Maternal exposure to polystyrene nanoplastics impacts developmental milestones and brain structure in mouse offspring†
Received
24th September 2022
, Accepted 14th February 2023
First published on 15th February 2023
Abstract
The presence of microplastics and nanoplastics (NPs) has recently been reported in human blood and tissues, raising concerns about their potential impacts on human health and fetal development. In this study, we investigated the effects of maternal exposure to NPs on the timing of developmental milestones and on brain structure using experimental mice. Healthy, pregnant CD-1 dams were given 106 ng L−1 of 50 nm polystyrene NPs in drinking water throughout gestation and lactation and the postnatal behavior and neuroanatomy of the offspring were studied. We found that NPs exposure resulted in earlier time to eye opening in male offspring but not in females (p = 0.01). 3D high-resolution ex vivo magnetic resonance imaging (MRI) revealed that offspring exposed to NPs had focal differences compared to controls in multiple brain structures that are involved in motor function, learning and memory, and physiological functions including the motor cortex, hippocampus, hypothalamus, medulla, and olfactory bulb. Several of these MRI-detectable neuroanatomical changes were dependent on sex. Our study demonstrates that maternal exposure to NPs results in abnormal postnatal brain development in the mouse. Further investigations are needed to determine the mechanisms whereby NPs exposure during fetal development may adversely affect dimensions of brain function in a sex-dependent manner.
Environmental significance
The worldwide use of plastics has resulted in the vast accumulation of micro- and nanoplastics in air, soil and, water. These plastics can be ingested by humans and potentially accumulate in tissues and organs. Microplastics were recently found in the placenta, raising the concern that plastics may have an impact on pregnancy and fetal development. This work used experimental mice, behavioural testing, and magnetic resonance imaging to determine how maternal exposure to nanoplastics effects brain development. We found nanoplastics exposure resulted in earlier time to eye opening in male offspring, but not in females, and in focal anatomical changes in the brain that were dependent on sex. These results motivate the development of regulations to minimize potentially harmful human exposure to plastics, particularly during pregnancy and early neonatal development.
|
1. Introduction
Plastics have become ubiquitous in modern life and can be found in common items such as food packaging, clothing, and furniture. It is estimated that almost 80% of consumer plastics are discarded in landfills or released to the natural environment.1 Not only can larger plastic fragments and fibers cause harm in the ocean and land environment, but they can further degrade over time, forming smaller particles termed microplastics and nanoplastics (NPs, particles with diameter <1 μm) that may become airborne and be therefore either inhaled or ingested to become potentially harmful to human health.2,3 Following inhalation, NPs are small enough to translocate from the lungs into the bloodstream and thus potentially disseminate around the body and accumulate in specific organs.4 In addition to inhalation, NPs may be ingested through food and drinking water.5 How much plastic material we are exposed to and the potential impact of such exposures on human health is still under active investigation. In 2022, Leslie et al. reported plastics particles > 700 nm in human whole blood from healthy volunteers using pyrolysis-gas chromatography/mass spectrometry.6 A recent study showed the first evidence of plastics (diameter 5–10 μm) in the human placenta of healthy women using Raman microspectroscopy.7 These preliminary findings raise significant concerns about NPs exposure and the associated health risks, particularly during the critical periods of brain development in fetal and early neonatal life.
The ability to establish causality between environmental exposure to NPs and subsequent adverse pregnancy outcomes in humans is challenging. To overcome this challenge, the mouse is often used as a mammalian model of human pregnancy8 that in tandem has considerable cross-species alignment in specific milestones of brain development.9 Maternal exposure of mice to polystyrene microplastics have been shown to cause metabolic disorders in offspring10,11 and disturbances to the maternal–fetal immune system.12 Our group recently demonstrated that maternal exposure to environmentally-relevant concentrations of polystyrene microplastics and NPs resulted in significant fetal growth restriction in late gestation.13 Another interesting finding was that the umbilical cord length was significantly shorter in the NP-exposed group compared to controls. Short umbilical cords are associated with decreased fetal movements and potentially with abnormal fetal brain maturation.14 In the present study, behavioral tests of developmental milestones and 3D high-resolution ex vivo magnetic resonance imaging (MRI) were used to characterize the effects of maternal exposure to NPs on early postnatal brain development in mice.
2. Methods
2.1 Animals
Twenty healthy, adult female CD-1 mice (8–15 weeks of age) were purchased from Charles River Laboratories (St Constant, QC, Canada) and mated in-house. The morning that a vaginal copulation plug was detected was designated embryonic day (E) 0.5 (full term for CD-1 mice is 18.5 days). All mice were given ad libitum access to food and water in standard laboratory conditions on a 12 hour light:dark cycle (lights on at 7:00AM). All animal experiments were approved by the Institutional Care Committee at Memorial University of Newfoundland and conducted in accordance with guidelines established by the Canadian Council on Animal Care.
2.2 Experimental design
At E0.5 the dams were randomly assigned to either a NP-exposed or control group (10 dams per group). The control group received standard filtered water (filtered through a three-phase micron filtration system (5 μm, 1 μm and 0.2 μm)). The NP-exposed group received the filtered water with the addition of 50 nm plain polystyrene NPs (dispersed in deionized water, purchased from Microspheres-Nanospheres, New York, USA) at a concentration of 106 ng L−1 from E0.5 to weaning (postnatal day 23 (P23)). The size of the NPs were determined by photon correlation spectroscopy and disk centrifuge. The NP concentration was selected based on our previous work.13 The pups were not culled after birth. At P2 the pups were paw tattooed for identification. To determine if maternal NP exposure impacts postnatal growth and developmental milestones, the pups from all of the litters (123 NP-exposed pups (67 females and 56 males) from 10 litters and 120 control pups (52 females and 68 males) from 10 litters) were weighed weekly (P2, P9, P16 and P23) and neonatal neurodevelopmental outcomes were assessed (righting reflex from P2–P6 and eye opening from P9–P15). At weaning (P23) a subset of the pups (2 females and 2 males per litter, when possible) were sacrificed by a transcardiac perfusion for ex vivo high-resolution MRI. Based on our previous work, a sample size of 10–12 mice per group per sex from 5 to 8 litters is sufficient to detect local brain volume changes of 3% in cross-sectional analysis.15,16 Mice for MRI were randomly selected from each litter and care was taken to have an equal distribution of females and males in each group to reduce potential sample bias.
2.3 Behavioral testing
Behavioral tests were conducted in a dedicated behavioral testing room during the standard light phase. The dams were removed from the cages prior to running the behavioral tests on the pups. The righting reflex test was performed daily on the pups from P2 to P6. We assessed the time to right, defined as the time it took for the pup to flip over and place all four paws on the ground after being manually turned onto their backs (maximum of 30 seconds). Eye opening was observed daily from P9 to P15. Each pup was given a score depending on the number of eyes open: 0 for no eyes, 1 for either left or right eye, and 2 for both eyes open.
2.4 Brain sample preparation
At P23, a subset of mice (16 female and 15 male NP-exposed from 8 litters and 12 female and 12 male control from 6 litters) were prepared for ex vivo MRI by transcardiac perfusion.17,18 Ketamine/xylazine were used to anesthetize the mice which were then perfused through the left ventricle with 30 mL phosphate buffered saline (PBS), 1 μL mL−1 heparin and 2 mM ProHance, followed by 30 mL of 4% paraformaldehyde (PFA) and 2 mM ProHance in PBS. The brains kept within the skull were incubated in 4% PFA containing 2 mM ProHance overnight at 4 °C. The brain samples were stored in a solution containing PBS, 2 mM ProHance and sodium azide for at least 30 days before imaging.19
2.5
Ex vivo magnetic resonance imaging
Images were acquired on a 7 Tesla 306 mm horizontal bore magnet (BioSpec 70/30 USR, Bruker, Ettlingen, Germany) with a ParaVision 6.0.1 console.20,21 Using a custom-built 8-coil solenoid array, 8 brain samples were imaged in parallel using the following scan parameters: T2-weighted 3D fast spin-echo sequence using a cylindrical k-space acquisition with TR = 350 ms, TE = 12 ms, TEeff = 30 ms, echo train length = 6, four effective averages, field-of-view = 20.2 mm × 20.2 mm × 25.2 mm, matrix size = 504 × 504 × 630. Total imaging time was 13.2 hours with resulting anatomical images of 40 μm voxel isotropic resolution. Images from two control mice and from nine NP-exposed mice had to be excluded from analysis because of damage during sample preparation or because of image misregistration. Analysis was completed on 11 female and 11 male NP-exposed brain images (from 8 litters) and 11 female and 11 male control brain images (from 6 litters).
To assess anatomical differences related to maternal NP exposure, an automated image registration approach22 using the Pydpiper toolkit23 and the advanced normalization tools (ANTs) deformation algorithm24 was used. The 44 ex vivo MR images were registered using a series of linear (6 parameter, then 12 parameter) and nonlinear registration steps.22 The registration yielded deformation fields for each individual brain and the Jacobian determinants provided an estimate of the local volume changes at every voxel in each brain image.
2.6 Statistical analysis
All statistical tests were conducted using the R statistical software (http://www.r-project.org/). Data are reported as means and 95% confidence intervals. Litter size and male:female ratio were analyzed using a one-way ANOVA to evaluate the effect of group (control, NP-exposed). The body weight growth curves and righting reflex curves for each mouse were fit with a natural spline with three degrees of freedom. The eye opening score for each mouse was fit with a natural spline with six degrees of freedom. A linear mixed effects model was used to analyze the spline parameters with group (control, NP-exposed) and sex (female, male) as fixed factors and allowing for interaction between the two. Litter was not a significant factor in the behavioral outcome measures; thus, litter means were not used for statistical analysis. However, litter was treated as a random effect to account for similarity among littermates.25 Statistical significance was defined as p < 0.05. To detect neuroanatomical differences from the ex vivo MRI images, we used a linear model at every voxel with the main effects of group, sex and group-by-sex interaction. Multiple comparisons were controlled for using the false discovery rate (FDR)26 and statistical significance was defined at an FDR threshold of 10%.
3. Results and discussion
Following maternal exposure to NPs, there was no significant difference in either litter size (NP-exposed 12 fetuses/litter (CI: 10–14) vs. control 12 fetuses/litter (CI: 10–14), p = 0.8) or the male:female ratio at birth (NP-exposed 1.0 (CI: 0.5–1.5) vs. control 1.9 (CI: 0.7–3.1), p = 0.1) compared to controls. Postnatal growth curves showed the expected increased mean weight gain in males compared to females (psex<0.0001), but this difference was unaffected by NP exposure (pgroup = 0.8) (Fig. S1†). The significant growth restriction our group observed previously in late gestation fetuses exposed to NPs13 did not persist at this postnatal stage.
Maternal exposure to NPs did not impact offspring motor function with no effect of either NP exposure (pgroup = 0.2) or sex (psex = 0.7) on the time it took for pups to right themselves during postnatal days 2 to 6 (Fig. S2†). There was no significant main effect of NP exposure (pgroup = 0.3) or sex (psex = 0.07) on when the eyes opened across postnatal days 11 to 15; however, how the spline parameters changed between groups was dependent on sex (p = 0.01). Post-hoc tests revealed that the time to eye opening occurred earlier in the NP-exposed group compared to controls for the male mice (p = 0.001) but not the females (p = 0.9) (Fig. 1). Eye opening is a marker for normal postnatal development, specifically as an indicator of cortical maturation.27 This milestone is delayed in mice following exposure to environmental contaminants such as perfluorooctanoic acid.28 In contrast, earlier time to eye opening suggests accelerated brain maturation. For healthy brain development, the synchrony of development processes is critical and when there is an imbalance, the overall organization of the brain may be negatively impacted and may have long-term consequences. In humans, growth restricted neonates often demonstrate accelerated maturation of visual evoked responses29 which has been found to predict abnormal cognitive function in childhood.30 The observation that eye opening was only abnormal in male mice exposed to NPs and not females is consistent with evidence in humans that males are more vulnerable than females.31
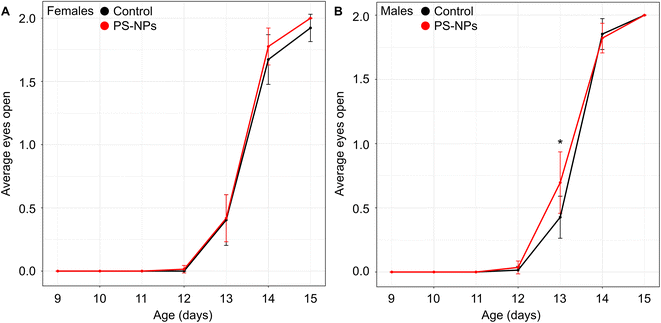 |
| Fig. 1 Developmental milestones following maternal exposure to nanoplastics. (A) There was no effect of group on when eyes opened in female mice from postnatal days 9 to 15 (p = 0.9). (B) The male mice exposed to polystyrene nanoplastics (PS-NPs, red) opened their eyes earlier than controls (black) (p = 0.001). n = 10 litters/group. Data are shown as means and 95% confidence intervals. *p < 0.05 compared to controls (as determined by a post-hoc test using a linear mixed effects model with postnatal age as the fixed effect and litter as a random effect). | |
Analysis of brain morphology using 3D high-resolution ex vivo MRI showed significant differences in specific brain regions in NP-exposed mice compared to controls (n = 11/group/sex). While there was no difference in mean whole brain volume between groups (NP-exposed 448 mm3 (CI: 440–456) vs. control 450 mm3 (CI: 440–460), pgroup = 0.7), a voxelwise comparison between NP-exposed and control mice demonstrated focal significant differences in absolute volume in grey and white matter regions of the brain that are involved in motor function, learning and memory, and physiological functions (e.g. heart rate, breathing, blood pressure, olfaction) (t-statistic = 2.97, 10% FDR, Fig. 2A). Areas of the brain that demonstrated a significant decrease in volume in the NP-exposed mice were the hypothalamus, medial septum, medulla, and olfactory bulb. Other areas of the brain were significantly larger in NP-exposed mice including the corpus callosum, areas of the cortex (primary somatosensory cortex, primary motor cortex), fornix, hippocampus, inferior colliculus, lateral ventricle, mammilothalamic tract, and striatum. Disruption in several of these brain regions have been previously associated with microplastics and NPs exposure in animal models. For example, exposure to polystyrene microplastics has been shown to cause olfactory bulb injury in goldfish.32 Adult exposure to polystyrene microplastics results in significant impairment in hippocampal-dependent learning and memory in mice.33 Jeong et al. reported that high doses of 50 nm polystyrene particles to dams (>500 μg day−1) significantly decreased the neural stem cells in the hippocampus and decreased the thickness of the corpus callosum in 1 week-old pups.34
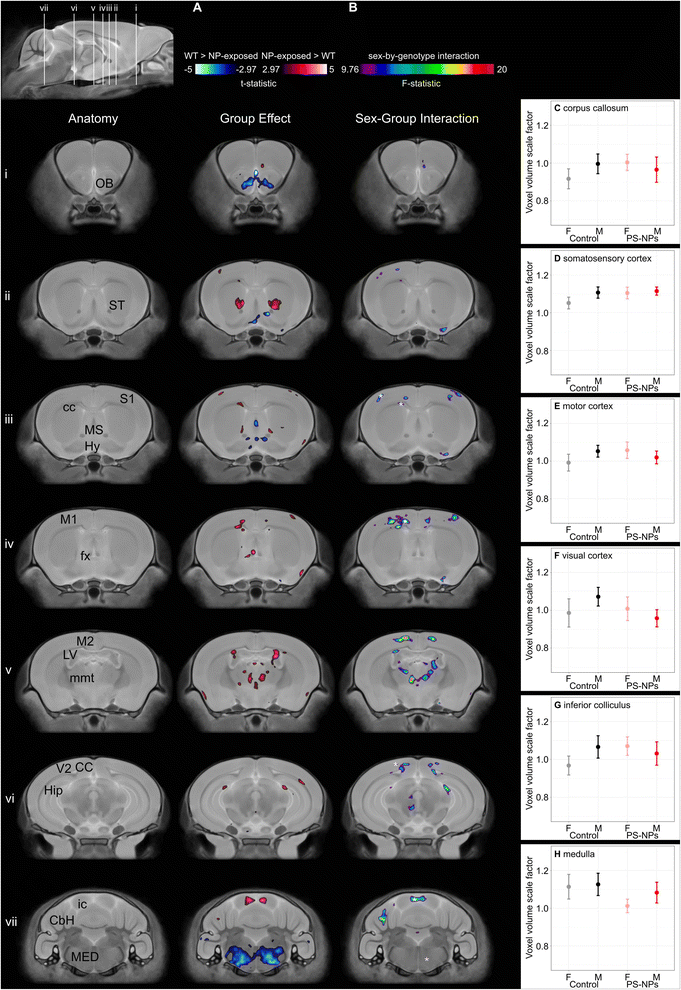 |
| Fig. 2 (A) Representative coronal slices comparing anatomical differences in the brains of control and NP-exposed mice. The images are overlaid with color maps indicating regions of absolute volume that are significantly larger (hot colors) and significantly smaller (cool colors) in NP-exposed mice compared to controls (t-statistic = 2.97, 10% FDR). (B) Representative coronal slices showing a significant sex-by-genotype interaction (F-statistic = 9.76, 10% FDR). (C–H) Volume of indicated voxels (asterisk in (A) and (B)) within several brain regions to give an indication of the scale difference illustrated in (A) and (B). The vertical axis shows the value of the Jacobian determinant, which represents a volume scale factor relative to the volume of the voxel in the average image. Data shown as means and 95% confidence intervals. n = 11 mice per sex per group. | |
A significant sex-by-group interaction demonstrated that several of the neuroanatomical differences between exposed and unexposed pups were sex-dependent (F-statistic 9.76, 10% FDR, Fig. 2B). For example, the medulla was smaller in only female NP-exposed mice (Fig. 2H). While the volume of several brain regions in the controls showed expected sexual dimorphisms, the volumes for the NP-exposed mice were either reversed or did not show sex differences. For example, the motor and visual cortex are known to be larger in males than females;17 however, in the NP-exposed mice the motor and visual cortex were larger in females than males (Fig. 2E and F). For the corpus callosum, primary somatosensory cortex and inferior colliculus, these male brain structures were larger than in females within the controls mice while no significant sex differences were found in NP-exposed mice (Fig. 2C,D and G). The reduction in the volume of the visual cortex observed in males exposed to NPs, but not in females, is consistent with the earlier time to eye opening observed only in males. In humans, visual responses are related to voluntary factors as well as maturation of behavioral state transitions. Since many of the MRI-detected brain areas impacted by NP exposure are involved in behavioral state control, the abnormal time to eye opening may reflect neurologic reprogramming and may be linked to abnormal behavioral state transitions.35 The NP exposure in this study occurred during a critical period of sexual differentiation during which the structure of the brain is being organized. While it is not clear what is causing the sex differences observed in this study, the differential impact of toxicants on brain development can be influenced by a variety of factors including hormones and sex chromosomes.36 Anatomical MRI is a highly sensitive tool for detecting morphological differences but cannot on its own establish the cause of these changes. In the context of environmental exposures during pregnancy the potential mechanisms are numerous, including an effect of the NP exposure on the supply of metabolic substrates from the mother to the fetus, effects on signaling molecules controlling fetal growth, and direct effects on the cells in the cerebral cortex, hippocampus, striatum, and medulla where the volumetric differences are seen on the MRI. That such effects would be modified by fetal sex is plausible given other examples, such as maternal exposure of mice to fine particulate matter (<2.5 μm) which has sex dependent effects on lipid and cytokine profiles in the liver37 and on glial cell activation in the brain.38 Identifying these causal pathways will require a multi-facet approach that examines changes at a molecular and cellular level as well as the kinetics of the NPs themselves.
The present study has several limitations. One is that the litters were not culled to help ensure equal food distribution amongst the pups.39 While the litters sizes were not significantly different between groups, it is possible that some of the variability in our measurements could have been the result of differences in availability of food resources. Another limitation is that while structural MRI provides information about changes in brain volume on the macroscopic and mesoscopic scale, it does not reveal cellular and molecular level changes. As a result, we were unable to link the MRI outcomes to the cellular and molecular mechanisms. Immunohistochemistry and a histological morphometric analysis would help explain the sex-dependent brain volume changes following maternal exposure to NPs. Future studies will focus on understanding the physiological responses following NP exposure, the cellular mechanisms by which the NPs affect the brain, and determining how this differs between females and males.
4. Conclusions
In summary, behavioral assays and high-resolution ex vivo MRI revealed sex-specific differences in the timing of developmental milestones and in neuroanatomy following maternal exposure to polystyrene NPs. The use of unbiased 3D whole brain imaging allowed us to identify specific brain regions that are impacted by maternal exposure to NPs, involving structures within the limbic system, the midbrain and specific areas of the cerebral cortex. This work adds to the growing body of literature demonstrating significant impacts of exposure to microplastics and NPs in the perinatal period of mammalian brain development. Further studies are needed to determine any threshold effect of NP concentrations in the environment, in order to guide the development of regulations to minimize potentially harmful human exposure and thus protect the health of future generations of children.
Abbreviations
E | Embryonic |
FDR | False discovery rate |
MRI | Magnetic resonance imaging |
NPs | Nanoplastics |
P | Postnatal |
PBS | Phosphate buffered saline |
PFA | Paraformaldehyde |
PS | Polystyrene |
CbH | Cerebellar hemisphere |
cc | Corpus callosum |
CC | Cingulate cortex |
fx | Fornix |
Hip | Hippocampus |
Hy | Hypothalamus |
ic | Inferior colliculus |
LV | Lateral ventricle |
M1 | Primary motor cortex |
M2 | Secondary motor cortex |
MED | Medulla |
mmt | Mammilothalamic tract |
MS | Medial septum |
OB | Olfactory bulbs |
S1 | Primary somatosensory cortex |
ST | Striatum |
V2 | Secondary visual cortex |
Conflicts of interest
There are no conflicts to declare.
Acknowledgements
This work was supported by the Government of Canada's New Frontiers in Research fund (NFRF).
References
- R. Geyer, J. R. Jambeck and K. L. Law, Production, use, and fate of all plastics ever made, Sci. Adv., 2017, 3, e1700782 CrossRef PubMed.
- R. M. Maertens, J. Bailey and P. A. White, The mutagenic hazards of settled house dust: a review, Mutat. Res., 2004, 567, 401 CAS.
- Y. Huang, X. Qing, W. Wang, G. Han and J. Wang, Mini-review on current studies of airborne microplastics: Analytical methods, occurrence, sources, fate and potential risk to human beings, TrAC, Trends Anal. Chem., 2020, 125, 1 CrossRef.
- J. Gasperi, S. L. Wright, R. Dris, F. Collard, C. Mandin, M. Guerrouache, V. Langlois, F. H. Kelly and B. Tassin, Microplastics in air: are we breathing it in?, Curr. Opin. Environ. Sci., 2018, 1, 1 Search PubMed.
- Q. Zhang, E. G. Xu, J. Li, Q. Chen, L. Ma, E. Y. Zeng and H. Shi, A review of microplastics in table salt, drinking water, and air: direct human exposure, Environ. Sci. Technol., 2020, 54, 3740 CrossRef CAS PubMed.
- H. A. Leslie, M. J. M. van Velzen, S. H. Brandsma, A. D. Vethaak, J. J. Garcia-Vallejo and M. H. Lamoree, Discovery and quantification of plastic particle pollution in human blood, Environ. Int., 2022, 163, 107199 CrossRef CAS PubMed.
- A. Ragusa, A. Svelato, C. Santacroce, P. Catalano, V. Notarstefano, O. Carnevali, F. Papa, M. C. A. Rongioletti, F. Baiocco, S. Draghi, E. D'Amore, D. Rinaldo, M. Matta and E. Giorgini, Plasticenta: First evidence of microplastics in human placenta, Environ. Int., 2021, 146, 106274 CrossRef CAS PubMed.
- P. Georgiades, A. C. Ferguson-Smith and G. J. Burton, Comparative developmental anatomy of the murine and human definitive placentae, Placenta, 2002, 23, 3 CrossRef CAS PubMed.
- B. Clancy, B. L. Finlay, R. B. Darlington and K. J. S. Anand, Extrapolating brain development from experimental species to humans, Neurotoxicology, 2007, 28, 931 CrossRef PubMed.
- T. Luo, Y. Zhang, C. Wang, X. Wang, J. Zhou, M. Shen, Y. Zhao, Z. Fu and Y. Jin, Maternal exposure to different sizes of polystyrene microplastics during gestation causes metabolic disorders in their offspring, Environ. Pollut., 2019, 255, 113122 CrossRef CAS PubMed.
- T. Luo, C. Wang, Z. Pan, C. Jin, Z. Fu and Y. Jin, Maternal polystyrene microplastic exposure during gestation and lactation altered metabolic homeostasis in the dams and their F1 and F2 offspring, Environ. Sci. Technol., 2019, 53, 10978 CrossRef CAS PubMed.
- J. Hu, X. Qin, J. Zhang, Y. Zhu, W. Zeng, Y. Lin and X. Liu, Polystyrene microplastics disturb maternal-fetal immune balance and cause reproductive toxicity in pregnant mice, Reprod. Toxicol., 2021, 106, 42 CrossRef CAS PubMed.
- Z. Aghaei, J. G. Sled, J. C. Kingdom, A. A. Baschat, P. A. Helm, K. J. Jobst and L. S. Cahill, Maternal exposure to polystyrene micro- and nanoplastics causes fetal growth restriction in mice, Environ. Sci. Technol. Lett., 2022, 9, 426 CrossRef CAS.
- A. C. Moessinger, W. A. Blanc, P. A. Marone and D. C. Polsen, Umbilical cord length as an index of fetal activity: experimental study and clinical implications, Pediatr. Res., 1982, 16, 109 CrossRef CAS PubMed.
- J. P. Lerch, A. P. Yiu, A. Martinez-Canabal, T. Pekar, V. D. Bohbot, P. W. Frankland, R. M. Henkelman, S. A. Josselyn and J. G. Sled, Maze training in mice induces MRI-detectable brain shape changes specific to the type of learning, NeuroImage, 2011, 54, 2086 CrossRef PubMed.
- L. S. Cahill, J. Hoggarth, J. P. Lerch, M. Seed, C. K. Macgowan and J. G. Sled, Fetal brain sparing in a mouse model of chronic maternal hypoxia, J. Cereb. Blood Flow Metab., 2019, 39, 1172 CrossRef PubMed.
- S. Spring, J. P. Lerch and R. M. Henkelman, Sexual dimorphism revealed in the structure of the mouse brain using three-dimensional magnetic resonance imaging, NeuroImage, 2007, 35, 1424 CrossRef PubMed.
- L. S. Cahill, C. L. Laliberté, J. Ellegood, S. Spring, J. A. Gleave, M. C. van Eede, J. P. Lerch and R. M. Henkelman, Preparation of fixed mouse brains for MRI, NeuroImage, 2012, 60, 933 CrossRef PubMed.
- A. E. de Guzman, M. D. Wong, J. A. Gleave and B. J. Nieman, Variations in post-perfusion immersion fixation and storage alter MRI measurements of mouse brain morphometry, NeuroImage, 2016, 142, 687 CrossRef PubMed.
- M. Zuo, N. M. Fettig, L.-P. Bernier, E. Pössnecker, S. Spring, A. Pu, X. I. Ma, D. S. W. Lee, L. A. Ward, A. Sharma, J. Kuhle, J. G. Sled, A.-K. Pröbstel, B. A. MacVicar, L. C. Osborne, J. L. Gommerman and V. Ramaglia, Age-dependent gray matter demyelination is associated with leptomeningeal neutrophil accumulation, JCI Insight, 2022, 7, e158144 CrossRef PubMed.
- A. Arbabi, L. Spencer Noakes, D. Vousden, J. Dazai, S. Spring, O. Botelho, T. Keshavarzian, M. Mattingly, J. E. Ellegood, L. M. J. Nutter, R. Wissmann, J. G. Sled, J. P. Lerch, R. M. Henkelman and B. J. Nieman, Multiple-mouse magnetic resonance imaging with cryogenic radiofrequency probes for evaluation of brain development, NeuroImage, 2022, 252, 119008 CrossRef CAS PubMed.
- B. J. Nieman, M. C. van Eede, S. Spring, J. Dazai, R. M. Henkelman and J. P. Lerch, MRI to assess neurological function, Curr. Protoc. Mouse Biol., 2018, 8, e44 CrossRef PubMed.
- M. Friedel, M. C. van Eede, J. Pipitone, M. M. Chakravarty and J. P. Lerch, Pydpiper: a flexible toolkit for constructing novel registration pipelines, Front. Neuroinform., 2014, 8, 67 Search PubMed.
- B. B. Avants, N. Tustison and G. Song, Advanced normalization tools (ANTS), Insight J, 2009, 2, 1 Search PubMed.
- M. S. Golub and C. A. Sobin, Statistical modeling with litter as a random effect in mixed models to manage “interlitter likeness”, Neurotoxicol. Teratol., 2020, 77, 106841 CrossRef CAS PubMed.
- C. R. Genovese, N. A. Lazar and T. Nichols, Thresholding of statistical maps in functional neuroimaging using the false discovery rate, NeuroImage, 2002, 15, 870 CrossRef PubMed.
- S. P. Gandhi, J. Cang and M. P. Stryker, An eye-opening experience, Nat. Neurosci., 2005, 8, 9 CrossRef CAS PubMed.
- C. Lau, J. R. Thibodeaux, R. G. Hanson, M. G. Narotsky, J. M. Rogers, A. B. Lindstrom and M. J. Strynar, Effects of perfluorooctanoic acid exposure during pregnancy in the mouse, Toxicol. Sci., 2006, 90, 510 CrossRef CAS PubMed.
- S. A. Scherjon, H. Oosting, B. W. de Visser, T. de Wilde, H. A. Zondervan and J. H. Kok, Fetal brain sparing is associated with accelerated shortening of visual evoked potential latencies during early infancy, Am. J. Obstet. Gynecol., 1996, 175, 1569 CrossRef CAS PubMed.
- S. Scherjon, J. Briët, H. Oosting and J. Kok, The discrepancy between maturation of visual-evoked potentials and cognitive outcome at five years in very preterm infants with and without hemodynamic signs of fetal brain-sparing, Pediatrics, 2000, 105, 385 CrossRef CAS PubMed.
- S. Kraemer, The fragile male, BMJ, 2000, 321, 1609 CrossRef CAS PubMed.
- W. Shi, S. Sun, Y. Han, Y. Tang, W. Zhou, X. Du and G. Liu, Microplastics impair olfactory-mediated behaviors of goldfish Carassius auratus, J. Hazard. Mater., 2021, 409, 125016 CrossRef CAS PubMed.
- C.-W. Lee, L.-F. Hsu, I.-L. Wu, Y.-L. Wang, W.-C. Chen, Y.-J. Liu, L.-T. Yang, C.-L. Tan, Y.-H. Luo, C. C. Wang, H.-W. Chiu, T. C.-K. Yang, Y.-Y. Lin, H.-A. Chang, Y.-C. Chiang, C.-H. Chen, M.-H. Lee, K.-T. Peng and C. C.-Y. Huang, Exposure to polystyrene microplastics impairs hippocampus-dependent learning and memory in mice, J. Hazard. Mater., 2022, 430, 128431 CrossRef CAS PubMed.
- B. Jeong, J. Y. Baek, J. Koo, S. Park, Y.-K. Ryu, K.-S. Kim, S. Zhang, C. Chung, R. Dogan, H.-S. Choi, D. Um, T.-K. Kim, W. S. Lee, J. Jeong, W.-H. Shin, L.-R. Lee, N.-S. Kim and D. Y. Lee, Maternal exposure to polystyrene nanoplastics causes brain abnormalities in progeny, J. Hazard. Mater., 2022, 426, 127815 CrossRef CAS PubMed.
- A. A. Baschat, Neurodevelopment following fetal growth restriction and its relationship with antepartum parameters of placental dysfunction, Ultrasound Obstet. Gynecol., 2011, 37, 501 CrossRef CAS PubMed.
- C. Torres-Rojas and B. C. Jones, Sex differences in neurotoxicogenetics, Front. Genet., 2018, 9, 196 CrossRef PubMed.
- N. D. Agnish and K. A. Keller, The rationale for culling of rodent litters, Fundam. Appl. Toxicol., 1997, 38, 2 CrossRef CAS PubMed.
- H. Chen, D. Van Reyk, A. Oliveira, Y. L. Chan, S. E. Town, B. Rayner, C. A. Pollock, S. Saad, J. George, M. P. Padula and B. G. Oliver, Sex-dependent responses to maternal exposure to PM2.5 in the offspring, Antioxidants, 2022, 11, 2255 CrossRef CAS PubMed.
- J. L. Allen, X. Liu, D. Weston, L. Prince, G. Oberdörster, J. N. Finkelstein, C. J. Johnston and D. A. Cory-Slechta, Developmental exposure to concentration ambient ultrasound particulate matter air pollution in mice results in persistent and sex-dependent behavioral neurotoxicity and glial activation, Toxicol. Sci., 2014, 140, 160 CrossRef CAS PubMed.
|
This journal is © The Royal Society of Chemistry 2023 |