 1). For each dependant variable at each time point, statistical tests were performed to determine any significant differences in the distributions between treatments at that time point. The following procedure applies to these dependant variables: dry biomass, strawberry yield, number of leaves, number of flower stalks, each enzyme's respective activity, each chemical contaminant's measured mass in leachate and measured concentration in soil, Shannon's diversity index, and phylum-level relative abundance of the soil microbial community. The Shapiro–Wilk test was performed to verify that the residuals of the data were normally distributed. If all the residuals were equal then the Shapiro–Wilk test was not performed, and the data was considered not normally distributed. Bartlett's test was performed to verify that the variance of the data from each treatment was equal. If any treatment had a variance of 0, then Bartlett's test was not performed, and it was considered that the variance among treatments were not equal. If the data was determined to have normally distributed residuals (Shapiro–Wilk test: p > 0.05) and equal variance among treatment groups (Bartlett's test: p > 0.05), then a one-way ANOVA was performed, otherwise a Kruskal–Wallis H test was performed. If any treatment group had N < 5, and the configuration (i.e., number of treatments and number of samples in each treatment) could not be found in a lookup table or the p-value would be higher than the highest p-value in the lookup table, then the result of the Kruskal–Wallis H test was deemed insignificant in that there were no significant differences in the means between treatments. If all the values in the data were equal (e.g., all values were below the limit of detection), then no significance test was performed, and a symbolic p-value of 1 was assigned to the test. A baseline for significance was α = 0.05. Since for each dependent variable, a statistical test was performed at each time point, a Holm–Bonferroni correction was applied to α such that αHB ≤ α, whereby the correction factor was equal to the number of time points. Therefore, a result of p < αHB was determined to be significant. Significant differences observed in a one-way ANOVA were followed by Tukey's HSD test. Significant differences observed in a Kruskal–Wallis H test were followed by the Mann–Whitney U test with Bonferroni correction. Tables S8–S16† contain the results to these tests. These tests were performed in Python 3.9.7 using numpy 1.21.2,35 pandas 1.3.3,36 scipy 1.7.1,37 and statsmodels 0.13.1 packages.38 Plots were made using matplotlib 3.4.2.39
To determine if the weighted UniFrac distances of the soil microbial communities' amplicon sequence variants (ASVs) (i.e., 100% similarity operational taxonomic units) between two treatments across all time points was significant, PERMANOVA was performed with q < 0.05 being considered significant. A principal coordinate analysis (PCoA) was performed on the weighted UniFrac distances as well to visualize the data.
A repository of this work's data can be found at https://doi.org/10.20383/102.0678 which includes the 16S rRNA sequences in FASTQ format, fluorescence measurements from enzyme activity experiments, and the Python code used to generate statistics and figures found in this work.
3 Results and discussion
3.1 Characterization of HDPE microplastics
Microscope images of the HDPE microplastics are shown in Fig. S4.† The mechanical weathering caused by the blender resulted in HDPE microplastics, not only of varying size, but also of vastly diverse shapes and aspect ratios, ranging from simple flat micrometer-sized fragments to long twisted microfibres. Therefore, a size distribution was measured on a mass basis by sieving the HDPE microplastics through a series of meshes and recording the mass retained by each mesh (Table 1). The initial mesh size used to separate the HDPE microplastics from the larger HDPE particles was ∼1 mm. Any plastic retained by this mesh was discarded and not used in experiments. The particle size distribution in both years was relatively similar, with decreasing mass proportion with decreasing size.
Table 1 Size distribution on a mass basis of HDPE microplastics in both years of the study. Microplastics were first passed through a ∼1 mm mesh size sieve before further use
Sieve mesh size |
2018 HDPE microplastic |
2019 HDPE microplastic |
>500 μm |
69.8% |
79.5% |
250–500 μm |
25.3% |
17.2% |
125–250 μm |
4.4% |
3.1% |
<125 μm |
0.4% |
0.2% |
Although the size distribution shows a decrease in mass concentration as microplastic size decreases, the opposite may be true when considering a particle count concentration. For instance, a 500 μm spherical microplastic would have the same mass as sixty-four 125 μm spherical microplastics. This would suggest that there could be more microplastic particles in the 125–250 μm range than there are >500 μm. A study by Wang et al. examined the distribution of several microplastic properties in agricultural soils, whereby they also observed a trend of increasing microplastic particle concentration as size decreased from 1 mm to 0.02 mm.40 They also found that polyethylene was the most prevalent microplastic found in the soil, making up 21% of microplastics found, followed closely by polyamide at 20%.40 They classified 54% of the microplastics they found as fragments and 27% as fibres.40 Overall, our results confirm that we have polydisperse microplastic-sized HDPE for our study.
3.2 Contaminant transport
The chemical contaminants covered a range of octanol–water partition coefficients (KOW) (Table S2†). The leachate and soil from the CTRL pots were examined to ascertain any background presence of these chemical contaminants. It was found that none of the chemical contaminants were observed in measurable quantities (i.e., none were above the instrument detection limit) in the CTRL soils or leachate at any time point throughout the study. Overall, the HDPE microplastics had no clear impact on the extent of these chemical contaminants leaching from the soil (Fig. 1 and S5†). Similarly, no significant differences in the concentration of any of the chemical contaminants in soil between CTRL, C, and CP100 treatments at any time point were observed (Fig. 2 and S6†). We note that due to having a low sample size (N = 3) in addition to applying a Holm–Bonferroni correction, we did not have the statistical power to distinguish the CTRL concentrations from C and CP100 soils. In year 1, most of the gemfibrozil and sulfamethoxazole was no longer present in the soil by day 35 (Fig. 2a and d). Similarly, ibuprofen was absent from the soils by day 6 (Fig. S6c†) and caffeine was almost absent by day 69 (Fig. S6b†). Conversely, triclosan and carbamazepine were still present in the soil for the duration of year 1, but did show a decline in concentration over the year (Fig. 2b and c). This indicates that some chemical contaminants may only have a short period in which they directly impact the soil, whereas others may stay in the soil for an extended period.
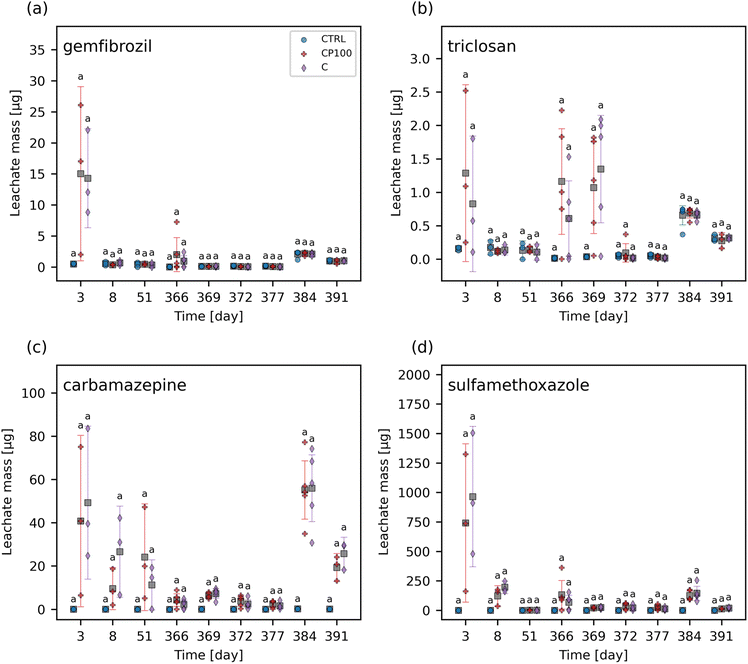 |
| Fig. 1 Leachate mass data for (a) gemfibrozil, (b) triclosan, (c) carbamazepine, and (d) sulfamethoxazole in control (CTRL), chemical contaminants + 100 mg HDPE microplastic kg−1 soil (CP100), and chemical contaminants (C) treated soils. No leachate data was collected for the intervals including day 52 – 363, 392 – 456. For each time point, treatments with the same letter have no significant difference between them. Markers represent individual samples. Grey squares represent the means. Error bars show 2 × the standard error of the mean. | |
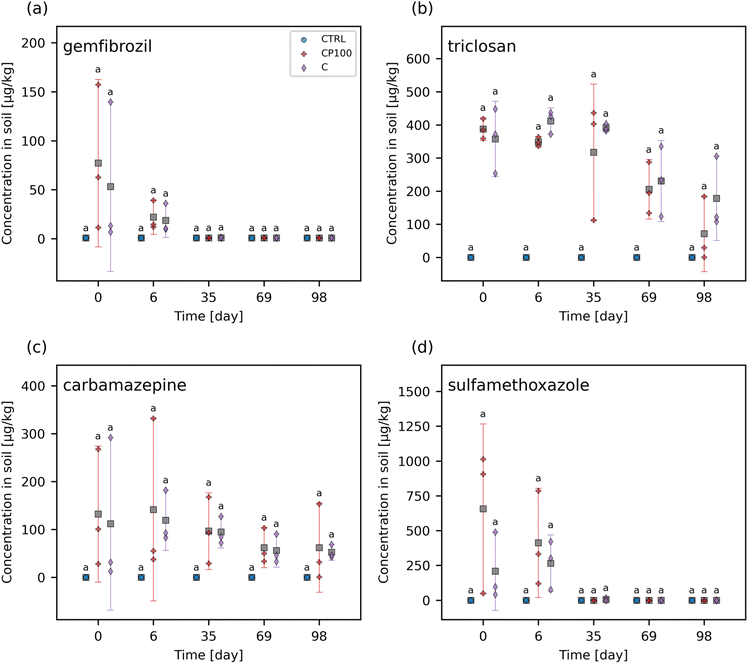 |
| Fig. 2 Concentration of (a) gemfibrozil, (b) triclosan, (c) carbamazepine, and (d) sulfamethoxazole in control (CTRL), chemical contaminants + 100 mg HDPE microplastic kg−1 soil (CP100), and chemical contaminants (C) treated soils. For each time point, treatments with the same letter have no significant difference between them. Markers represent individual samples. Grey squares represent the means. Error bars show 2 × the standard error of the mean. | |
3.3 Effect on soil microbial community composition
Soil microbial communities consist of a variety of bacteria, many of which play a vital role in the cycling of nutrients – in part by releasing extracellular enzymes – which can then be used by the plant.19 Therefore, monitoring changes to the soil microbial community composition can help explain observations regarding soil enzyme activity and, in turn, plant growth. The most abundant phyla were Acidobacteria (24.9%) and Actinobacteria (22.2%) followed by Chloroflexi (13.5%) and Proteobacteria (13.1%). Values in parentheses represent the number of reads from that phylum divided by the total reads across all samples. Phyla that represented less than 1% of the overall sequence counts across all samples were grouped together and labelled as “Other”. Acidobacteria engage in the general breakdown of soil organic matter, specifically, genes have been identified for the production of enzymes that may decompose cellulose, hemicellulose, chitin, and xylan.41 It has also been suggested that Acidobacteria can be manipulated to increase the productivity of agricultural crops.41 Actinobacteria has been shown to play a role in plant growth promotion as well.42 Interestingly, some Chloroflexi bacteria are organohalide respirers,43 while forms of Proteobacteria play a role in nitrogen fixation.44 Significant differences in the phylum-level microbial community were rare, occurring only on day 35, whereby C soils had a significantly lower relative abundance of Proteobacteria than CTRL and P10 soils, and on day 397, whereby C, CP100, and P10 soils had a significantly higher relative abundance of Actinobacteria than CTRL soils. The complete phylum-level taxonomy data is shown in Fig. 3.
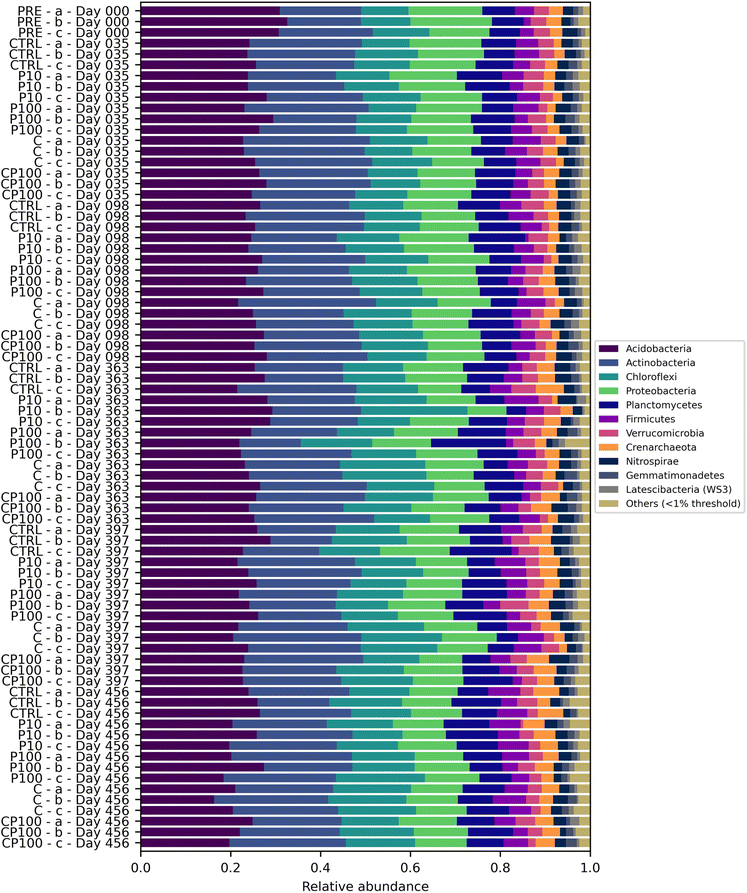 |
| Fig. 3 Soil microbial community composition based on the relative abundance of phyla found in control (CTRL), 10 mg HDPE microplastic kg−1 soil (P10), 100 mg HDPE microplastic kg−1 soil (P100), chemical contaminants + 100 mg HDPE microplastic kg−1 soil (CP100), and chemical contaminants (C) treated soils. PRE (pre-treatment) is a composite sample of the uncontaminated soils. Row labels have the following nomenclature: A-B-C, where A is the treatment, B is the sample ID, and C is the time point. | |
The alpha and beta diversity of the soils' microbial community composition based on ASVs were assessed. There were no significant differences in the Shannon's diversity index of the soil microbial community (Fig. S7†) among treatments for any of the time points measured. A PERMANOVA was performed on the pairwise weighted UniFrac distances of the soils' ASVs. The complete results can be found in Table S6.† The chemical contaminant (C) soils had a significant weighted UniFrac distance from the CTRL (q = 0.0076), P100 (q = 0.0050), PRE (q = 0.0062), and CP100 (q = 0.0329) treatments. The CP100 treatment also had a significant weighted UniFrac distance from the PRE (q = 0.0062) soils. However, the most noteworthy observation is the fact that although the CTRL-C pair showed a significant distance (q = 0.0076), no significance was observed in the CTRL-P100 pair (q = 0.421) or the CTRL-CP100 pair (q = 0.186). Moreover, the significant distance observed in the C-CP100 pair (q = 0.0329), but not the CTRL-P100 pair (q = 0.421) clearly shows that the HDPE microplastics only play a role in the composition of the microbial community in the presence of the chemical contaminants. This suggests that HDPE microplastics may play a role in minimizing the impact of chemical contaminants on the microbial community composition of soils, especially since an increase in significance (i.e., a decreasing q-value) is observed when moving from the CTRL-P100 pair (q = 0.4214) to the CTRL-CP100 pair (q = 0.186) to the significant CTRL-C pair (q = 0.0076). These results are similar to those found by Kleinteich et al. whereby polyethylene microplastics were able to reduce the impact of polycyclic aromatic hydrocarbons, such as phenanthrene and anthracene, on the bacterial community of freshwater sediments.45 A previous study by Rochman et al. found that HDPE microplastics, alongside low-density polyethylene (LDPE) and polypropylene, had particularly high sorption capacity towards polychlorinated biphenyls and polycyclic aromatic hydrocarbons, compared to polyvinyl chloride and polyethylene terephthalate microplastics in San Diego Bay, California.11 Considering that all the compounds in our study contain some form of aromatic structure (Table S2†), it is likely that a similar mechanism is at play in our study. We hypothesize that the HDPE microplastics may have adsorbed a portion of the chemical contaminants, making them less bioavailable to the soil and in turn, lessening the impact that these chemical contaminants may have had on the soil microbial community.
To visualize these results, a principal coordinate analysis (PCoA) of the weighted UniFrac distances is shown in Fig. 4. We note that the first and second principal coordinate axes combined only account for 28% of the total explained variance, and therefore the significant distances mentioned previously are not easily visible in the PCoA plot. Interestingly, Fig. S8† shows that changes in the soil microbial community composition over time are readily apparent. This helps confirm the validity of our microbial community analytical methods, since these temporal changes were easily distinguishable.
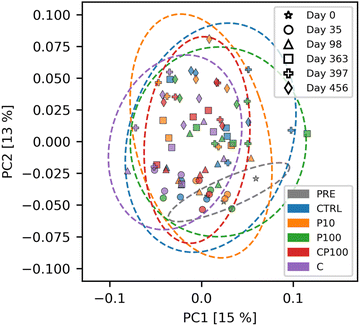 |
| Fig. 4 Principal coordinate analysis (PCoA) of the weighted UniFrac distance of the soil microbial communities' ASVs in control (CTRL), 10 mg HDPE microplastic kg−1 soil (P10), 100 mg HDPE microplastic kg−1 soil (P100), chemical contaminants + 100 mg HDPE microplastic kg−1 soil (CP100), and chemical contaminants (C) treated soils. PRE (pre-treatment) is a composite sample of the uncontaminated soils. Ellipses represent the zone where 95% of samples from a bivariate normal distribution with the same mean and covariance matrix as the respective treatment would be drawn from. Numbers in brackets indicate the amount of variance explained by that principal coordinate (PC). | |
In a study by Lin et al., LDPE with a volume-weighted mean diameter of 37 μm was applied to field soil at concentrations up to 15 g m−2 and the soil was then sampled after 287 days.46 They found that the addition of LDPE was associated with a significant decrease in the abundance of various types of microarthropods and nematodes, but had no significant impact on the overall abundance of microorganisms.46 Previously, Huang et al. exposed soil to 76 mg kg−1 LDPE microplastic films (2 mm × 2 mm × 0.01 mm) over 90 days, and observed a change in the taxonomic composition of the soil microbial community composition.47 However, they did not see any change in the Shannon's diversity index between the control soil and the soil exposed to LDPE microplastics. Similarly, Li et al. conducted a microplastic study on the sediments found in the Huangjinxia Reservoir and saw no significant difference in the Shannon's diversity index between sediment samples containing less than 400 microplastics per kg and those sediment samples containing over 600 microplastics per kg.48
The effects of chemical contaminants on soil microbial communities have also been assessed previously. Liu et al. showed that 100 mg kg−1 sulfamethoxazole can reduce the Shannon's diversity index of the soil microbial community relative to the control after 7 days of exposure, but this effect disappeared after 21 days.49 Meanwhile, Park et al. found that triclosan (10 or 50 mg kg−1 soil) had a minimal effect on the soil microbial community composition and, similar to our findings, observed that time played a greater role in soil microbial community composition changes than triclosan.50 Zhang et al. found that wastewater treated with 250 μg L−1 caffeine reduced the bacterial community diversity during a 210 days exposure in a wetland system.51
In our study, we saw no effect of any of the treatments on the Shannon's diversity index of the soil microbial community (Fig. S7†). This is in agreement with scientific literature on the impact of microplastics on the Shannon's diversity index of soil, however, the literature suggests that the presence of chemical contaminants can increase or decrease the Shannon's diversity index of the soil. Since our study consisted of an exposure involving eight chemical contaminants simultaneously, it is perhaps not so surprising that they collectively had no effect on the microbial biodiversity of the soils as their individual abilities to increase or decrease diversity may have negated each other to some extent. From the weighted UniFrac distances, it was clear that the presence of the chemical contaminants was able to significantly alter the microbial community composition of the soil relative to the control (CTRL-C pair, q = 0.0076), but was unable to significantly change it in the co-presence of 100 mg kg−1 HDPE microplastics (CTRL-CP100 pair, q = 0.1862).
3.4 Effect on soil enzyme activity
The enzyme activity of four extracellular soil enzymes were measured. Fig. 5 shows the enzyme activities for β-glucosidase, chitinase, and xylan 1,4-β-xylosidase for each treatment and time point. The enzyme activity for phosphatase (day 0 and day 69 onward) can be found in Fig. S9.† No significant difference in xylan 1,4-β-xylosidase activity between treatments were observed at any time point. The only significant differences observed were on day 6 whereby chitinase activity was significantly higher in P10 soils than CTRL and CP100 soils. Also, on day 425, β-glucosidase activity was significantly higher in CP100 and C soils when compared to CTRL and phosphatase activity was significantly higher in C and P10 soils when compared to CTRL. It is important to note that there were no significant differences in enzyme activity observed at day 0, which signifies that the soil in all the treatments had similar baseline enzyme activities, since day 0 measurements were taken just before the addition of HDPE microplastics and/or chemical contaminants.
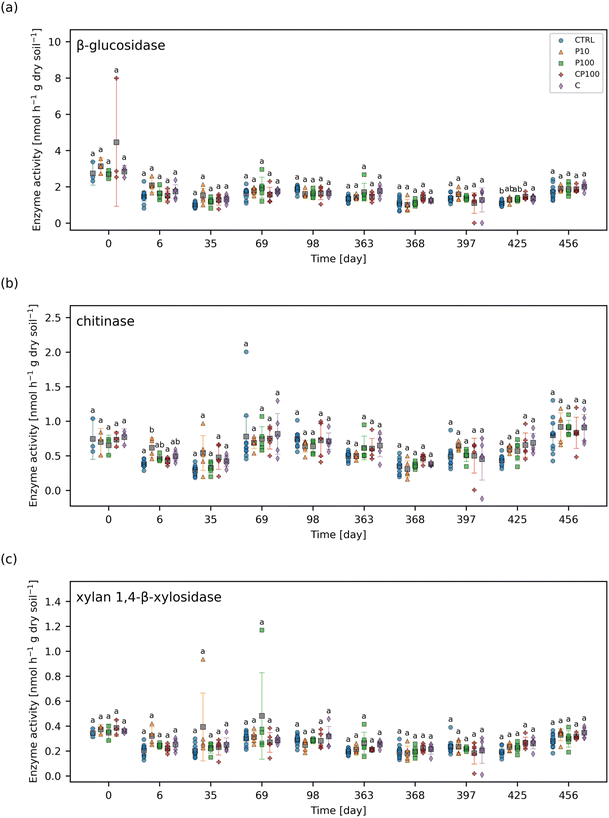 |
| Fig. 5 (a) β-Glucosidase, (b) chitinase, and (c) xylan 1,4-β-xylosidase activities over the course of two growing seasons in control (CTRL), 10 mg HDPE microplastic kg−1 soil (P10), 100 mg HDPE microplastic kg−1 soil (P100), chemical contaminants + 100 mg HDPE microplastic kg−1 soil (CP100), and chemical contaminants (C) treated soils. For each time point, treatments with the same letter have no significant difference between them. Markers represent individual samples. Grey squares represent the means. Error bars show 2 × the standard error of the mean. | |
A study by Fei et al. showed that soil containing 10
000 mg kg−1 LDPE microplastics (678 μm) for 50 days exhibited an increase in soil urease and acid phosphatase activity and a decrease in fluorescein diacetate hydrolase activity.52 Furthermore, Huang et al. treated soil with a relatively low dose of 76 mg kg−1 LDPE microplastic films (2 mm × 2 mm × 0.01 mm) over 90 days, and found that urease and catalase activity increased while invertase activity showed no significant change.47 In another study, it was shown that 70
000 mg kg−1 polypropylene microplastics (<180 μm) contaminated soil can increase fluorescein diacetate hydrolase activity, but does not affect phenyl oxidase activity after 30 days.53 These studies have shown that microplastics can have a variety of effects on the activity of different soil enzymes ranging from decreasing activity to increasing it, or having no effect altogether.
The observed effects of some of the chemical contaminants on soil enzyme activity have been previously reported in the literature. Waller and Kookana measured the effects of triclosan (1–100 mg kg−1 soil) on soil enzyme activity.54 They found that triclosan increased acid phosphatase activity in sandy soil, but not clay soil, and alkali phosphatase activity was largely unaffected in both soil types.54 They also noted that triclosan typically reduced β-glucosidase activity in sandy soil, but not clay soil, and that chitinase activity decreased significantly in clay soil when exposed to 50–100 mg triclosan kg−1 soil.54 Liu et al. also studied the effects of triclosan (0.1–50 mg kg−1 soil) and found that its initial addition to the soil inhibits phosphatase activity, but this activity slowly recovers over time.55 They suggested that this could be due to the degradation of triclosan in the soil over time.55 In a separate study, sulfamethoxazole (1–100 mg kg−1 soil) was shown to inhibit soil phosphatase activity over a 22 days exposure period.56 These studies reveal that triclosan has been shown to have variable effects on soil enzyme activity, whereas sulfamethoxazole may decrease it. In our study, we added 1.42 mg kg−1 sulfamethoxazole and 3.14 mg kg−1 triclosan along with six other chemical contaminants to soil (Table S2†), but significant effects were rare. This could be due to the outdoor exposure conditions of our experiment which could have affected the transport or degradation of these chemical contaminants in our soils. For instance, we observed greater leaching of sulfamethoxazole from soil in year 1 relative to year 2 (Fig. 1d). Heavy rain could also cause these contaminants to leach out faster than they may have otherwise under drip irrigation alone or in an indoor environmental chamber. Therefore, although the same mass of chemical contaminants was applied to the soil in each year, the amount present at each time point was likely variable.
3.5 Effect on strawberry plant growth
The total yield of fresh strawberries, the dry biomass of the remaining plant (i.e., without any strawberry fruit remaining), the number of leaves, and the number of flower stalks at the end of each year was measured (Fig. 6). No significant differences were observed in any of these endpoints between any of the treatments at any time point suggesting that any impact of the HDPE microplastics and/or chemical contaminants on these endpoints was smaller than the general variance that occurs from growing strawberries under outdoor conditions. This variance can be caused by several factors such as the interaction of the strawberry plants with birds, land animals, and insects, as well as variations in the weather, precipitation, and temperature which would not be present in a climate-controlled lab environment.
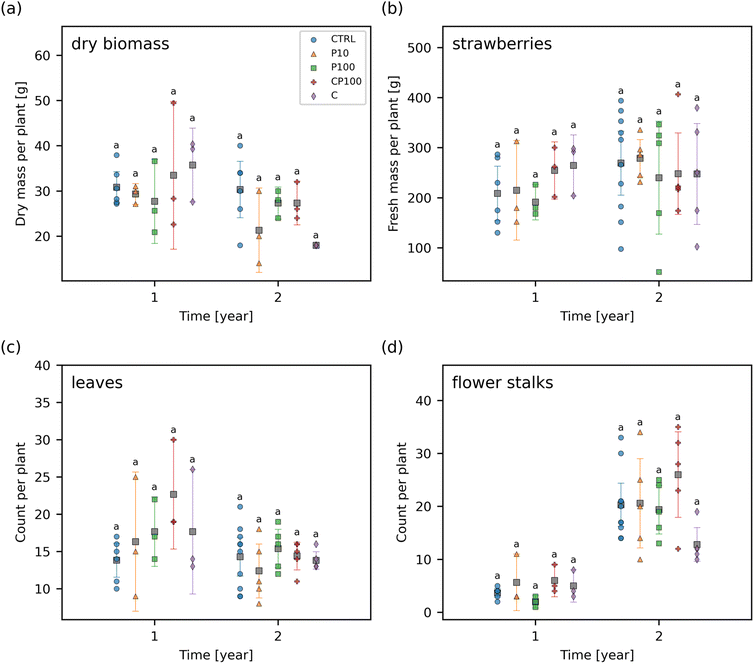 |
| Fig. 6 (a) Dry biomass of the remaining plant without any strawberry fruit, (b) total yield of fresh strawberries, (c) number of leaves, and (d) number of flower stalks in control (CTRL), 10 mg HDPE microplastic kg−1 soil (P10), 100 mg HDPE microplastic kg−1 soil (P100), chemical contaminants + 100 mg HDPE microplastic kg−1 soil (CP100), and chemical contaminants (C) treated soils. For each time point, treatments with the same letter have no significant difference between them. Markers represent individual samples. Grey squares represent the means. Error bars show 2 × the standard error of the mean. | |
The effect of microplastics on other plant species has previously been studied. For instance, our results are similar to those of a previous study by Meng et al. whereby soil containing 250–1000 μm LDPE microplastics at 25
000 mg kg−1 dry soil had no significant effect on the shoot, root, or fruit biomass of Phaseolus vulgaris L. in an outdoor experiment over approximately 4 months.57 Conversely, Pignattelli et al. examined the effect of 184 mg kg−1 polyethylene microplastics (<125 μm) on garden cress (Lepidium sativum) over 21 days.58 They found that soil containing polyethylene microplastics had a significantly higher germination inhibition of 7.1% when compared to 0% in the control, and reduced shoot biomass by 38% relative to the control soil; however, there was no significant effect on the shoot height or the number of leaves.58 Qi et al. examined the effect of LDPE microplastics (<1 mm) on wheat (Triticum aestivum) growth.59 Over a 4 month period, there was generally little or no significant difference between wheat grown in soil contaminated with 10
000 mg kg−1 LDPE microplastics and the control soil over a variety of factors including: plant height, number of tillers, number of fruits, shoot and root biomass, leaf area, number of leaves, stem diameter, and relative chlorophyll content.59 Likewise, our results show that a similar phenomenon was observed in strawberry plants with HDPE microplastics, in an outdoor environment over a similar time frame. Moreover, our study was done at a lower exposure concentration than these previous studies.
When considering the chemical contaminants added to the soil, triclosan has previously been shown to have no adverse effects on maize, soybean, and spring wheat.60 Barbera et al. exposed rice (Oryza sativa) to a mixture of ibuprofen and caffeine which resulted in a 51% increase in rice grain yield.61 Conversely, sulfamethoxazole (8.0 μM in Murashige and Skoog solid medium) has been shown to decrease the root length and shoot biomass of Arabidopsis thaliana by 95% and 90%, respectively.62 In our work, we added 5.6 μmol sulfamethoxazole per kg fresh soil along with triclosan, ibuprofen, caffeine, and other chemical contaminants at various concentrations (Table S2†). The combination of these growth enhancing and growth inhibiting effects as well as the nature of the experiment being outdoors in a highly variable environment is consistent with our results that indicate that there is no significant effect of the chemical contaminants on the dry biomass, strawberry yield, number of leaves, and number of flower stalks (Fig. 6).
4 Conclusions
The effects of emerging contaminants in the presence and absence of HDPE microplastics on soil microbial community composition, soil enzyme activity, and strawberry plant growth were measured. It was found that the weighted UniFrac distance between the CTRL-C soils were significant but were not significant for the CTRL-CP100 and CTRL-P100 pairs. This suggests that the presence of HDPE may have mitigated the ability of the chemical contaminants to alter the soil microbial community composition. We hypothesize that due to the aromatic nature of the chemical contaminants, the adsorption of the chemical contaminants to the HDPE microplastics reduced their bioavailability, thereby lessening their impact on the soil microbial community. This is further supported by earlier work.11 Moreover, significant differences in the soil enzyme activity, and the phylum-level soil microbial community composition among treatments were rare. No significant differences between treatments in the strawberry yield, plant dry biomass, the number of leaves or flower stalks, and the Shannon's diversity index were observed at any time point.
Other researchers have also observed little or no effect of microplastics on Tripneustes gratilla larvae survival;63Lemna minor growth or photosynthetic efficiency, as well as the mortality or mobility of Gammarus duebeni that ingested the microplastic-contaminated L. minor;64Echinogammarus marinus growth or food consumption;65 and the foraging activity of Acanthurus triostegus.66 While there were little to no significant differences among treatments in many of the endpoints studied in this work, we believe there are several aspects that make this study of interest. For instance, the study was designed to mimic real world conditions as closely as possible without having to contaminate an open field. This was done by using agricultural soil in pots outdoors over a long two-year period with environmentally relevant concentrations of microplastics and chemical contaminants. We also acknowledge that some endpoints were measured with a small sample size and may have contributed to the lack of observed effect. It is also important to share “no negative effects” data in an effort to contribute to an unbiased microplastic literature and to prevent the duplication of similar resource-intensive experiments by other researchers.67 We believe the data presented here is particularly important to the agriculture industry due to the widespread usage of HDPE and other plastics in agriculture (e.g., irrigation parts, plastic mulch, etc.). These results should help in understanding the pros and cons of plastic use in agricultural soils.
Conflicts of interest
The authors declare that they have no known competing financial interests or personal relationships that could have appeared to influence the work reported in this paper.
Acknowledgements
The authors acknowledge funding from the Natural Sciences and Engineering Research Council of Canada (Discovery and Strategic Project programs), the Canada Research Chairs Program, the Canada Foundation for Innovation (grants to NT, SB, SG, and VG), the Killam Research Fellowship, and the Fonds de recherche du Québec – Nature et technologies (grant no. 286120). We appreciate the support of Environment and Climate Change Canada, Health Canada, the Canadian Food Inspection Agency, the Mass Spectrometry Platform at the Macdonald Campus of McGill University, and Vive Crop Protection. S. Chahal acknowledges NSERC for a PGS D scholarship, McGill University for a MEDA fellowship, the support of the EUL fund in the Department of Chemical Engineering, and EcotoQ. V. B. was supported in part by the McGill Engineering Doctoral Award. The authors would like to thank S. Fan, P. Gopal, I. Hader, M. Bleho, L. Caralampides, and the team at the Macdonald Campus Horticulture Research Centre for their assistance during the field experiment.
References
- D.- K. A. Barnes, F. Galgani, R. C. Thompson and M. Barlaz, Accumulation and fragmentation of plastic debris in global environments, Philos. Trans. R. Soc., B, 2009, 364, 1985–1998 CrossRef CAS PubMed.
- A. A. de Souza Machado, W. Kloas, C. Zarfl, S. Hempel and M. C. Rillig, Microplastics as an emerging threat to terrestrial ecosystems, Global Change Biol., 2018, 24, 1405–1416 CrossRef PubMed.
- A. A. Horton, A. Walton, D. J. Spurgeon, E. Lahive and C. Svendsen, Microplastics in freshwater and terrestrial environments: Evaluating the current understanding to identify the knowledge gaps and future research priorities, Sci. Total Environ., 2017, 586, 127–141 CrossRef CAS PubMed.
- S. Allen, D. Allen, V. R. Phoenix, G. Le Roux, P. Durántez Jiménez, A. Simonneau, S. Binet and D. Galop, Atmospheric transport and deposition of microplastics in a remote mountain catchment, Nat. Geosci., 2019, 12, 339–344 CrossRef CAS.
- S. Fuller and A. Gautam, A Procedure for Measuring Microplastics using Pressurized Fluid Extraction, Environ. Sci. Technol., 2016, 50, 5774–5780 CrossRef CAS PubMed.
- E.-L. Ng, E. Huerta Lwanga, S. M. Eldridge, P. Johnston, H.-W. Hu, V. Geissen and D. Chen, An overview of microplastic and nanoplastic pollution in agroecosystems, Sci. Total Environ., 2018, 627, 1377–1388 CrossRef CAS PubMed.
- S. Piehl, A. Leibner, M. G. J. Löder, R. Dris, C. Bogner and C. Laforsch, Identification and quantification of macro- and microplastics on an agricultural farmland, Sci. Rep., 2018, 8, 17950 CrossRef CAS PubMed.
- M. Scheurer and M. Bigalke, Microplastics in Swiss Floodplain Soils, Environ. Sci. Technol., 2018, 52, 3591–3598 CrossRef CAS PubMed.
-
L. Mai, L.-J. Bao, C. S. Wong and E. Y. Zeng, in Microplastic Contamination in Aquatic Environments, ed. E. Y. Zeng, Elsevier, 2018, pp. 365–378, DOI:10.1016/B978-0-12-813747-5.00012-6.
- E. Huerta Lwanga, H. Gertsen, H. Gooren, P. Peters, T. Salánki, M. van der Ploeg, E. Besseling, A. A. Koelmans and V. Geissen, Incorporation of microplastics from litter into burrows of Lumbricus terrestris, Environ. Pollut., 2017, 220, 523–531 CrossRef CAS.
- C. M. Rochman, E. Hoh, B. T. Hentschel and S. Kaye, Long-Term Field Measurement of Sorption of Organic Contaminants to Five Types of Plastic Pellets: Implications for Plastic Marine Debris, Environ. Sci. Technol., 2013, 47, 1646–1654 CAS.
- I. V. Kirstein, S. Kirmizi, A. Wichels, A. Garin-Fernandez, R. Erler, M. Löder and G. Gerdts, Dangerous hitchhikers? Evidence for potentially pathogenic Vibrio spp. on microplastic particles, Mar. Environ. Res., 2016, 120, 1–8 CrossRef CAS PubMed.
- D. P. Mohapatra, M. Cledón, S. K. Brar and R. Y. Surampalli, Application of Wastewater and Biosolids in Soil: Occurrence and Fate of Emerging Contaminants, Water, Air, Soil Pollut., 2016, 227, 77 CrossRef.
- S. A. Carr, J. Liu and A. G. Tesoro, Transport and fate of microplastic particles in wastewater treatment plants, Water Res., 2016, 91, 174–182 CrossRef CAS.
- F. Corradini, P. Meza, R. Eguiluz, F. Casado, E. Huerta-Lwanga and V. Geissen, Evidence of microplastic accumulation in agricultural soils from sewage sludge disposal, Sci. Total Environ., 2019, 671, 411–420 CrossRef CAS PubMed.
- L. Nizzetto, M. Futter and S. Langaas, Are Agricultural Soils Dumps for Microplastics of Urban Origin?, Environ. Sci. Technol., 2016, 50, 10777–10779 CrossRef CAS PubMed.
- J. Liu, Y. Ma, D. Zhu, T. Xia, Y. Qi, Y. Yao, X. Guo, R. Ji and W. Chen, Polystyrene Nanoplastics-Enhanced Contaminant Transport: Role of Irreversible Adsorption in Glassy Polymeric Domain, Environ. Sci. Technol., 2018, 52, 2677–2685 CrossRef CAS PubMed.
- L. Fu, J. Li, G. Wang, Y. Luan and W. Dai, Adsorption behavior of organic pollutants on microplastics, Ecotoxicol. Environ. Saf., 2021, 217, 112207 CrossRef CAS PubMed.
- I. Alkorta, A. Aizpurua, P. Riga, I. Albizu, I. Amézaga and C. Garbisu, Soil Enzyme Activities as Biological Indicators of Soil Health, Rev. Environ. Health, 2003, 18, 65–73 Search PubMed.
- D. E. Stott, S. S. Andrews, M. A. Liebig, B. J. Wienhold and D. L. Karlen, Evaluation of β-Glucosidase Activity as a Soil Quality Indicator for the Soil Management Assessment Framework, Soil Sci. Soc. Am. J., 2010, 74, 107–119 CrossRef CAS.
- R. L. Sinsabaugh, C. L. Lauber, M. N. Weintraub, B. Ahmed, S. D. Allison, C. Crenshaw, A. R. Contosta, D. Cusack, S. Frey, M. E. Gallo, T. B. Gartner, S. E. Hobbie, K. Holland, B. L. Keeler, J. S. Powers, M. Stursova, C. Takacs-Vesbach, M. P. Waldrop, M. D. Wallenstein, D. R. Zak and L. H. Zeglin, Stoichiometry of soil enzyme activity at global scale, Ecol. Lett., 2008, 11, 1252–1264 CrossRef PubMed.
- P. C. Selmants, S. C. Hart, S. I. Boyle and J. M. Stark, Red alder (Alnus rubra) alters community-level soil microbial function in conifer forests of the Pacific Northwest, USA, Soil Biol. Biochem., 2005, 37, 1860–1868 CrossRef CAS.
- H. Rodríguez and R. Fraga, Phosphate solubilizing bacteria and their role in plant growth promotion, Biotechnol. Adv., 1999, 17, 319–339 CrossRef.
-
IndexBox, World: Strawberries – Market Report. Analysis and Forecast to 2025, 2017 Search PubMed.
- R. K. Schofield and A. W. Taylor, The Measurement of Soil pH, Soil Sci. Soc. Am. J., 1955, 19, 164–167 CrossRef CAS.
-
C. Hansch, A. Leo and D. Hoekman, Exploring QSAR: Hydrophobic, Electronic, and Steric Constants, American Chemical Society, Washington, DC, 1995 Search PubMed.
- S. A. Snyder, P. Westerhoff, Y. Yoon and D. L. Sedlak, Pharmaceuticals, Personal Care Products, and Endocrine Disruptors in Water: Implications for the Water Industry, Environ. Eng. Sci., 2003, 20, 449–469 CrossRef CAS.
- A. Dal Pozzo, G. Donzelli, L. Rodriquez and A. Tajana,
In vitro model for the evaluation of drug distribution and plasma protein-binding relationships, Int. J. Pharm., 1989, 50, 97–101 CrossRef CAS.
- A. Avdeef, pH-Metric log P. II: Refinement of Partition Coefficients and Ionization Constants of Multiprotic Substances, J. Pharm. Sci., 1993, 82, 183–190 CrossRef CAS PubMed.
- Z. Krascsenits, E. Hiller and M. Bartal, Distribution of four human pharmaceuticals, carbamazepine, diclofenac, gemfibrozil, and ibuprofen between sediment and water, J. Hydrol. Hydromech., 2008, 56, 237–246 CAS.
- M. G. Pintado-Herrera, P. A. Lara-Martín, E. González-Mazo and I. J. Allan, Determination of silicone rubber and low-density polyethylene diffusion and polymer/water partition coefficients for emerging contaminants, Environ. Toxicol. Chem., 2016, 35, 2162–2172 CrossRef CAS PubMed.
- E. Bolyen, J. R. Rideout, M. R. Dillon, N. A. Bokulich, C. C. Abnet, G. A. Al-Ghalith, H. Alexander, E. J. Alm, M. Arumugam, F. Asnicar, Y. Bai, J. E. Bisanz, K. Bittinger, A. Brejnrod, C. J. Brislawn, C. T. Brown, B. J. Callahan, A. M. Caraballo-Rodríguez, J. Chase, E. K. Cope, R. Da Silva, C. Diener, P. C. Dorrestein, G. M. Douglas, D. M. Durall, C. Duvallet, C. F. Edwardson, M. Ernst, M. Estaki, J. Fouquier, J. M. Gauglitz, S. M. Gibbons, D. L. Gibson, A. Gonzalez, K. Gorlick, J. Guo, B. Hillmann, S. Holmes, H. Holste, C. Huttenhower, G. A. Huttley, S. Janssen, A. K. Jarmusch, L. Jiang, B. D. Kaehler, K. B. Kang, C. R. Keefe, P. Keim, S. T. Kelley, D. Knights, I. Koester, T. Kosciolek, J. Kreps, M. G. I. Langille, J. Lee, R. Ley, Y.-X. Liu, E. Loftfield, C. Lozupone, M. Maher, C. Marotz, B. D. Martin, D. McDonald, L. J. McIver, A. V. Melnik, J. L. Metcalf, S. C. Morgan, J. T. Morton, A. T. Naimey, J. A. Navas-Molina, L. F. Nothias, S. B. Orchanian, T. Pearson, S. L. Peoples, D. Petras, M. L. Preuss, E. Pruesse, L. B. Rasmussen, A. Rivers, M. S. Robeson, P. Rosenthal, N. Segata, M. Shaffer, A. Shiffer, R. Sinha, S. J. Song, J. R. Spear, A. D. Swafford, L. R. Thompson, P. J. Torres, P. Trinh, A. Tripathi, P. J. Turnbaugh, S. Ul-Hasan, J. J. J. van der Hooft, F. Vargas, Y. Vázquez-Baeza, E. Vogtmann, M. von Hippel, W. Walters, Y. Wan, M. Wang, J. Warren, K. C. Weber, C. H. D. Williamson, A. D. Willis, Z. Z. Xu, J. R. Zaneveld, Y. Zhang, Q. Zhu, R. Knight and J. G. Caporaso, Reproducible, interactive, scalable and extensible microbiome data science using QIIME 2, Nat. Biotechnol., 2019, 37, 852–857 CrossRef CAS PubMed.
- B. Asadishad, S. Chahal, A. Akbari, V. Cianciarelli, M. Azodi, S. Ghoshal and N. Tufenkji, Amendment of Agricultural Soil with Metal Nanoparticles: Effects on Soil Enzyme Activity and Microbial Community Composition, Environ. Sci. Technol., 2018, 52, 1908–1918 CrossRef CAS.
- C. Peyrot, K. J. Wilkinson, M. Desrosiers and S. Sauvé, Effects of silver nanoparticles on soil enzyme activities with and without added organic matter, Environ. Toxicol. Chem., 2014, 33, 115–125 CrossRef CAS PubMed.
- C. R. Harris, K. J. Millman, S. J. van der Walt, R. Gommers, P. Virtanen, D. Cournapeau, E. Wieser, J. Taylor, S. Berg, N. J. Smith, R. Kern, M. Picus, S. Hoyer, M. H. van Kerkwijk, M. Brett, A. Haldane, J. F. del Río, M. Wiebe, P. Peterson, P. Gérard-Marchant, K. Sheppard, T. Reddy, W. Weckesser, H. Abbasi, C. Gohlke and T. E. Oliphant, Array programming with NumPy, Nature, 2020, 585, 357–362 CrossRef CAS PubMed.
-
W. McKinney, Data Structures for Statistical Computing in Python, Austin, Texas, 2010 Search PubMed.
- P. Virtanen, R. Gommers, T. E. Oliphant, M. Haberland, T. Reddy, D. Cournapeau, E. Burovski, P. Peterson, W. Weckesser, J. Bright, S. J. van der Walt, M. Brett, J. Wilson, K. J. Millman, N. Mayorov, A. R. J. Nelson, E. Jones, R. Kern, E. Larson, C. J. Carey, İ. Polat, Y. Feng, E. W. Moore, J. VanderPlas, D. Laxalde, J. Perktold, R. Cimrman, I. Henriksen, E. A. Quintero, C. R. Harris, A. M. Archibald, A. H. Ribeiro, F. Pedregosa, P. van Mulbregt, A. Vijaykumar, A. P. Bardelli, A. Rothberg, A. Hilboll, A. Kloeckner, A. Scopatz, A. Lee, A. Rokem, C. N. Woods, C. Fulton, C. Masson, C. Häggström, C. Fitzgerald, D. A. Nicholson, D. R. Hagen, D. V. Pasechnik, E. Olivetti, E. Martin, E. Wieser, F. Silva, F. Lenders, F. Wilhelm, G. Young, G. A. Price, G.-L. Ingold, G. E. Allen, G. R. Lee, H. Audren, I. Probst, J. P. Dietrich, J. Silterra, J. T. Webber, J. Slavič, J. Nothman, J. Buchner, J. Kulick, J. L. Schönberger, J. V. de Miranda Cardoso, J. Reimer, J. Harrington, J. L. C. Rodríguez, J. Nunez-Iglesias, J. Kuczynski, K. Tritz, M. Thoma, M. Newville, M. Kümmerer, M. Bolingbroke, M. Tartre, M. Pak, N. J. Smith, N. Nowaczyk, N. Shebanov, O. Pavlyk, P. A. Brodtkorb, P. Lee, R. T. McGibbon, R. Feldbauer, S. Lewis, S. Tygier, S. Sievert, S. Vigna, S. Peterson, S. More, T. Pudlik, T. Oshima, T. J. Pingel, T. P. Robitaille, T. Spura, T. R. Jones, T. Cera, T. Leslie, T. Zito, T. Krauss, U. Upadhyay, Y. O. Halchenko, Y. Vázquez-Baeza and C. SciPy, SciPy 1.0: fundamental algorithms for scientific computing in Python, Nat. Methods, 2020, 17, 261–272 CrossRef CAS PubMed.
-
S. Seabold and J. Perktold, Statsmodels: Econometric and Statistical Modeling with Python, Austin, TX, 2010 Search PubMed.
- J. D. Hunter, Matplotlib: A 2D Graphics Environment, Comput. Sci. Eng., 2007, 9, 90–95 Search PubMed.
- J. Wang, J. Li, S. Liu, H. Li, X. Chen, C. Peng, P. Zhang and X. Liu, Distinct microplastic distributions in soils of different land-use types: A case study of Chinese farmlands, Environ. Pollut., 2021, 269, 116199 CrossRef CAS PubMed.
- S. Kalam, A. Basu, I. Ahmad, R. Z. Sayyed, H. A. El-Enshasy, D. J. Dailin and N. L. Suriani, Recent Understanding of Soil Acidobacteria and Their Ecological Significance: A Critical Review, Front. Microbiol., 2020, 11, 580024 CrossRef PubMed.
-
S. N. Hazarika and D. Thakur, in Beneficial Microbes in Agro-Ecology, ed. N. Amaresan, M. Senthil Kumar, K. Annapurna, K. Kumar and A. Sankaranarayanan, Academic Press, 2020, pp. 443–476, DOI: DOI:10.1016/B978-0-12-823414-3.00021-6.
- M. J. Krzmarzick, B. B. Crary, J. J. Harding, O. O. Oyerinde, A. C. Leri, S. C. B. Myneni and P. J. Novak, Natural Niche for Organohalide-Respiring Chloroflexi, Appl. Environ. Microbiol., 2012, 78, 393–401 CrossRef CAS PubMed.
-
K. Kersters, P. De Vos, M. Gillis, J. Swings, P. Vandamme and E. Stackebrandt, in The Prokaryotes: Volume 5: Proteobacteria: Alpha and Beta Subclasses, ed. M. Dworkin, S. Falkow, E. Rosenberg, K.-H. Schleifer and E. Stackebrandt, Springer New York, New York, NY, 2006, pp. 3–37, DOI:10.1007/0-387-30745-1_1.
- J. Kleinteich, S. Seidensticker, N. Marggrander and C. Zarfl, Microplastics Reduce Short-Term Effects of Environmental Contaminants. Part II: Polyethylene Particles Decrease the Effect of Polycyclic Aromatic Hydrocarbons on Microorganisms, Int. J. Environ. Res. Public Health, 2018, 15, 287 CrossRef.
- D. Lin, G. Yang, P. Dou, S. Qian, L. Zhao, Y. Yang and N. Fanin, Microplastics negatively affect soil fauna but stimulate microbial activity: insights from a field-based microplastic addition experiment, Proc. R. Soc. B, 2020, 287, 20201268 CrossRef CAS PubMed.
- Y. Huang, Y. Zhao, J. Wang, M. Zhang, W. Jia and X. Qin, LDPE microplastic films alter microbial community composition and enzymatic activities in soil, Environ. Pollut., 2019, 254, 112983 CrossRef CAS PubMed.
- C. Li, Y. Gan, J. Dong, J. Fang, H. Chen, Q. Quan and J. Liu, Impact of microplastics on microbial community in sediments of the Huangjinxia Reservoir—water source of a water diversion project in western China, Chemosphere, 2020, 253, 126740 CrossRef CAS PubMed.
- F. Liu, J. Wu, G.-G. Ying, Z. Luo and H. Feng, Changes in functional diversity of soil microbial community with addition of antibiotics sulfamethoxazole and chlortetracycline, Appl. Microbiol. Biotechnol., 2012, 95, 1615–1623 CrossRef CAS PubMed.
- I. Park, N. Zhang, T. A. Ogunyoku, T. M. Young and K. M. Scow, Effects of Triclosan and Biosolids on Microbial Community Composition in an Agricultural Soil, Water Environ. Res., 2013, 85, 2237–2242 CrossRef CAS PubMed.
- D. Zhang, J. Luo, Z. M. P. Lee, R. M. Gersberg, Y. Liu, S. K. Tan and W. J. Ng, Characterization of microbial communities in wetland mesocosms receiving caffeine-enriched wastewater, Environ. Sci. Pollut. Res., 2016, 23, 14526–14539 CrossRef CAS PubMed.
- Y. Fei, S. Huang, H. Zhang, Y. Tong, D. Wen, X. Xia, H. Wang, Y. Luo and D. Barceló, Response of soil enzyme activities and bacterial communities to the accumulation of microplastics in an acid cropped soil, Sci. Total Environ., 2020, 707, 135634 CrossRef CAS PubMed.
- H. Liu, X. Yang, G. Liu, C. Liang, S. Xue, H. Chen, C. J. Ritsema and V. Geissen, Response of soil dissolved organic matter to microplastic addition in Chinese loess soil, Chemosphere, 2017, 185, 907–917 CrossRef CAS PubMed.
- N. J. Waller and R. S. Kookana, Effect of triclosan on microbial activity in Australian soils, Environ. Toxicol. Chem., 2009, 28, 65–70 CrossRef CAS PubMed.
- F. Liu, G.-G. Ying, L.-H. Yang and Q.-X. Zhou, Terrestrial ecotoxicological effects of the antimicrobial agent triclosan, Ecotoxicol. Environ. Saf., 2009, 72, 86–92 CrossRef CAS.
- F. Liu, G.-G. Ying, R. Tao, J.-L. Zhao, J.-F. Yang and L.-F. Zhao, Effects of six selected antibiotics on plant growth and soil microbial and enzymatic activities, Environ. Pollut., 2009, 157, 1636–1642 CrossRef CAS PubMed.
- F. Meng, X. Yang, M. Riksen, M. Xu and V. Geissen, Response of common bean (Phaseolus vulgaris L.) growth to soil contaminated with microplastics, Sci. Total Environ., 2021, 755, 142516 CrossRef CAS PubMed.
- S. Pignattelli, A. Broccoli and M. Renzi, Physiological responses of garden cress (L. sativum) to different types of microplastics, Sci. Total Environ., 2020, 727, 138609 CrossRef CAS PubMed.
- Y. Qi, X. Yang, A. M. Pelaez, E. Huerta Lwanga, N. Beriot, H. Gertsen, P. Garbeva and V. Geissen, Macro- and micro- plastics in soil-plant system: Effects of plastic mulch film residues on wheat (Triticum aestivum) growth, Sci. Total Environ., 2018, 645, 1048–1056 CrossRef CAS PubMed.
- R. S. Shahmohamadloo, L. Lissemore, R. S. Prosser and P. K. Sibley, Evaluating the effects of triclosan on 3 field crops grown in 4 formulations of biosolids, Environ. Toxicol. Chem., 2017, 36, 1896–1908 CrossRef CAS PubMed.
- A. C. Barbera, G. Leonardi, M. Ferrante, P. Zuccarello and C. Maucieri, Effects of pharmaceuticals (Caffeine and Ibuprofen) and AMF inoculation on the growth and yield of Oryza sativa L, Agric. Water Manag., 2020, 232, 106005 CrossRef.
- D. Xu, H. Pan, J. Yao, Y. Feng, P. Wu and K. Shao, Stress responses and biological residues of sulfanilamide antibiotics in Arabidopsis thaliana, Ecotoxicol. Environ. Saf., 2020, 199, 110727 CrossRef CAS PubMed.
- K. L. Kaposi, B. Mos, B. P. Kelaher and S. A. Dworjanyn, Ingestion of Microplastic Has Limited Impact on a Marine Larva, Environ. Sci. Technol., 2014, 48, 1638–1645 CrossRef CAS.
- A. Mateos-Cárdenas, D. T. Scott, G. Seitmaganbetova, v. P. Frank N, O. H. John and J. Marcel A, Polyethylene microplastics adhere to Lemna minor (L.), yet have no effects on plant growth or feeding by Gammarus duebeni (Lillj.), Sci. Total Environ., 2019, 689, 413–421 CrossRef PubMed.
- S. Bruck and A. T. Ford, Chronic ingestion of polystyrene microparticles in low doses has no effect on food consumption and growth to the intertidal amphipod Echinogammarus marinus?, Environ. Pollut., 2018, 233, 1125–1130 CrossRef CAS PubMed.
- H. Jacob, A. Gilson, C. Lanctôt, M. Besson, M. Metian and D. Lecchini, No Effect of Polystyrene Microplastics on Foraging Activity and Survival in a Post-larvae Coral-Reef Fish, Acanthurus triostegus, Bull. Environ. Contam. Toxicol., 2019, 102, 457–461 CrossRef CAS PubMed.
- I. Lynch and R. Klaper, Telling the important stories of “no adverse effect” nanomaterials data, Environ. Sci.: Nano, 2021, 8, 1496–1499 RSC.
|
This journal is © The Royal Society of Chemistry 2023 |