DOI:
10.1039/C4RA12548G
(Paper)
RSC Adv., 2015,
5, 440-447
Magnesium complexes bearing N,N-bidentate phenanthrene derivatives for the stereoselective ring-opening polymerization of rac-lactides†
Received
16th October 2014
, Accepted 20th November 2014
First published on 20th November 2014
Abstract
Three unreported magnesium complexes (1: R = C6H5, 2: R = 2,6-Et2C6H3, 3: R = 2-tBuC6H4) bearing N,N-bidentate phenanthrene derivatives derived from Schiff bases were synthesized. These complexes were characterized using 1H and 13C NMR spectroscopy and elemental analyses and employed for rac-lactide and L-lactide polymerization. Complex 1 showed the highest activity among these Mg complexes for the ring-opening polymerization (ROP) of L-lactide, and complex 3 showed the highest stereoselectivity for the ROP of rac-lactide, achieving partially heterotactic polylactide (PLA) with a Pr of 0.76. The polymerization kinetics utilizing 2 as a catalyst were researched in detail. The kinetics of the polymerization data revealed that the rate of polymerization was first-order with respect to monomer, and had an order of 0.97 with respect to catalyst. There was a linear relationship between the L-lactide conversion and the number-average molecular weight of PLA.
Introduction
Biodegradable and biocompatible polymers have been exploited for a wide range of applications such as sutures, disposal containers, bone fracture fixation devices, controlled release drug carriers, scaffolds, textiles and tissue engineering.1,2 In particular, polylactide (PLA) derived from lactic acid, which is a renewable resource, has proved to be one of the most promising polyesters exhibiting biological degradation.2 The presence of two chiral centers in the lactide monomer results in different lactide stereoisomers, namely L-lactide (L-LA), D-lactide (D-LA) and meso-lactide (Fig. 1). The stereochemistry of the polymer chains influences PLA’s physical and chemical properties.1b Commonly, PLA is synthesized by the ring-opening polymerization (ROP) of lactide initiated by metal complexes, such as some alkoxides of Sn,3 Al,4,13,14 Zn,5 Mg,6 Fe,7 Ti,8 In,9 rare-earth metals,10 organocatalysts,11 and enzymes.12 Among them, biocompatible zinc and magnesium provoke researchers’ attention because they are essential nutrients and minerals for plants and humans.1h Moreover, zinc and magnesium catalysts are highly effective initiators employed for the ROP of PLA.5 In this context, our group has reported some zinc complexes derived from β-diketone ligands.5i These complexes proved to be highly effective single-site living initiators for the well-controlled ROP of lactides. Furthermore, the more sterically hindered zinc catalysts offered effective control of the polymer microstructures in the ROP of rac-lactide. In the past twenty years, much effort has been expended in the selection of proper ancillary ligands to enhance the performance of initiators in polymerizations. A number of research workers have tried to expound the relation among rac-lactide (rac-LA), metal complexes and the tacticity of polymers (see Fig. 2).13,14
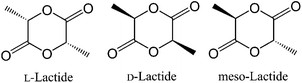 |
| Fig. 1 Stereoisomers of lactide. | |
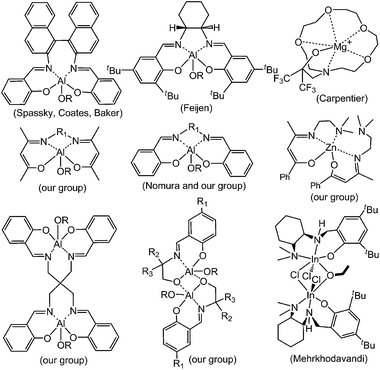 |
| Fig. 2 Initiators for the stereoselective ROP of lactide. | |
However, to our knowledge, little research on magnesium complexes bearing diamido derivatives derived from phenanthrene Schiff base derivatives (see Scheme 1) has been carried out for the stereoselective ROP of rac-LA. Based on the efficient uses of magnesium complexes,1h,6b,d,e we believed that magnesium complexes bearing N,N-bidentate diamido ligands might be potential catalysts for the ROP of rac-LA. In this work, the preliminary results of the preparation of magnesium complexes bearing phenanthrene derivatives and their use as initiators to polymerize rac-LA in a controlled manner are reported.
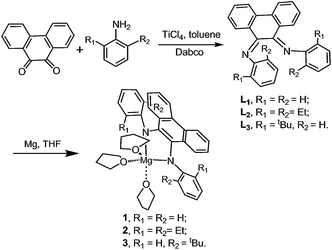 |
| Scheme 1 Synthesis of pro-ligands and Mg complexes. | |
Results and discussion
Synthesis of pro-ligands
As shown in Scheme 1, pro-ligands L1, L2 and L3 were prepared in middling yields (35.2–64.7%) by the condensation reaction between phenanthraquinone and modified aniline via catalysis with titanic chloride and dabco in toluene according to the literature.15 The 1H NMR spectra of the pro-ligands displayed two sets of signals that could be assigned to two different ortho-substituents at the phenyl rings. For example, the resonances δ 1.07 and 0.74 ppm in the 1H NMR spectrum of pro-ligand L2 were assigned to the two sets of primary protons from the ethyls (see Fig. 3) This revealed that these ligands possess Z/E configuration.16 A similar situation was also reported in Ar′,Ar–BIAN ligands.17
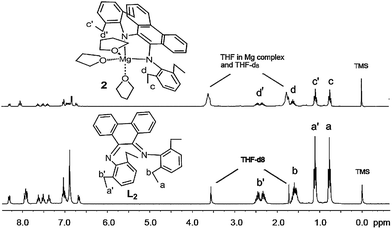 |
| Fig. 3 Stacked 400 MHz 1H NMR spectra of pro-ligand L2 and complex 2, in THF-d8. | |
Synthesis of magnesium complexes
As shown in Scheme 1, Mg complexes 1–3 were prepared easily in high yields (84.7–95.6%) by combining magnesium with corresponding pro-ligands in a glovebox and were isolated as red solids from THF. The magnesium was activated by iodine and rinsed with THF three times. All three complexes were sensitive to air and moisture. Complexes 1–3 were characterized using 1H and 13C NMR spectroscopy in THF-d8 at room temperature and elemental analysis. The 1H and 13C NMR spectra of complexes 1–3 showed one ligand and three THF molecules were coordinated to magnesium in these complexes. For instance, the resonances (δ 3.58 and 1.73 ppm) in the 1H NMR spectrum of complex 2 were assigned to the secondary protons in three THF molecules coordinating to complex 2 (see Fig. 3). Interestingly, the 1H NMR spectra of 1–3 revealed that the phenyl groups of the ligands in these complexes were inequivalent. For example, two groups of resonances from ethyls (δ 2.52–2.18, 1.58, 1.05, 0.71 ppm) are observed in the 1H NMR spectrum (see Fig. 3). It appeared the geometry of 2 in solution was “b” rather than “a” (Fig. S1†), i.e. the Mg atom in 2 exhibits a trigonal bipyramidal geometry and the two N donors occupied axial and equatorial positions. This was consistent with the single crystal structure of 2 in the solid state. Similar configurations have also been seen in salan aluminium complexes.13e The geometry of complex 2 in the solid state was confirmed via X-ray diffraction analysis. The molecular structure is depicted in Fig. 4. Selected bond distances and angles and other crystallographic data are listed in Table 1 and Table S1,† respectively. X-Ray structural analysis revealed that complex 2 is mononuclear and the central magnesium atom is coordinated by two N atoms and three O atoms. In complex 2 (see Table 1), the Mg1–O1 bond length, 2.161(2) (Å), was the longest among these bond lengths; N1–Mg1–O2, N1–Mg1–N2, N2–Mg1–O3 and O2–Mg1–O3 angles were 116.10(10), 80.60(10), 96.62(10) and 110.67(9)°, respectively. The quantity of the distortion can be calculated by structural index parameter τ.18 The τ values range from 0 (perfectly square pyramidal) to 1 (perfectly trigonal bipyramidal). For complex 2, the τ value was 0.59, which suggests the magnesium is in a distorted trigonal bipyramidal environment. The C–N bond average distance of 1.397 Å for 2 (see Table 1) approximated that of 1.390 Å in BIAN magnesium complex 1 (ref. 19) reported previously. It showed that the ligand bonded in a dianionic diamido manner. In other words, the pro-ligand was a Schiff base, but upon reaction with Mg, oxidative addition occurred in which Mg(0) was oxidized to Mg(2+) and the Schiff base was reduced to a diamido ligand. Hence, the Mg complex should be referred to as a diamido magnesium complex.
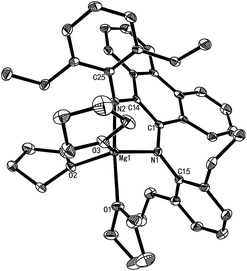 |
| Fig. 4 Perspective view of 2 with thermal ellipsoids drawn at the 30% probability level. Hydrogens are omitted for clarity. | |
Table 1 Selected bond lengths (Å) and angles (°) for complex 2
Mg1–N1 |
2.051(3) |
N1–Mg1–O2 |
116.10(10) |
Mg1–N2 |
2.071(3) |
N1–Mg1–N2 |
80.60(10) |
Mg1–O1 |
2.161(2) |
N2–Mg1–O3 |
96.62(10) |
Mg1–O2 |
2.078(2) |
O2–Mg1–O3 |
110.67(9) |
Mg1–O3 |
2.085(2) |
|
|
C1–N1 |
1.384(4) |
|
|
C14–N2 |
1.409(4) |
|
|
Lactide polymerization
All magnesium complexes were studied as catalysts for the ROP of L-LA or rac-LA. The polymerization reactions were performed in tetrahydrofuran or toluene, and representational polymerization data are recorded in Table 2. These magnesium complexes showed moderate to high activities (87.8–97.7% monomer conversion) with the co-catalysis of isopropanol at 70 °C. 1H NMR and GPC were used to confirm the Mn values of the PLA. The number-averaged molecular weights (measured via 1H NMR spectroscopy20) were close to theoretical values (calculated from the monomer/magnesium complexes molar ratio). The activities of these complexes declined with the increase of substituent bulk on the phenyl rings. Among the three complexes, the monomer conversion for complex 1 was the highest under the same conditions (see Fig. 5, Table 2, Entries 1–6).
Table 2 Polymerization data of LA with complexes 1–3a
Entry |
Catalyst |
Monomer |
Temp. (°C) |
t (h) |
[LA]/[cat.] |
Conv.b (%) |
Mn(calcd)c × 10−4 |
MnGPCd × 10−4 |
PDId |
kapp (h−1) × 10−3 |
Pr |
The polymerization reactions were carried out in toluene solution except that several actions processed in THF at 25 and 40 °C, [LA]0 = 0.5 mol L−1, [isopropanol]/[cat.] = 1.0. Measured using 1H NMR spectroscopy. Computed from the 144 × [LA]0/[cat.]0 × conversion + Mwisopropanol. From GPC analysis and calibration against a polystyrene standard. The veritable value of Mn(calcd) could be computed according to the formula Mn = 0.58MnGPC.20 |
1 |
1 |
L-LA |
70 |
6 |
100 |
97.7 |
1.41 |
2.52 |
1.30 |
n.a. |
0 |
2 |
2 |
L-LA |
70 |
6 |
100 |
93.6 |
1.35 |
2.40 |
1.17 |
n.a. |
0 |
3 |
3 |
L-LA |
70 |
6 |
100 |
89.4 |
1.29 |
2.26 |
1.21 |
n.a. |
0 |
4 |
1 |
L-LA |
40 |
12 |
100 |
71.3 |
1.03 |
1.72 |
1.15 |
103.2 |
0 |
5 |
2 |
L-LA |
40 |
12 |
100 |
64.0 |
0.92 |
1.54 |
1.10 |
82.5 |
0 |
6 |
3 |
L-LA |
40 |
12 |
100 |
58.3 |
0.84 |
1.45 |
1.13 |
72.4 |
0 |
7 |
2 |
L-LA |
40 |
12 |
75 |
70.5 |
1.05 |
1.71 |
1.11 |
107.6 |
0 |
8 |
2 |
L-LA |
40 |
12 |
125 |
55.9 |
1.01 |
1.74 |
1.12 |
65.8 |
0 |
9 |
2 |
L-LA |
40 |
12 |
150 |
51.7 |
1.12 |
1.94 |
1.10 |
53.7 |
0 |
10 |
1 |
rac-LA |
70 |
6 |
100 |
95.6 |
1.38 |
2.31 |
1.35 |
n.a. |
0.51 |
11 |
2 |
rac-LA |
70 |
6 |
100 |
92.2 |
1.33 |
2.17 |
1.22 |
n.a. |
0.55 |
12 |
3 |
rac-LA |
70 |
6 |
100 |
87.8 |
1.26 |
2.21 |
1.28 |
n.a. |
0.58 |
13 |
1 |
rac-LA |
40 |
12 |
100 |
69.2 |
1.00 |
1.53 |
1.21 |
n.a. |
0.56 |
14 |
2 |
rac-LA |
40 |
12 |
100 |
61.9 |
0.89 |
1.57 |
1.13 |
n.a. |
0.65 |
15 |
3 |
rac-LA |
40 |
12 |
100 |
56.9 |
0.82 |
1.44 |
1.14 |
n.a. |
0.71 |
16 |
3 |
rac-LA |
25 |
20 |
100 |
52.4 |
0.75 |
1.29 |
1.13 |
n.a. |
0.76 |
17 |
1 |
L-LA |
90 |
16 |
400 |
98.0 |
5.64 |
9.85 |
1.40 |
n.a. |
0 |
18 |
2 |
L-LA |
90 |
18 |
400 |
92.3 |
5.32 |
9.77 |
1.33 |
n.a. |
0 |
19 |
3 |
L-LA |
90 |
18 |
400 |
87.5 |
5.04 |
8.82 |
1.38 |
n.a. |
0 |
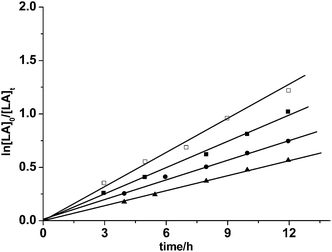 |
| Fig. 5 Kinetics of the ROP of L-LA by 2 with isopropanol at 40 °C in THF with ([LA]0 = 0.5 mol L−1; kp = kapp/[cat.]0). ■: L-LA, [cat.]0 = 0.005 mol L−1, kapp = 82.5 × 10−3 h−1; □: L-LA, [cat.]0 = 0.0067 mol L−1, kapp = 107.6 × 10−3 h−1; ●: L-LA, [cat.]0 = 0.004 mol L−1, kapp = 65.8 × 10−3 h−1; ▲: L-LA, [cat.]0 = 0.0033mol L−1, kapp = 53.7 × 10−3 h−1. | |
Stereoselective polymerization
We have also studied poly(rac-LA) (Table 2, Entry 16) from the homonuclear decoupled 1H NMR spectrum of the methane fragment21 (see Fig. 6). The Pr (ref. 22) value, 0.76, certified that these polymer chains were heterotactic enriched. The results indicated that the Pr selectivities increased from 0.51 to 0.58 with the increase of the bulk of the substituents on the pro-ligands (from hydrogen atoms to tert-butyls on the phenyl rings) at 70 °C (see Table 2, Entries 10 and 12). The stereoregularity of the poly(rac-LA) was also affected by the reaction temperature. For complex 3, on reducing the temperature from 70 to 25 °C, the Pr value increased from 0.58 to 0.76 (Table 2, Entries 12 and 16).
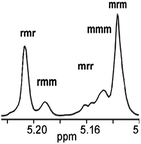 |
| Fig. 6 Homonuclear decoupled 1H NMR spectrum of the methine part of poly(rac-LA) prepared using complex 3 at 25 °C, Pr = 0.76, (Table 2, Entry 16, 400 MHz, CDCl3). | |
Kinetics of lactide polymerization
The variation of the kinetics of the ROP of L-LA with some conditions, such as monomer/initiator ratio, were researched in THF employing 2. The molecular weight (Mn) of the polymers increased linearly with increasing monomer conversion. The PDI values of these polymers remained low (1.05–1.10). This illustrated the living characteristic of the catalytic systems (Fig. 7). In order to calculate the order in initiator, the kapp value was plotted versus the concentration of complex 2 (see Fig. 8). For each condition, first-order kinetics in monomer was seen (see Fig. 8). So the ROP of LA by 2 speculatively agreed with the formula:where kapp = kp[cat.]x, and kp is the propagation speed constant. In order to calculate the order in catalyst, ln
kapp was plotted versus ln[cat.]0 (Fig. 9). The order in catalyst was calculated as 0.97–1. So the ROP of LA by 2 followed the entire kinetic equation: |
−d[LA]/dt = kp[cat.]0.97[LA]
| (2) |
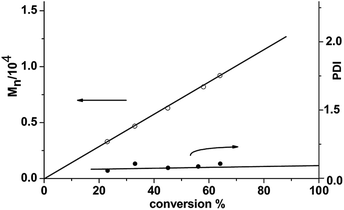 |
| Fig. 7 Plots of PLA Mn and PDI in the light of L-LA conversion employing complex 2/isopropanol, [LA]0/[cat.]0 = 100, at 40 °C in THF. | |
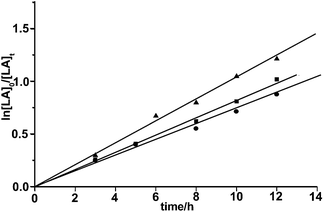 |
| Fig. 8 Kinetics of the ROP of L-LA by 1–3 with isopropanol at 40 °C in toluene with [LA]0 = 0.5 mol L−1, [cat.]0 = 5 × 10−3 mol L−1, [LA]0/[cat.]0 = 100, ▲: 1, kapp = 103.2 × 10−3 h−1; ■: 2, kapp = 82.5 × 10−3 h−1; ●: 3, kapp = 72.4 h−1 × 10−3 h−1. | |
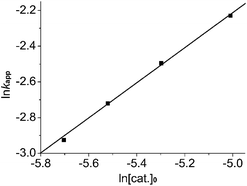 |
| Fig. 9 ln kapp versus the ln[cat.]0 of 2/isopropanol initiator for the L-LA polymerization at 40 °C in THF. | |
In addition, complexes 1–3 were also examined as catalysts in high [LA]0/[cat.]0 molar ratio ([LA]0/[cat.]0 = 400) for the ROP of L-LA in toluene solution at 90 °C, where high degrees of monomer conversion (87.5–98.0%) were achieved (Table 2, Entries 17–19).
Mechanism of lactide polymerization
Chain end analysis of the oligomer of L-LA, which was prepared by the polymerization of L-LA at low monomer/initiator ratio ([LA]t
:
[2]t = 41
:
1) (Fig. 10) was measured using 1H NMR spectroscopy. The integral ratio of the two peaks at δ 1.24 ppm (assigned to the methyl protons from the isopropoxycarbonyl end-group, “a” in Fig. 10) and δ 4.34 ppm (assigned to the methine proton neighboring the hydroxyl end-group, “d” in Fig. 10) approximated 6
:
1. This meant the aggregating chain was end-capped by an isopropyl ester with a hydroxyl.14a,d In other words, the magnesium complex had transformed isopropoxy magnesium reactive species at the initiation of the aggregation, so the genuine initiator might be the isopropoxy magnesium species. This ROP of lactide using magnesium complexes adopted a coordination insertion mechanism.6a
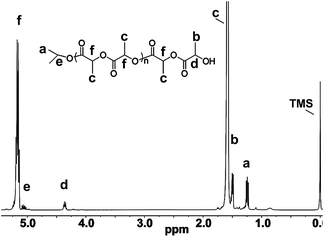 |
| Fig. 10 1H NMR spectrum of oligomer of L-LA at low monomer/initiator ratio ([LA]t : [2]t = 41 : 1) in CDCl3. | |
Experimental section
General
All experimental operations were performed using Schlenk techniques.23,24 Elemental analysis was accomplished using a Varian EL microanalyzer; 1H NMR and 13C NMR spectra were measured on Bruker AV 400M or 300M instruments at RT in THF-d8 or CDCl3 with TMS for compounds and polymers. Gel permeation chromatography (GPC) measurements were conducted with a Waters 515 GPC with CHCl3 as the eluant (flow rate: 1 mL min−1, at 35 °C). The molecular weight was adjusted through a PS standard. Crystallographic data were gathered and analyzed according to the experimental sections of ref. 14b–d, 25. Magnesium, titanic chloride, isopropanol, 9,10-phenanthrenequinone, 1,4-diazabicyclo[2.2.2]octane (dabco), aniline, 2,6-diethylaniline and 2-tert-butylaniline were purchased from J&K Scientific Ltd.
Synthesis of pro-ligands (general procedure)
To a stirred solution of aniline (50 mmol) and dabco (150 mmol) in toluene (130 mL), was added TiCl4 (50 mmol) in toluene (25 mL) dropwise at 90 °C, then 9,10-phenanthrenequinone (25 mmol) was added. The reaction was maintained at 140–145 °C for 20–24 h and was then cooled to ca. 80 °C. Insoluble substance was removed by hot filtration and then the solvent was removed under vacuum. The product was isolated as a deep red solid by silica-gel column chromatography (Vpetroleum
:
Vethyl
acetate = 6
:
1).
L1. Yield 64.7%. 1H NMR (400 MHz, THF-d8, δ, ppm): 8.42 (d, J = 7.2 Hz, 1H, ArH), 8.28 (d, J = 7.9 Hz, 2H, ArH), 8.20 (t, J = 7.6 Hz, 1H, ArH), 7.56 (t, J = 7.4 Hz, 1H, ArH), 7.46 (t, J = 7.5 Hz, 1H, ArH), 7.21–6.84 (m, 11H, ArH), 6.69 (d, J = 7.8 Hz, 1H, ArH). 13C NMR (100 MHz, THF-d8, δ, ppm): 162.2, 158.5, 149.1, 147.8, 136.0, 132.4, 133.6, 131.9, 131.8, 129.4, 128.6, 128.0, 127.4, 127.0, 126.8, 125.5, 124.6, 124.3, 123.6, 123.3, 122.7. Anal. calcd for C26H18N2 (%): C, 87.12; H, 5.06; N, 7.82. Found: C, 87.15; H, 5.10; N, 7.86. HRMS (m/z): calcd for C26H18N2: 358.15; found: 358.20 [M + H]+.
L2. Yield 45.3%. 1H NMR (400 MHz, THF-d8, δ, ppm): 8.32 (d, J = 7.4 Hz, 1H, ArH), 7.93 (m, 2H, ArH), 7.49 (t, J = 7.4 Hz, 1H, ArH), 7.36 (t, J = 7.6 Hz, 1H, ArH), 7.09–6.94 (m, 3H, ArH), 6.92–6.84 (m, 5H, ArH), 6.68 (d, J = 7.9 Hz, 1H, ArH), 2.36 (ddt, J = 36.5, 15.0, 7.6 Hz, 4H, CH2CH3), 1.72–1.50 (m, 4H, CH2CH3), 1.07 (t, J = 6.0 Hz, 6H, CH2CH3), 0.74 (t, J = 6.0 Hz, 6H, CH2CH3). 13C NMR (100 MHz, THF-d8, δ, ppm): 159.04, 134.86, 134.15, 133.62, 131.91, 131.40, 130.77, 130.43, 129.08, 128.78, 128.12, 127.41, 127.27, 125.42, 124.66, 123.61, 123.55, 122.80, 24.82, 23.39, 14.89, 13.36. Anal. calcd for C34H34N2 (%): C, 86.77; H, 7.28; N, 5.95. Found: C, 86.66; H, 7.27; N, 5.90. HRMS (m/z): calcd for C34H34N2: 470.27; found: 470.30 [M + H]+.
L3. Yield 35.2%. 1H NMR (400 MHz, THF-d8, δ, ppm): 8.26 (d, J = 7.4 Hz 1H, ArH), 7.88 (dd, J = 13.2, 7.9 Hz, 2H, ArH), 7.59 (m, 1H, ArH), 7.51 (t, J = 7.5 Hz, 1H, ArH), 7.30 (m, 1H, ArH), 7.09–7.01 (m, 4H, ArH), 6.96–6.81 (m, 5H, ArH), 6.63 (d, J = 7.9 Hz, 1H, ArH), 1.25 (s, 9H, C(CH3)3), 1.13 (s, 9H, C(CH3)3). 13C NMR (100 MHz, THF-d8, δ, ppm): 159.82, 146.57, 144.91, 135.56, 135.40, 135.11, 134.76, 124.49, 131.78, 131.05, 129.42, 129.10, 128.77, 127.40, 127.04, 124.07, 123.47, 123.41, 123.37, 122.50, 58.23, 54.59, 28.30, 27.25. Anal. calcd for C34H34N2 (%): C, 86.77; H, 7.28; N, 5.95. Found: C, 86.69; H, 7.23; N, 5.89. HRMS (m/z): calcd for C34H34N2: 470.27; found: 470.20 [M + H]+.
Synthesis of Mg complexes
A mixture of pro-ligand (10 mmol) and magnesium powder (50 mmol) activated with iodine in tetrahydrofuran (50 mL) was stirred for ca. 1 h in a glovebox at RT. The deep red solid complex was isolated through filtering and removing the solvent under vacuum.
1. Yield 94.5%. 1H NMR (400 MHz, THF-d8, δ, ppm): 8.38 (d, J = 7.4 Hz, 1H, ArH), 8.25 (t, J = 7.6 Hz, 2H, ArH), 7.62 (t, J = 7.5 Hz, 1H, ArH), 7.58–7.45 (m, 2H, ArH), 7.39 (t, J = 7.9 Hz, 1H, ArH), 7.12–6.95 (m, 9H, ArH), 6.91–6.82 (m, 2H, ArH), 3.61 (bs, 12H, α-CH2(THF)), 1.75 (bs, 12H, β-CH2(THF)). 13C NMR (100 MHz, THF-d8, δ, ppm): 162.00, 135.21, 134.05, 132.77, 130.56, 129.85, 129.43, 129.22, 128.87, 128.33, 127.92, 127.80, 127.64, 126.39, 125.91, 125.72, 124.98, 124.40, 124.03, 123.88, 123.44, 69.02 (α-CH2 (THF)), 26.43 (β-CH2(THF)). Anal. calcd for C38H42MgN2O3 (%): C, 76.19; H, 7.07; N, 4.68. Found: C, 76.24; H, 7.11; N, 4.75. M.p. 189 °C.
2. Yield 91.0%. 1H NMR (400 MHz, THF-d8, δ, ppm): 8.26 (d, J = 7.5 Hz, 1H, ArH), 8.01 (t, J = 8.1 Hz, 2H, ArH), 7.59 (t, J = 7.4 Hz, 1H, ArH), 7.53–7.42 (m, 2H, ArH), 7.36 (t, J = 7.5 Hz, 1H, ArH), 6.99 (d, J = 7.4 Hz, 2H, ArH), 6.94–6.64 (m, 3H, ArH), 3.58 (bs, 12H, α-CH2 (THF)), 2.52–2.18 (m, 4H, CH2CH3), 1.73 (bs, 12H, β-CH2(THF)), 1.58 (dt, J = 15.3, 7.7 Hz, 4H, CH2CH3), 1.05 (t, J = 7.4 Hz, 6H, CH2CH3), 0.71 (t, J = 7.4 Hz, 6H, CH2CH3). 13C NMR (100 MHz, THF-d8, δ, ppm): 159.65, 132.49, 132.11, 131.12, 129.92, 129.44, 128.89, 128.55, 128.27, 128.07, 127.83, 127.7, 127.55, 126.10, 125.81, 125.42, 124.43, 124.15, 123.91, 123.26, 122.95, 68.34 (α-CH2(THF)), 28.73, 26.05 (β-CH2(THF)), 23.96, 13.39, 13.32. Anal. calcd for C46H58MgN2O3 (%): C, 77.68; H, 8.22; N, 3.94. Found: C, 77.65; H, 88.19; N, 3.89. M.p. 213 °C. A crystal of 2 suitable for X-ray structural determination was grown in tetrahydrofuran solution.†
3. Yield 87.3%. 1H NMR (400 MHz, THF-d8, δ, ppm): 8.24 (d, J = 7.8 Hz, 1H, ArH), 7.94 (t, J = 7.9 Hz, 2H, ArH), 7.57 (m, 1H, ArH), 7.50–7.38 (m, 2H, ArH), 7.36 (t, J = 7.8 Hz, 1H, ArH), 6.96–6.60 (m, 5H, ArH), 3.55 (bs, 12H, α-CH2(THF)), 1.70 (bs, 12H, β-CH2(THF)), 1.23 (s, 9H, C(CH3)3), 1.10 (s, 9H, C(CH3)3). 13C NMR (100 MHz, THF-d8, δ, ppm): 159.45, 133.00, 132.54, 131.03, 129.83, 129.35, 128.71, 128.48, 128.12, 128.00, 127.56, 127.66, 127.48, 126.01, 125.59, 125.33, 124.37, 124.26, 123.75, 123.11, 122.77, 66.87 (α-CH2(THF)), 29.02, 28.95, 26.05 (β-CH2(THF)). Anal. calcd for C44H54MgN2O3 (%): C, 77.35; H, 7.97; N, 4.10. Found: C, 77.41; H, 8.00; N, 4.14. M.p. 227 °C.
General procedure for lactide polymerization
In a typical polymerization reaction: magnesium complex (0.4 mmol) and isopropanol (0.4 mmol) in toluene (80 mL) were added in a flame-dried ampule including a magnetic stir bar. The ampule was placed in an oil bath at 70 °C. The solution was stirred for ca. 10 min, when the catalyst was activated by isopropanol and whereafter the selected quantity of lactide was added. The polymer was isolated by precipitating with cold methanol or refrigerated centrifuge, after the required length of reaction time. The solid was attained and dried in vacuo at 30 °C for 40 h.
Conclusions
In conclusion, three magnesium complexes 1–3 were synthesized in moderate yields from the reactions of magnesium with Schiff bases containing phenanthrene derivatives. These complexes were employed as catalysts for the polymerization of L-LA and rac-LA. Bulky substituents on the ligand affect the tacticities of the polymers. Microstructural analysis of the polymers catalyzed by these complexes revealed that the Schiff base ligands have a certain ability to affect the tacticity of the polymer, and this ability varies according to ligand bulk. In addition, kinetic analysis indicated that polymerizations of lactide by complex 2 are first-order with respect to monomer, and have an order of 0.97 with respect to catalyst.
Acknowledgements
This work was supported by the National Natural Science Foundation of China (nos 21204082, 51173183, 51021003, 51321062); the Ministry of Science and Technology of China (no. 2011AA02A202); Jilin Science & Technology Department, Science and Technology Development Project (no. 20140204017GX); Science and Technology Bureau of Changchun City (no. 2013060).
Notes and references
-
(a) R. Langer and J. P. Vacanti, Science, 1993, 260, 920–926 CAS;
(b) K. E. Uhrich, S. M. Cannizzaro, R. S. Langer and K. M. Shakesheff, Chem. Rev., 1999, 99, 3181–3198 CrossRef CAS PubMed;
(c) A. M. DiCiccio and G. W. Coates, J. Am. Chem. Soc., 2011, 133, 10724–10727 CrossRef CAS PubMed;
(d) M. J. Stanford and A. P. Dove, Chem. Soc. Rev., 2010, 39, 486–494 RSC;
(e) C. M. Thomas, Chem. Soc. Rev., 2010, 39, 165–173 RSC;
(f) D. Q. Wu, X. Z. Zhang and C. C. Chu, Am. J. Drug Delivery, 2005, 3, 253–267 CrossRef CAS;
(g) R. M. Thomas, P. C. Widger, S. M. Ahmed, R. C. Jeske, W. Hirahata, E. B. Lobkovsky and G. W. Coates, J. Am. Chem. Soc., 2010, 132, 16520–16525 CrossRef CAS PubMed;
(h) M. H. Chisholm, J. C. Gallucci and K. Phomphrai, Inorg. Chem., 2005, 44, 22 Search PubMed.
-
(a) W. Chen, H. C. Yang, R. Wang, R. Cheng, F. H. Meng, W. X. Wei and Z. Y. Zhong, Macromolecules, 2010, 43, 201–207 CrossRef CAS;
(b) A. Kowalski, A. Duda and S. Penczek, Macromolecules, 2000, 33, 689–695 CrossRef CAS;
(c) R. E. Drumright, P. R. Gruber and D. E. Henton, Adv. Mater., 2000, 12, 1841–1846 CrossRef CAS;
(d) D. Sykes and M. D. Ward, Chem. Commun., 2011, 47, 2279–2281 RSC;
(e) X. Song, F. Ling and X. S. Chen, Acta Polym. Sin., 2013, 1, 95–101 Search PubMed;
(f) R. J. Cox and P. S. H. Wang, J. Chem. Soc., Perkin Trans. 1, 2001, 2006–2008 RSC.
-
(a) E. E. Schmitt, R. A. Polistina, U.S. Patent 3,463, 158, 1969;
(b) K. M. Stridsberg, M. Ryner and A. C. Albertsson, Adv. Polym. Sci., 2002, 157, 41–65 CrossRef CAS;
(c) M. Lahcini, P. M. Castro, M. Kalmi, M. Leskelä and T. Repo, Organometallics, 2004, 23, 4547–4549 CrossRef CAS;
(d) A. Tullo, Chem. Eng. News, 2000, 3, 13–15 Search PubMed;
(e) W. Chen, H. C. Yang, R. Wang, R. Cheng, F. H. Meng, W. X. Wei and Z. Y. Zhong, Macromolecules, 2010, 43, 201–207 CrossRef CAS.
-
(a) R. K. Iha, K. L. Wooley, A. M. Nyström, D. J. Burke, M. J. Kade and C. J. Hawker, Chem. Rev., 2009, 109, 5620–5686 CrossRef CAS PubMed;
(b) J. M. Leiston-Belanger, T. P. Russell, E. Drockenmuller and C. J. Hawker, Macromolecules, 2005, 38, 7676–7683 CrossRef CAS;
(c) A. Alaaeddine, C. M. Thomas, T. Roisnel and J. F. Carpentier, Organometallics, 2009, 28, 1469–1475 CrossRef CAS;
(d) C.-T. Chen, C.-A. Huang and B.-H. Huang, Dalton Trans., 2003, 3799–3803 RSC;
(e) N. Iwasaa, M. Fujiki and K. Nomura, J. Mol. Catal. A: Chem., 2008, 292, 67–75 CrossRef;
(f) Y. Wang and H. Y. Ma, Chem. Commun., 2012, 48, 6729–6731 RSC;
(g) W. J. Zhang, Y. H. Wang, W.-H. Sun, L. Wang and C. Redshaw, Dalton Trans., 2012, 41, 11587–11596 RSC;
(h) Z. Liu, W. Gao, J. Zhang, D. M. Cui, Q. Wu and Y. Mu, Organometallics, 2010, 29, 5783–5790 CrossRef CAS;
(i) C. Robert, F. Montigny and C. M. Thomas, Nat. Commun., 2011, 2, 586 CrossRef PubMed.
-
(a) G. Labourdette, D. J. Lee, B. O. Patrick, M. B. Ezhova and P. Mehrkhodavandi, Organometallics, 2009, 28, 1309–1319 CrossRef CAS;
(b) C. A. Wheaton, B. J. Ireland and P. G. Hayes, Organometallics, 2009, 28, 1282–1285 CrossRef CAS;
(c) V. Poirier, T. Roisnel, J.-F. Carpentier and Y. Sarazin, Dalton Trans., 2009, 9820–9827 RSC;
(d) C. K. Williams, L. E. Breyfogle, S. K. Choi, W. Nam, V. G. Young, Jr, M. A. Hillmyer and W. B. Tolman, J. Am. Chem. Soc., 2003, 125, 11350–11359 CrossRef CAS PubMed;
(e) E. L. Whitelaw, G. Loraine, M. F. Mahon and M. D. Jones, Dalton Trans., 2011, 40, 11469–11473 RSC;
(f) A. J. Nijenhuis, D. W. Grijpma and A. J. Pennings, Macromolecules, 1992, 25, 6419–6424 CrossRef CAS;
(g) J. Ejfler, S. Szafert, K. Mierzwicki, L. B. Jerzykiewicz and P. Sobota, Dalton Trans., 2008, 6556–6562 RSC;
(h) R. R. Gowda and D. Chakraborty, J. Mol. Catal. A: Chem., 2010, 333, 167–172 CrossRef CAS;
(i) X. Pang, X. S. Chen, X. L. Zhuang and X. B. Jing, J. Polym. Sci., Part A: Polym. Chem., 2008, 46, 643–649 CrossRef CAS;
(j) Y.-E. Tai, C.-Y. Li, C.-H. Lin, Y.-C. Liu, B.-T. Ko and Y.-S. Sun, J. Polym. Sci., Part A: Polym. Chem., 2011, 49, 4027–4036 CrossRef CAS.
-
(a) H.-Y. Tang, H.-Y. Chen, J.-H. Huang and C.-C. Lin, Macromolecules, 2007, 40, 8855–8860 CrossRef CAS;
(b) J.-C. Wu, Y.-Z. Chen, W.-C. Hung and C.-C. Lin, Organometallics, 2008, 27, 4970–4978 CrossRef CAS;
(c) L. F. Sánchez-Barba, D. L. Hughes, S. M. Humphrey and M. Bochmann, Organometallics, 2006, 25, 1012–1020 CrossRef;
(d) I. J. Blackmore, V. C. Gibson, P. B. Hitchcock, C. W. Rees, D. J. Williams and A. J. P. White, J. Am. Chem. Soc., 2000, 122, 11845–11854 CrossRef;
(e) I. L. Fedushkin, A. G. Morozov, V. A. Chudakova, G. K. Fukin and V. K. Cherkasov, Eur. J. Inorg. Chem., 2009, 4995–5003 CrossRef CAS;
(f) L. Wang and H. Ma, Macromolecules, 2010, 43, 6535–6537 CrossRef CAS.
-
(a) J. B. Chen, J. L. Gorczynski, G. Q. Zhang and C. L. Fraser, Macromolecules, 2010, 43, 4909–4920 CrossRef CAS;
(b) D. S. Mcguinness, E. L. Marshall, V. C. Gibson and J. W. Steed, J. Polym. Sci., Part A: Polym. Chem., 2003, 41, 3798–3803 CrossRef CAS.
-
(a) D. Takeuchi and T. Aida, Macromolecules, 2000, 33, 4607–4609 CrossRef CAS;
(b) Y. Kim and J. G. Verkade, Organometallics, 2002, 21, 2395–2399 CrossRef CAS;
(c) Y. Takashima, Y. Nakayama, K. Watanabe, T. Itono, N. Ueyama, A. Nakamura, H. Yasuda, A. Harada and J. Okuda, Macromolecules, 2002, 35, 7538–7544 CrossRef CAS;
(d) Y. Kim and J. G. Verkade, Macromol. Rapid Commun., 2002, 23, 917–921 CrossRef CAS;
(e) Y. Kim, P. N. Kapoor and J. G. Verkade, Inorg. Chem., 2002, 41, 4834–4838 CrossRef CAS PubMed;
(f) H. Y. Chen, M. Y. Liu, A. K. Sutar and C.-C. Lin, Inorg. Chem., 2010, 49, 665–674 CrossRef CAS PubMed;
(g) J. Okuda and I. L. Rushkin, Macromolecules, 1993, 26, 5530–5532 CrossRef CAS;
(h) E. L. Whitelaw, M. D. Jones, M. F. Mahon and G. Kociok-Kohn, Dalton Trans., 2009, 9020–9025 RSC.
-
(a) A. F. Douglas, B. O. Patrick and P. Mehrkhodavandi, Angew. Chem., Int. Ed., 2008, 47, 2290–2293 CrossRef CAS PubMed;
(b) I. Yu, A. Acosta-Ramírez and P. Mehrkhodavandi, J. Am. Chem. Soc., 2012, 134, 12758–12773 CrossRef CAS PubMed;
(c) A. Pietrangelo, S. C. Knight, A. K. Gupta, L. J. Yao, M. A. Hillmyer and W. B. Tolman, J. Am. Chem. Soc., 2010, 132, 11649–11657 CrossRef CAS PubMed.
-
(a) B. Liu, T. Roisnel, L. Maron, J.-F. Carpentier and Y. Sarazin, Chem.–Eur. J., 2013, 19, 3986–3994 CrossRef CAS PubMed;
(b) S. Marks, J. G. Heck, M. H. Habicht, P. OñaBurgos, C. Feldmann and P. W. Roesky, J. Am. Chem. Soc., 2012, 134, 16983–16986 CrossRef CAS PubMed;
(c) P. Benndorf, J. Kratsch, L. Hartenstein, C. M. Preuss and P. W. Roesky, Chem.–Eur. J., 2012, 18, 14454–14463 CrossRef CAS PubMed;
(d) J. Wang, Y. Yao, Y. Zhang and Q. Shen, Inorg. Chem., 2009, 48, 744–751 CrossRef CAS PubMed;
(e) H. Ma, T. P. Spaniol and J. Okuda, Inorg. Chem., 2008, 47, 3328–3339 CrossRef CAS PubMed;
(f) B. Liu, D. Cui, J. Ma, X. Chen and X. Jing, Chem.–Eur. J., 2007, 13, 834–845 CrossRef CAS PubMed;
(g) X. Liu, X. Shang, T. Tang, N. Hu, F. Pei, D. Cui, X. Chen and X. Jing, Organometallics, 2007, 26, 2747–2757 CrossRef CAS;
(h) I. Westmoreland and J. Arnold, Dalton Trans., 2006, 4155–4163 RSC;
(i) G. Giesbrecht, G. D. Whitener and J. Arnold, J. Chem. Soc., Dalton Trans., 2001, 923–927 RSC.
- R. H. Platel, L. M. Hodgson and C. K. Williams, Polym. Rev., 2008, 48, 11–63 CrossRef CAS.
-
(a) R. A. Gross, A. Kumar and B. Kalra, Chem. Rev., 2001, 101, 2097–2124 CrossRef CAS PubMed;
(b) O. Dechy-Cabaret, B. Martin-Vaca and D. Bourissou, Chem. Rev., 2004, 104, 6147–6176 CrossRef CAS PubMed;
(c) N. E. Kamber, W. Jeong, R. M. Waymouth, R. C. Pratt, B. G. G. Lohmeijer and J. L. Hedrick, Chem. Rev., 2007, 107, 5813–5840 CrossRef CAS PubMed.
-
(a) N. Spassky, M. Wisniewski, C. Pluta and A. L. Borgne, Macromol. Chem. Phys., 1996, 197, 2627 CrossRef CAS;
(b) T. M. Ovitt and G. W. Coates, J. Am. Chem. Soc., 2002, 124, 1316 CrossRef CAS PubMed;
(c) C. P. Radano, G. L. Baker and M. R. Smith, J. Am. Chem. Soc., 2000, 122, 1552 CrossRef CAS;
(d) Z. Y. Zhong, P. J. Dijkstra and J. Feijen, Angew. Chem., 2002, 114, 4692 (Angew. Chem., Int. Ed., 2002, 41, 4510) CrossRef;
(e) H. Du, A. H. Velders, P. J. Dijkstra, J. Sun, Z. Zhong, X. S. Chen and J. Feijen, Chem.–Eur. J., 2009, 15, 9836–9845 CrossRef CAS PubMed;
(f) N. Nomura, R. Ishii, M. Akakura and K. Aoi, J. Am. Chem. Soc., 2002, 124, 5938 CrossRef CAS PubMed;
(g) N. Nomura, R. Ishii, Y. Yamamoto and T. Kondo, Chem.–Eur. J, 2007, 13, 4433 CrossRef CAS PubMed;
(h) K. Majerska and A. Duda, J. Am. Chem. Soc., 2004, 126, 1026 CrossRef CAS PubMed;
(i) Y. Sarazin, B. Liu, T. Roisnel, L. Maron and J.-F. Carpentier, J. Am. Chem. Soc., 2011, 133, 9069–9087 CrossRef CAS PubMed;
(j) I. Yu, A. Acosta-Ramírez and P. Mehrkhodavandi, J. Am. Chem. Soc., 2012, 134, 12758–12773 CrossRef CAS PubMed.
-
(a) H. Du, A. H. Velders, P. J. Dijkstra, Z. Y. Zhong, X. S. Chen and J. Feijen, Macromolecules, 2009, 42, 1058–1066 CrossRef CAS;
(b) Z. H. Tang, X. S. Chen, X. Pang, Y. K. Yang, X. F. Zhang and X. B. Jing, Biomacromolecules, 2004, 5, 965–970 CrossRef CAS PubMed;
(c) X. Pang, H. Z. Du, X. S. Chen, X. Wang and X. B. Jing, Chem.–Eur. J., 2008, 14, 3126–3136 CrossRef CAS PubMed;
(d) Z. H. Tang, X. S. Chen, Y. K. Yang, X. Pang, J. R. Sun, X. F. Zhang and X. B. Jing, J. Polym. Sci., Part A: Polym. Chem., 2004, 42, 5974–5982 CrossRef CAS;
(e) X. Pang, H. Z. Du, X. S Chen, X. L. Zhuang, D. M. Cui and X. B. Jing, J. Polym. Sci., Part A: Polym. Chem., 2005, 43, 6605–6612 CrossRef CAS;
(f) X. Pang, R. L. Duan, X. Li and X. S. Chen, Polym. Chem., 2014, 5, 3894–3900 RSC;
(g) X. Pang, R. L. Duan, X. Li, Z. Sun, H. Zhang, X. H. Wang and X. S. Chen, Polym. Chem., 2014, 5, 6857–6864 RSC;
(h) X. Pang, R. L. Duan, X. Li, B. Gao, Z. Sun, X. H. Wang and X. S. Chen, RSC Adv., 2014, 4, 22561–22566 RSC.
-
(a) H. K. Jr. Hall, A. B. Padias, P. A. Williams, J. Gosau, H. W. Boone and D. Park, Macromolecules, 1995, 28, 1–8 CrossRef;
(b) M. Jeon and S. Y. Kim, Polym. J., 2008, 40, 409–413 CrossRef CAS.
-
(a) A. W. Addison, T. N. Rao, J. Reedijk, J. Rijn and G. C. Verschoor, J. Chem. Soc., Dalton Trans., 1984, 1349 RSC.
-
(a) A. Scarel, M. R. Axet, F. Amoroso, F. Ragaini, C. J. Elsevier, A. Holuigue, C. Carfagna, L. Mosca and B. Milani, Organometallics, 2008, 27, 1486 CrossRef CAS;
(b) M. Gasperini, F. Ragaini, E. Gazzola, A. Caselli and P. Macchi, Dalton Trans., 2004, 3376 RSC.
- M.-A. Munoz-Hernandez, T. S. Keizer, P. Wei, S. Parkin and D. A. Atwood, Inorg. Chem., 2001, 40, 6782–6787 CrossRef CAS PubMed.
- I. L. Fedushkin, A. A. Skatova, V. A. Chudakova, G. K. Fukin, S. Dechert and H. Schumann, Eur. J. Inorg. Chem., 2003, 3336 CrossRef CAS.
- J. Baran, A. Duda, A. Kowalski, R. Szymanski and S. Penczek, Macromol. Rapid Commun., 1997, 18, 325–333 CrossRef CAS.
- K. A. M. Thakur, R. T. Kean, E. S. Hall, J. J. Kolstad, T. A. Lingren, M. A. Doscotch, J. I. Siepmann and E. J. Munson, Macromolecules, 1997, 30, 2422–2428 CrossRef CAS.
- Pr is the probability of racemic linkages between monomer units and is determined from the methane region of the homonuclear decoupled 1H NMR spectrum (Pr + Pm = 1). The expressions for the tetrad concentrations in terms of Pr, assuming Bernoullian statistics and the absence of transesterification, are as follows: [mmm] = (2(1 − Pr)2 + Pr(1 − Pr))/2; [mrm] = (Pr2 + Pr(1 − Pr))/2; [mmr] = [rmm] = (Pr(1 − Pr))/2; [rmr] = Pr2/2. See: B. M. Chamberlain, M. Cheng, D. R. Moore, T. M. Ovitt, E. B. Lobkovsky and G. W. Coates, J. Am. Chem. Soc., 2001, 123, 3229–3238 CrossRef CAS PubMed.
-
(a) R. J. Cox, D. J. Ritson, T. A. Dane, J. Berge, J. P. H. Charmant and A. Kantacha, Chem. Commun., 2005, 1037–1039 RSC;
(b) A. Fahad, A. Abood, K. M. Fisch, A. Osipow, J. Davison, M. Avramović, C. P. Butts, J. Piel, T. J. Simpson and R. J. Cox, Chem. Sci., 2014, 5, 523–527 RSC;
(c) A. Yakasai, J. Davison, Z. Wasil, L. M. Halo, C. P. Butts, C. M. Lazarus, A. M. Bailey, T. J. Simpson and R. J. Cox, J. Am. Chem. Soc., 2011, 133, 10990–10998 CrossRef CAS PubMed;
(d) R. J. Cox and A. Al-Fahad, Curr. Opin. Chem. Biol., 2013, 17, 532–536 CrossRef CAS PubMed;
(e) R. J. Cox, T. S. Hitchman, K. J. Byrom, I. S. Findlow, J. A. Tanner, J. Crosby and T. J. Simpson, FEBS Lett., 1997, 405, 267–272 CrossRef CAS PubMed;
(f) R. J. Cox, Nat. Prod. Rep., 1996, 13, 29–43 RSC.
-
(a) I. S. Tidmarsh, T. B. Faust, H. Adams, L. P. Harding, L. Russo, W. Clegg and M. D. Ward, J. Am. Chem. Soc., 2008, 130, 15167–15175 CrossRef CAS PubMed;
(b) M. Whitehead, S. Turega, A. Stephenson, C. A. Hunter and M. D. Ward, Chem. Sci., 2013, 4, 2744–2751 RSC;
(c) A. Stephenson, D. Sykes and M. D. Ward, Dalton Trans., 2013, 42, 6756–6767 RSC;
(d) A. H. Shelton, I. V. Sazanovich, J. A. Weinstein and M. D. Ward, Chem. Commun., 2012, 48, 2749–2751 RSC;
(e) D. Sykes, I. S. Tidmarsh, A. Barbieri, I. V. Sazanovich, J. A. Weinstein and M. D. Ward, Inorg. Chem., 2011, 50, 11323–11339 CrossRef CAS PubMed;
(f) A. Stephenson, S. P. Argent, T. Riis-Johannessen, I. S. Tidmarsh and M. D. Ward, J. Am. Chem. Soc., 2011, 133, 858–870 CrossRef CAS PubMed.
- G. M. Sheldrick, SHELXTL, Version 5.1, Siemens Industrial Automation, Inc., 1997 Search PubMed.
Footnote |
† Electronic supplementary information (ESI) available: X-ray crystallographic data and refinement for complex 2 in CIF format. CCDC 1029408. For ESI and crystallographic data in CIF format see DOI: 10.1039/c4ra12548g |
|
This journal is © The Royal Society of Chemistry 2015 |
Click here to see how this site uses Cookies. View our privacy policy here.