DOI:
10.1039/D0QM00536C
(Research Article)
Mater. Chem. Front., 2021,
5, 406-417
Small organic molecule-based nanoparticles with red/near-infrared aggregation-induced emission for bioimaging and PDT/PTT synergistic therapy†
Received
27th July 2020
, Accepted 28th September 2020
First published on 29th September 2020
Abstract
Organic nanomaterials with efficient ROS generation and high photothermal conversion efficiency have emerged as a new generation therapeutic agent for PDT/PTT synergistic cancer treatment. However, most of the organic nanomaterials exhibited weak emission in water because of the aggregation-caused quenching (ACQ) effect, which seriously hampers their biological application in fluorescence bioimaging. In this study, we design and synthesize a new organic small molecule (T-BDP) with a donor–acceptor–donor (D–A–D) structure by the conjugation of boron-dipyrromethene (BODIPY) and triphenylamine (TPA). Moreover, a 1,8-naphthalenediimide (NI) structure with electron-withdrawing ability is introduced onto the core of BODIPY to further enhance the intramolecular charge transfer, leading to the redshift of absorption to the near-infrared region. T-BDP displays significant aggregation-induced emission (AIE) performance, probably due to the presence of two twisted TPA groups onto the BODIPY core. Accordingly, after self-assembly into nanoparticles, T-BDP NPs exhibit strong near-infrared emission in water. Under single 635 nm laser irradiation, T-BDP NPs could generate reactive oxygen species and heat simultaneously. The photothermal conversion efficiency of T-BDP NPs is determined to be 50.9%. The low dark toxicity and high photocytotoxicity of T-BDP NPs are verified against A549 cells using the MTT assay and the AM/PI staining method. Due to the strong emission of T-BDP NPs, their accumulation and subcellular localization in cancer cells are observed using a laser confocal fluorescence microscope. The results demonstrate that T-BDP NPs are mainly located in the lysosomes of cancer cells. Thus, the as-prepared small molecule-based AIE nanoparticles hold great potential for fluorescence imaging-guided PDT/PTT synergistic tumor therapy.
Introduction
Phototherapeutic approaches, including photodynamic therapy (PDT) and photothermal therapy (PTT), have attracted intensive attention in cancer treatment due to their promising potential advantages, such as minimal invasiveness, minor side effects, and high efficiency.1–4 For PDT, cancer cells are killed by the reactive oxygen species (ROS) generated by photosensitizers,5–7 which transfer energy to the surrounding molecular oxygen under light irradiation.8–10 Thus, the PDT effect will terminate with the consumption of molecular oxygen in the hypoxic environment of tumor sites.11 As to PTT, the ablation of cancer cells mainly relies on the heat generated by the photothermal agents upon near-infrared light irradiation.12,13 Despite the significant achievements made in individual PDT or PTT,14–19 there is growing interest in combining PDT and PTT in a single system for synergistic therapy.8,20–22 Such synergistic treatment has been recently found to effectively circumvent the limitations of PDT and PTT alone.
To date, most of the photothermal agents are based on inorganic materials, such as carbon nanomaterials, metal sulfide and gold nanoparticles. However, the nonbiodegradable characteristic and long-term cytotoxicity severely limit their further applications in the clinic.23 In contrast, organic materials, especially the small molecules, possess some intrinsic merits such as outstanding biocompatibility, potential biodegradability, well-defined chemical structure, and easy processability, which thus gain extensive interest. However, most of the reported organic molecules are hydrophobic, which limits their biomedical applications in cancer treatment.24,25 Recently, the self-assembly of amphiphilic organic molecules into related nanoparticles is demonstrated to be an efficient way to fabricate organic nanomaterials with improved biocompatibility, high photothermal conversion efficiency,26,27 and reasonable particle size, endowing them with enhanced permeability and retention (EPR) effect.28–31 However, just like a coin has two sides, the π–π stacking of molecules in nanostructures could induce the quenching of fluorescence because of the aggregation-caused quenching (ACQ) effect, which severely hampers their biological applications in fluorescence bioimaging.32
Aggregation-induced emission (AIE) is a kind of phenomenon oppositional to ACQ.33–35 Organic nanoparticles with the AIE effect are promising candidates for fluorescence bioimaging as the brightness of organic nanomaterials can be enhanced by the self-aggregation of molecules inside.36–38 Until now, various AIE nanomaterials with blue, green, and red emission have been successfully developed and applied in bioimaging in vitro and in vivo.39 However, the development of organic AIE nanomaterials with near-infrared emission and phototherapeutic function is still challenging.40,41 Boron-dipyrromethene (BODIPY) is an excellent photosensitizer with high photostability, low cytotoxicity, and excellent PDT effect.42,43 It is also a popular fluorophore that has been widely studied in the field of biomarkers and ion probes because of its strong fluorescence in dilute solution.44,45 Therefore, the design and synthesis of BODIPY-based organic nanoparticles can be used for fluorescence imaging-guided cancer phototherapy.
Herein, we design and synthesize a single-component BODIPY-based nanoparticle for fluorescence imaging and PDT/PTT synergistic therapy. Specifically, triphenylamine (TPA) was conjugated with BODIPY at the 2′ and 6′ positions through triple bonds. Due to the electron-donating characteristic of TPA and the electron-withdrawing ability of BODIPY, the conjugate exhibits a typical donor (D)–acceptor (A)–donor (D) structure, narrowing the bandgap of the organic molecule due to the intramolecular charge transfer (ICT), which further redshifts the absorption to the near-infrared region. Moreover, triphenylamine (TPA) is an important unit for AIE molecules because of its non-planar configuration and three freely rotatable benzene rings. Therefore, the introduction of two twisted TPA groups onto the BODIPY core endows the synthesized molecule with aggregation-induced emission (AIE) performance. 1,8-Naphthalimide also has strong electron-withdrawing ability. Thus, the conjugation of naphthalimide with the BODIPY core can further promote the electron transfer.46,47 To improve the hydrophilicity of the organic molecule, the polyethylene glycol (PEG) chain was introduced onto the hydrophobic BODIPY component. Accordingly, the amphiphilic molecules (T-BDP) could spontaneously self-assemble into related nanoparticles (T-BDP NPs) without adding any extra hydrophilic polymer.11,48 The as-prepared T-BDP NPs have good water solubility and display spherical nanoparticles with an average size of around 200 nm. On comparing with the organic molecule, T-BDP NPs exhibit redshifted absorption and stronger fluorescence in the near-infrared region with a maximum peak at around 724 nm. Under single 635 nm laser irradiation, T-BDP NPs could induce the generation of reactive oxygen species and heat them simultaneously, benefiting the ablation of cancer cells through photodynamic and photothermal effects. The biocompatibility and high photocytotoxicity of T-BDP NPs are verified through the MTT method using A549 cells as a model. Due to its favorable biocompatibility and strong fluorescence in an aqueous solution, T-BDP NPs could be applied as a bioimaging agent in vitro and the confocal microscopy imaging indicates the efficient accumulation of T-BDP NPs in the lysosomes of A549 cells. Therefore, these results demonstrate that the single-component T-BDP NPs could be used as promising nanoagents for both fluorescence imaging and cancer phototherapy.
Results and discussion
Synthesis
Scheme 1 shows the synthetic procedure of the T-BDP molecule and T-BDP NPs. Triphenylamine (TPA) was introduced onto the boron-dipyrromethene (BODIPY) core to form a D–A–D structure. The triple bond between TPA and BODIPY did not affect the performance of AIE49 and could enhance the near-infrared absorption. The PEG chain terminated naphthalimide (NI) group was introduced to improve hydrophilicity and facilitate the self-assembly.50 The amino group-terminated PEG chain (3) was synthesized by Gabriel's amine synthesis (see the ESI†). Aldehyde 5 was obtained by the conjugation of 4-formylphenylboronic acid with bromized NI through a Suzuki coupling reaction. After reacting with pyrrole, followed by the iodinated reaction through the conventional method,51I-BDP was synthesized in medium yield. Finally, T-BDP was obtained in a 52% yield through the Sonogashira coupling reaction between I-BDP and 4-ethynyl triarylamine, using Pd(PPh3)4 and CuI as catalysts. The self-assembly of T-BDP into related nanoparticles (T-BDP NPs) was carried out using the nanoprecipitation method.
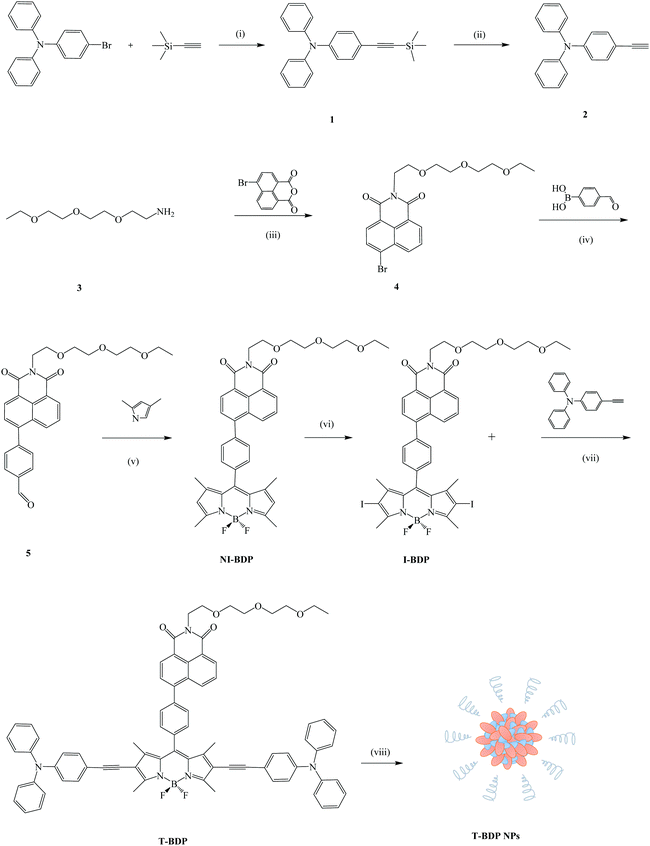 |
| Scheme 1 Synthetic routes of T-BDP and T-BDP NPs. (i) DBU, PdCl2(PPh3)2, CuI, trimethylsilylacetylene, 70 °C, 5 h, 52%; (ii) K2CO3, DCM/MeOH (1 : 1), rt, N2, 76%; (iii) ethanol, 80 °C, 4 h, 80%; (iv) K2CO3, Pd(PPh3)4, THF/H2O (3 : 1), 70 °C, 12 h, 72%; (v) TFA, DDQ, TEA, BF3·Et2O, CH2Cl2, rt, 11.5 h, 22.5%; (vi) NIS, DCM, 30 70 °C, 0.5 h, 90%; (vii) toluene/TEA (5 : 1), Pd(PPh3)4, CuI, 90 °C, 18 h, 52%; (viii) nanoprecipitation. | |
Theoretical calculation
The optimized geometries of T-BDP were performed with Gaussian 09 using the PM6 method. As indicated in Fig. 1a, the BODIPY core was coplanar with an NI structure while it formed a twisted configuration with two triphenylamine fragments. Using density functional theory (DFT) analysis, the theoretical calculations of T-BDP were performed with Gaussian using the B3LYP/STO-3G method. Fig. 1b shows the electron cloud distributions and composition characteristics of the frontier molecular orbitals. The HOMOs mainly located on the triphenylamine fragments and triple bonds while the LUMOs were predominantly distributed on the NI structure and BODIPY core, indicating that the electron transfer from triphenylamine to BODIPY and NI units could occur when T-BDP was excited from the ground states to the excited state. This further verified the D–A–D structure of T-BDP, where the triphenylamine unit acted as an electron donor while BODIPY and NI units act as electron acceptors. The calculated HOMO and LUMO energy levels of T-BDP were at −8.4899 eV and −3.6781 eV, respectively. According to the calculated HOMO and LUMO energy levels, the calculated bandgap for T-BDP was determined to be 4.8118 eV.
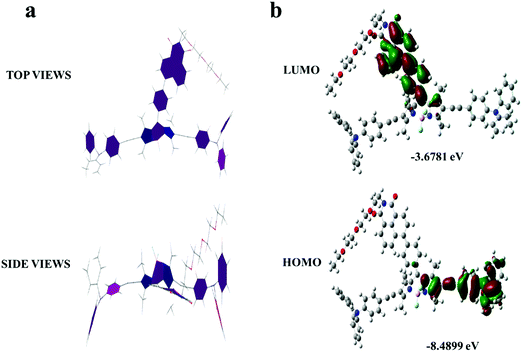 |
| Fig. 1 (a) Optimized geometry of T-BDP; (b) the frontier molecular orbitals of T-BDP. | |
Characterization
As shown in Fig. 2a and b, the nanoparticles had a uniform particle size and good spherical morphology, which facilitated the passive targeting of T-BDP NPs into tumor sites through the EPR effect. Size analysis using DLS showed that the hydrodynamic diameter of T-BDP NPs was 200 nm, which was consistent with the result of transmission electron microscopy (TEM). The zeta potential of T-BDP NPs was determined to be −28.7 mV (Fig. 2c), which was beneficial for the stability of nanoparticles due to electrostatic repulsion.52 In addition, there was no significant change of hydrodynamic diameter of the nanoparticles after storage for 6 months (Fig. 2d), indicating the high stability of T-BDP NPs in aqueous solution.
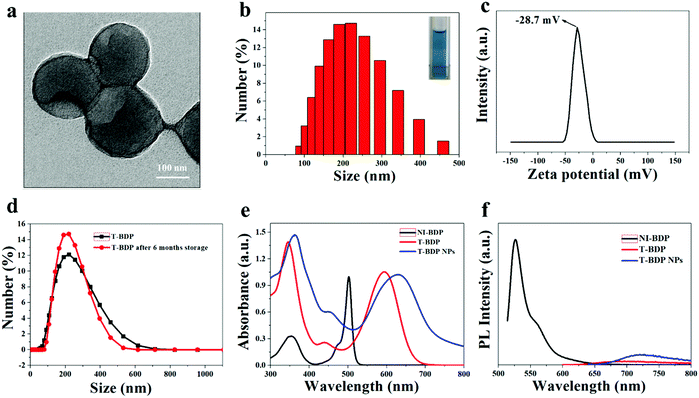 |
| Fig. 2 (a) TEM image of T-BDP NPs; (b) DLS size distribution of T-BDP NPs (insets are the photographs of the aqueous solution of T-BDP NPs); (c) zeta potential of the T-BDP NPs in DI water; (d) dynamic light scattering measurement of T-BDP NPs; (e) UV-vis absorption spectra of NI-BDP and T-BDP in ethanol, and T-BDP NPs in DI water; (f) fluorescence spectra of NI-BDP and T-BDP in ethanol, and T-BDP NPs in DI water at the same concentration. | |
Photophysical properties
The photophysical data of T-BDP and T-BDP NPs are summarized in Table 1.
Table 1 Photophysical properties of T-BDP and T-BDP NPs
Sample |
Absorption peak (nm) |
Emission peak (nm) |
ε (L mol−1 cm−1) |
ϕ
f
|
NI-BDP
|
353, 500 |
520 |
7.07 × 104 |
0.194 |
T-BDP
|
344, 593 |
692 |
3.75 × 104 |
0.0008 |
T-BDP NPs
|
363, 631 |
724 |
4.73 × 104 |
0.0067 |
The absorption spectra of NI-BDP and T-BDP were studied in ethanol solution. Compared to NI-BDP, T-BDP exhibited a broader absorption spectrum in the range of 500–700 nm with the maximum peak red-shifted by 93 nm (Fig. 2e), probably attributed to the conjugation of triphenylamine through triple bonds, extending the π-conjugated system. The remarkable increase in the absorption band at around 350 nm (usually assigned to the S0 → S2 transition) was attributed to the interaction of the peripheral aromatic chains with the chromophoric core. After T-BDP was transformed into NPs, the absorption spectrum became a little broader, along with a bathochromic-shift of around 38 nm, owing to the intermolecular π–π stacking interactions. The redshift of the absorption peak from red to the near-infrared region was crucial for the application of T-BDP NPs in PDT/PTT.
The fluorescence emission of NI-BDP, T-BDP and T-BDP NPs was measured at the same concentration. As shown in Fig. 2f, compared with NI-BDP, the fluorescence of T-BDP was quenched and the Stokes shift increased to nearly 100 nm, which was probably attributed to the enhancement of intramolecular charge transfer (ICT) caused by the D–A–D structure. Both T-BDP and T-BDP NPs showed near-infrared fluorescence. After self-assembly into nanoparticles, the fluorescence of the molecule was significantly enhanced, due to the AIE performance as we expected. The fluorescence quantum yields (QYs) of T-BDP and T-BDP NPs were calculated to be 0.0008 and 0.0067, respectively.
Aggregation-induced fluorescence properties
The emission properties of aggregated T-BDP were then investigated by varying the water fractions (fw) in tetrahydrofuran (THF)/water mixtures. For comparison, NI-BDP was also studied under the same conditions. As shown in Fig. 3a and b, as the content of deionized water in the mixture increased, the fluorescence intensity of NI-BDP continued to decrease. When fw increased to 70%, the original bright yellow-green fluorescence was almost quenched. The fluorescence would not recover with the further addition of water into THF until fw = 100%, possibly due to the ACQ effect. After conjugation with triphenylamine through triple bonds, T-BDP showed significant aggregation-induced emission (AIE) effect. As shown in Fig. 3c and d, with the water content increasing from 0 to 50%, the fluorescence intensity of T-BDP decreased gradually along with the red-shift of the maximum peak. With the further enhancement of the deionized water content, the T-BDP molecules formed nano aggregates, and the fluorescence intensity of the solution was intensified distinctly. In particular, when fw reached 90%, the fluorescence intensity was significantly higher than its original emission intensity in THF, with the emission peak shifting from 692 nm to 724 nm, indicating an obvious AIE phenomenon. Thus, the twisting phenyl rings in TPA enabled the T-BDP molecule to possess an AIE characteristic due to the unique restriction of the intramolecular motion mechanism.
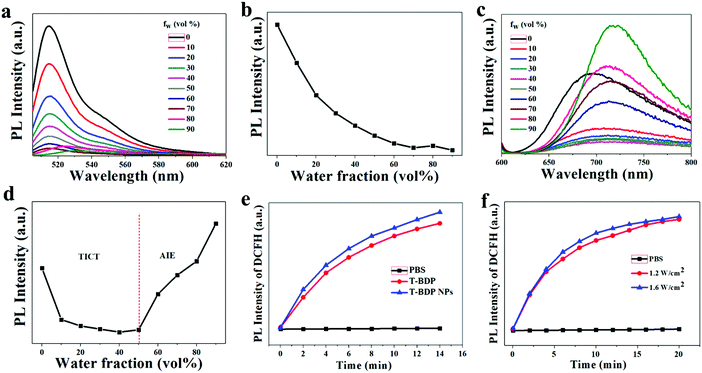 |
| Fig. 3 (a) PL spectra of NI-BDP in THF/water mixtures with different water fractions (fw); (b) diagram of fluorescence intensity change of NI-BDP in the THF/water mixture; (c) PL spectra of T-BDP in THF/water mixtures with different water fractions (fw); (d) diagram of fluorescence intensity change of T-BDP in the THF/water mixture; (e) the changes of fluorescence intensity at the characteristic peak of DCFH (525 nm) as a function of irradiation time for T-BDP and T-BDP NPs at the same concentration upon 635 nm laser irradiation at 1.2 W cm−2; (f) T-BDP NPs upon 635 nm laser irradiation at different laser power densities. | |
ROS generation
The reactive oxygen species (ROS) are cytotoxic and can effectively kill tumor cells. To measure the ROS generation of T-BDP and T-BDP NPs under light irradiation, the DCFH was used a fluorescent probe. DCFH alone has no fluorescence and can be rapidly oxidized by ROS to generate green fluorescent DCF. Therefore, the generation of ROS can be reflected by detecting the fluorescence intensity of DCFH. As shown in Fig. 3e, the fluorescence intensity of DCFH increased with the extension of laser irradiation in the presence of T-BDP or T-BDP NPs, and the ROS generation rate of T-BDP NPs was slightly higher than that of T-BDP. Moreover, the DCFH that mixed with T-BDP NPs showed similar fluorescence intensity enhancement under the irradiation of a 635 nm laser at 1.2 W cm−2 and 1.6 W cm−2 (Fig. 3f), indicating that the power density of the laser has little effect on its ROS generation.
Photochemical and photothermal properties of T-BDP NPs
Because of the efficient absorption in the region of 550–800 nm, the photothermal effect of T-BDP NPs was investigated. Firstly, nanoparticles with different concentrations (0, 25, 50, 100, 150 and 200 μg mL−1) were irradiated with a 635 nm laser (1.2 W cm−2) for 10 min. As shown in Fig. 4a, the temperature elevation of the T-BDP NP solution exhibited concentration-dependent behavior. At the concentration of 100 μg mL−1, the temperature of T-BDP NPs increased by 23 °C. In contrast, the temperature of pure water did not display any obvious variation under the same irradiation. Moreover, the effect of laser power density (1.0, 1.2, 1.4 and 1.6 W cm−2) on the photothermal property of T-BDP NPs was also studied. As shown in Fig. 4b, the maximum temperature of the T-BDP NP solution increased significantly with the laser power density. The photothermal conversion efficiency of T-BDP NPs was then calculated using the reported methods.53Fig. 4c shows the typical temperature change curve of T-BDP NPs at 25 μg mL−1 under 635 nm laser irradiation (1.2 W cm−2) for 10 min. The temperature of the solution increased by 17 °C in 10 min. By linearly fitting the relationship between the negative natural logarithm of the thermal driving force and the cooling time in the cooling curve (Fig. 4d), the time constant was calculated to be 331.5 s. Based on this, the photothermal conversion efficiency (η) of T-BDP NPs was determined to be 50.9%, indicating that T-BDP NPs could be used as a potential photothermal agent in PTT. In addition, the T-BDP NPs can still achieve the initial photothermal effect after five laser on/off cycles (Fig. 4e), and the absorption spectrum did not display any significant change after multiple cycles of irradiation (Fig. 4f), indicating the excellent thermal- and photo-stability of the T-BDP NPs.
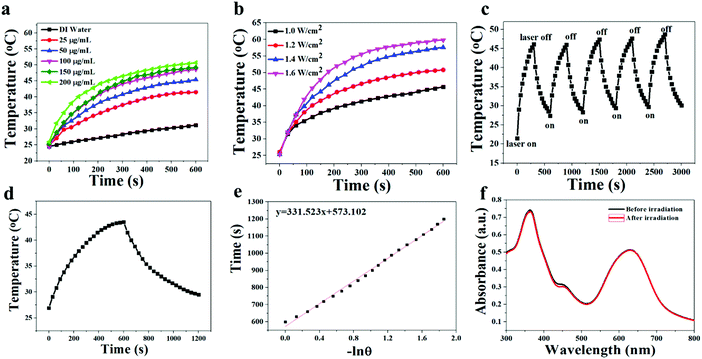 |
| Fig. 4 The photothermal heating curve of T-BDP NPs (a) at different concentrations upon 635 nm laser irradiation at 1.2 W cm−2; (b) at 100 μg mL−1 upon 635 nm laser irradiation at different laser power densities; (c) alternate temperature rising and dropping curve of T-BDP NPs (c = 25 μg mL−1); (d) linear correlation of cooling times versus the negative natural logarithm of driving force temperatures (c = 25 μg mL−1); (e) temperature variations of T-BDP NPs under 635 nm laser irradiation at a power density of 1.2 W cm−2 for five laser on/off cycles (5 min of irradiation for each cycle); (f) absorption spectrum of T-BDP NPs before and after 5 cycles of laser irradiation. | |
Cell viability assay
Encouraged by the high photostability, efficient reactive oxygen species generation, and high photothermal conversion efficiency of T-BDP NPs, their feasibility as nanoagents for cancer phototherapy was evaluated in vitro. The biocompatibility and photocytotoxicity of T-BDP NPs against A549 cells were studied using the standard MTT method. As shown in Fig. 5a, the cells that were incubated with T-BDP NPs in the dark maintained high viability (>90%) even at a high concentration, suggesting the good biocompatibility of T-BDP NPs. In contrast, after the irradiation of a 635 nm laser for 5 min, the survival rate of the cancer cells decreased sharply with the concentration of T-BDP NPs. The cell viability decreased to 11.4% at a concentration of 75 μg mL−1, and the half inhibitory concentration (IC50) was calculated to be 26.5 μg mL−1 under 635 nm laser (0.75 W cm−2) irradiation, indicating that the T-BDP NPs could effectively kill cancer cells through the photo-induced effect. To evaluate the photodynamic/photothermal effects of NPs in vitro, the A549 cells that were incubated with different concentrations of T-BDP NPs were divided into different treatment groups (635 nm laser, 635 nm laser + cooling, and 635 nm laser + VC). Vitamin C (VC) was used as a ROS scavenger to eliminate the PDT effect while ice was used to cool the cells to eliminate the PTT effect. As indicated in Fig. 5b, the cells that were treated with “laser + VC” displayed a lower viability than the cells of the “laser + cooling” group, indicating that the PTT effect played a dominant role in the photocytotoxicity of T-BDP NPs. Furthermore, the “laser” group exhibited a higher photocytotoxicity than the “laser + VC” group, suggesting PTT/PDT synergistic effects of T-BDP NPs upon 635 nm laser irradiation. To further verify the cytocompatibility and high photocytotoxicity of T-BDP NPs, a co-staining experiment was carried out where the living cells were stained with calcein AM (green emission) and the dead cells were stained with propidium iodide (PI) (red emission). As shown in Fig. 5c, all the control groups (PBS only, laser irradiation only, and T-BDP NPs only) displayed green fluorescence, indicating the high survival rate of cancer cells upon these treatments. In contrast, the cancer cells that were treated with T-BDP NPs followed by 635 nm laser irradiation showed obvious red emission, suggesting a high phototherapeutic effect of T-BDP NPs, which agreed well with the results of the MTT assay.
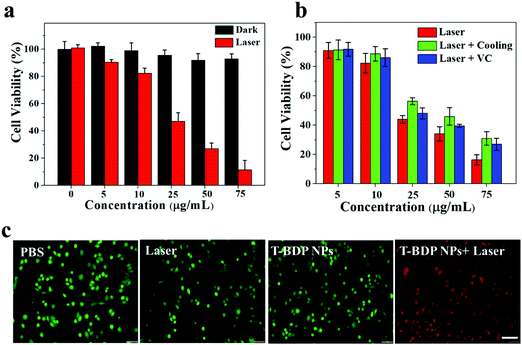 |
| Fig. 5 (a) Viability of A549 cells at different concentrations (0, 5, 10, 25, 50, and 75 μg mL−1) of T-BDP NPs without or with irradiation (635 nm laser, 0.75 W cm−2); (b) viability of A549 cells incubated with various concentrations of T-BDP NPs (5, 10, 25, 50, and 75 μg mL−1) followed by the different laser (635 nm laser, 0.75 W cm−2) treatments (laser, laser + cooling, and laser + VC); (c) fluorescence images of calcein AM (green fluorescence; live cells) and PI (red fluorescence; dead cells) co-stained A549 cells in different treatment groups (PBS only, laser irradiation only, T-BDP NPs only, and T-BDP NPs + laser irradiation) (scale bar = 20 μm). | |
The intracellular ROS generation
To find out whether the ROS played a role in the photocytotoxicity of T-BDP NPs, the A549 cells were co-stained with DCFH-DA, which would be deacetylated by lactonase to form DCFH in living cells. DCFH could react quickly with ROS to obtain a bright green fluorescent DCF, indicating the level of reactive oxygen species. As shown in Fig. 6, a strong green fluorescence was found around the nucleus of cancer cells, which was stained with Hoechst 33342 (blue emission), indicating the efficient generation of ROS in the cytoplasm of tumor cells upon laser irradiation.
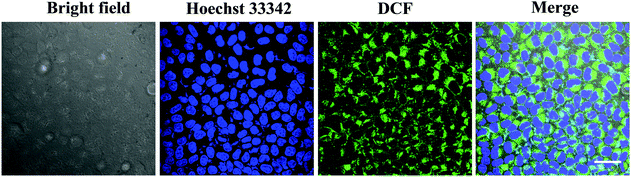 |
| Fig. 6 The ROS generation in A549 cells with DCFH-DA as a probe (the scale bar is 20 μm). | |
Fluorescence imaging and subcellular localization
Considering that the uptake of nanoparticles by tumor cells is critical for efficient cancer phototherapy in vitro and in vivo, we performed the subcellular localization of T-BDP NPs through laser scanning confocal microscopy images. The nucleus and lysosomes of cancer cells were co-stained with Hoechst 33342 and LysoTracker green, respectively. As shown in Fig. 7, the cancer cells that were incubated with T-BDP NPs exhibited intense red fluorescence in the cytoplasm, suggesting that the NPs could be effectively accumulated in tumor cells. Moreover, the red fluorescence was almost overlapped with the green emission from LysoTracker, indicating that the T-BDP NPs were mainly distributed in the lysosomes of cancer cells.
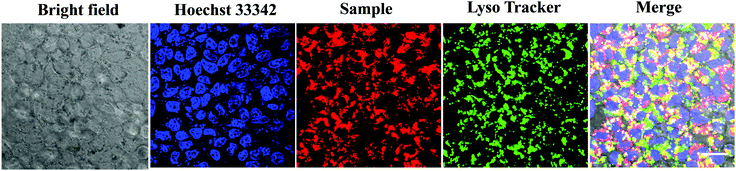 |
| Fig. 7 Laser scanning confocal microscopy image of A549 cells incubated with T-BDP NPs for 4 h (laser excitation at 488 nm). Lysosomes (green) were stained with LysoTracker green (50 μM). Nuclei (blue) were stained with Hoechst 33342 (50 μM) (scale bar = 20 μm). | |
Conclusion
In summary, we designed and synthesized a new BODIPY-based organic molecule (T-BDP) with AIE characteristics. The amphiphilic T-BDP was self-assembled into related nanoparticles (T-BDP NPs) with improved hydrophilicity and enhanced absorbance in the NIR region. Under the irradiation of a single 635 nm laser, T-BDP NPs could generate reactive oxygen species and heat simultaneously with photothermal conversion efficiency as high as 50.9%. T-BDP NPs exhibited strong near-infrared emission in water because of the excellent AIE characteristic. The biocompatibility and photocytotoxicity of T-BDP NPs were verified in vitro against A549 cells. The fluorescence imaging and subcellular localization experiments indicated that T-BDP NPs efficiently accumulated in the lysosome of cancer cells. Thus, the as-prepared T-BDP NPs were demonstrated to be a promising nanoagent with AIE activity and PDT/PTT effect for cancer imaging and treatment.
Experimental section
Chemicals and characterization
All reagents were purchased from Aladdin Corporation (China) and used as received. All solvents were of analytical grade. Dichloromethane and toluene were dried with calcium hydride before use. 1H NMR spectra were recorded using an Agilent 400-MR spectrometer. High resolution mass spectra and mass spectra were obtained using a Bruker Autoflex MALDI-TOF mass spectrometer and a Finnigan TSQ 710 (FAB-MS) mass spectrometer, respectively. Absorption and emission spectra were recorded on a TU-1901 UV-visible spectrophotometer and a Fluoromax-4C-L fluorescence spectrophotometer, respectively. The nanoparticle size was obtained using a JEM-2100 transmission electron microscope (TEM) and a Zetasizer NanoZS 90 system (DLS, Malvern Instruments, Malvern UK), respectively. The fluorescence images were observed on a Confocal Laser Scanning Fluorescence Microscope (FV1200) obtained from Olympus, Japan. The synthesis and characterization of compounds 1–5 are shown in the ESI.†
Synthesis of NI-BDP
A mixture of 2′,4′-dimethylpyrrole (240.0 mg, 2.5 mmol) and PEG-NI-benzaldehyde (466.0 mg, 1.0 mmol) in dichloromethane (120 mL) was stirred at room temperature under a nitrogen atmosphere for 30 min, followed by the addition of 10 μL of TFA. The resultant mixture was stirred at room temperature for another 6 h, followed by the addition of a solution of DDQ (227.0 mg, 1.0 mmol) in CH2Cl2 (30 mL). After stirring for 2 h, Et3N (2.0 mL) was added into the mixture. The resulting mixture was continuously stirred for 5 min, followed by the addition of BF3·Et2O (2.0 mL). After stirring at room temperature for 3 h, the solvent was removed under vacuum. The residue was purified via silica gel column chromatography using CH2Cl2 as an eluent to obtain a reddish-orange crystal (117 mg, 22.5%). 1H-NMR (400 MHz, CDCl3): δ (ppm) 8.67 (s, J = 7.7 Hz, 2H, Ar
), 8.18 (d, J = 7.9 Hz, 1H, Ar
), 7.75 (dd, J = 18.1 Hz, 2H, Ar
), 7.65 (d, J = 7.9 Hz, 2H, Ar
), 7.49 (d, J = 8.0 Hz, 2H, Ar
), 6.04 (s, 2H, CC
C), 4.46 (t, J = 6.0 Hz, 2H, OC
2), 3.89–3.42 (m, 12H, OC
2), 2.57 (s, 6H, C
3), 1.54 (s, 6H, C
3), 1.16 (t, J = 7.0 Hz, 3H,CH2C
3). MALDI-TOF MS: calcd for: 679.3010, found: 679.3014.
Synthesis of I-BDP
NI-BDP (100 mg, 0.15 mmol) and NIS (83 mg, 0.45 mmol) were dissolved in anhydrous CH2Cl2 (15 mL). After stirring at 30 °C for 0.5 h, the resulting mixture was washed with a saturated solution of Na2SO3. The organic fraction was extracted with DCM and then concentrated under reduced pressure. The residue was purified via silica gel column chromatography to obtain a red solid (125 mg, 90%). 1H-NMR (400 MHz, CDCl3): δ (ppm) 8.67 (d, J = 4.7 Hz, 2H, Ar
), 8.17 (d, J = 7.9 Hz, 1H, Ar
), 7.73 (dd, J = 35.7 Hz, 4H, Ar
), 7.47 (d, J = 7.3 Hz, 2H, Ar
), 4.48 (d, J = 4.9 Hz, 2H, OC
2), 3.93–3.38 (m, 12H, OC
2), 2.66 (s, 6H, C
3), 1.56 (s, 6H, C
3), 1.17 (dd, J = 7.7 Hz, 3H, CH2C
3). MS (ESI): m/z = 930.22 [M]+; 804.51 [M − I]+.
Synthesis of T-BDP
NI-BDP (50 mg, 0.05 mmol) was dissolved in 12 mL dry toluene/triethylamine mixture (5
:
1). 4-Ethynyltriphenylamine (0.08 g, 0.28 mmol) was then added and the solution was deaerated with nitrogen for 15 min. The coupling reaction was initiated by the addition of a catalytic amount of Pd(PPh3)4 (7 mg, 6 μmol) and CuI (1.5 mg, 8 μmol). After being refluxed under nitrogen for 18 h, the reaction mixture was concentrated under vacuum. The residue was then purified via silica gel column chromatography to yield a dark blue solid (30 mg, 52%). 1H-NMR (400 MHz, CDCl3): δ (ppm) 8.73–8.63 (m, 2H, Ar
), 8.19 (d, J = 8.2 Hz, 1H, Ar
), 7.82–7.66 (m, 4H, Ar
), 7.52 (d, J = 8.1 Hz, 2H, Ar
), 7.37–7.26 (m, 9H, Ar
), 7.14–6.95 (m, 15H, Ar
), 4.48 (s, 2H, OC
2), 3.86–3.54 (m, 12H, OC
2), 2.73 (s, 6H, C
3), 1.68 (s, 6H, C
3), 1.17 (t, J = 7.0 Hz, 3H, CH2C
3). MALDI-TOF MS: calcd for: 1213.5125, found: 1213.5162.
Preparation of organic nanoparticles
T-BDP NPs were prepared using the nanoprecipitation method. In brief, T-BDP was dissolved in 5 mL THF with a concentration of 1 mg mL−1. The dark solution was then injected into 10 mL of DI water through a micro syringe. After ultrasonication for 20 min, THF was removed by N2 bubbling.
Aggregation-induced fluorescence properties
The aggregation-induced fluorescence properties were studied by measuring the change of the emission spectrum of molecules in the THF solution with the increase of water content. T-BDP was formulated into a solution with a water content of 0–90% at a concentration of 10 μM, and the change of the spectrum was recorded. For comparison, the solution of NI-BDP was investigated under the same conditions.
ROS assay
2′,7′-Dichlorofluorescein (DCFH) was used as a probe to examine the production of reactive oxygen species (ROS). Firstly, the acetate-protected DCFH-DA was hydrolyzed with 0.1 M NaOH, and PBS buffer (pH = 7.4) was added to prepare a DCFH solution of 5 × 10−7 M. The emission spectrum of DCFH that was mixed with T-BDP or T-BDP NPs was recorded under 635 nm laser irradiation.
Photothermal performances
To evaluate the photothermal effect of T-BDP NPs, nanoparticles with different concentrations (25, 50, 100, 150 and 200 μg mL−1) were irradiated by a 635 nm laser at 1.2 W cm−2 for 600 s. In order to evaluate the dependence of the temperature rise of NPs on the power density of the laser, the temperature of the nanoparticles at 100 μg mL−1 was detected under laser irradiation at different laser power densities (1.0, 1.2, 1.4 and 1.6 W cm−2). The temperature of the solution was monitored every 30 s by an FLIR Thermal Imager. The photothermal conversion efficiency was calculated by measuring the cooling curve of T-BDP NPs under 635 nm laser irradiation at 1.2 W cm−2 for 10 min.
Cell viability assay
The cytotoxicity of T-BDP NPs against A549 cells was evaluated using the MTT assay. First, the A549 cell suspension was seeded in a 96-well plate, and the corresponding medium was added to adjust the cell density to 1 × 105 per well. After 24 h of incubation, DMEM (200 μL) containing T-BDP NPs at different concentrations (0, 5, 10, 25, 50, 75 μg mL−1) was added to the designated wells. After 12 h of incubation at 37 °C in the dark, half of the cells were irradiated with a 635 nm laser (0.75 W cm−2) for 5 min. All these plates were then incubated for an additional 24 h at 37 °C in the dark. The sample wells were washed twice with PBS buffer, and then 100 μL of MTT solution (0.5 mg mL−1) in a freshly prepared medium was added to each sample well. After 4 h of incubation, the medium was completely removed, and dimethyl sulfoxide (DMSO, 200 μL) was then added to each well. The absorbance of the solution at 450 nm in each well was measured using a microplate reader. Each concentration was set up in five parallel groups and the analysis was repeated three times. To further examine the cell viability under the different treatment groups (PBS only, laser only, T-BDP NPs only, and T-BDP NPs + laser), the calcein AM/propidium iodide staining method was carried out using A549 cells.
The intracellular ROS generation
The intracellular ROS generation of T-BDP NPs was studied using DCFH-DA as a probe. First, A549 cells were incubated with T-BDP NPs for 24 h. After washing with PBS, a serum-free medium containing 1 × 10−7 mM DCFH-DA was added and the cells were incubated for another 20 min. The cells were then washed three times with PBS to remove extracellular DCFH-DA, followed by irradiation for 8 min with a 635 nm laser at 1.2 W cm−2. The fluorescence images were recorded using a fluorescence microscope.
Fluorescence imaging and subcellular localization
A confocal laser scanning microscope was used to study the uptake and localization of T-BDP NPs in A549 cells. First, A549 cells were incubated in an incubator at 37 °C for 24 h on a confocal dish. The medium was then renewed. After incubating with the nanoparticles at a concentration of 15 μg mL−1 for 8 h, the cells were washed 3 times with PBS buffer. The fluorescence images were then obtained using a laser scanning confocal microscope.
Co-staining of NPs, Hoechst 33342 and LysoTracker green: A549 cells were incubated with T-BDP NPs at 37 °C for 12 h, and then incubated with Hoechst 33342 (50 nM) or LysoTracker green (50 nM) for 10 min. After washing 3 times with PBS solution, the cells were observed using a laser scanning confocal microscope.
Conflicts of interest
There are no conflicts to declare.
Acknowledgements
We are grateful for the support from the National Natural Science Foundation of China (No. 21601142 and 81902356) and Programs for Medical Science and Technology Research Project of Henan Province Health Commission (No. 2018020025 and SB201901029). J. Zhang thanks the Scientific Research Foundation of Taizhou University (No. 2017JQ002), the Taizhou Science and Technology Plan Project (No. 1803gy02), and the Natural Science Foundation of Zhejiang Province (No. LY21B010002).
References
- M. Aioub and M. A. El-Sayed, A Real-Time Surface Enhanced Raman Spectroscopy Study of Plasmonic Photothermal Cell Death Using Targeted Gold Nanoparticles, J. Am. Chem. Soc., 2016, 138, 1258–1264 CrossRef CAS.
- Y. Liu, M. Yang, J. Zhang, X. Zhi, C. Li, C. Zhang, F. Pan, K. Wang, Y. Yang and J. Martinez de la Fuentea, Human Induced Pluripotent Stem Cells for Tumor Targeted Delivery of Gold Nanorods and Enhanced Photothermal Therapy, ACS Nano, 2016, 10, 2375–2385 CrossRef CAS.
- X. Song, Q. Chen and Z. Liu, Recent Advances in the Development of Organic Photothermal Nano-Agents, Nano Res., 2015, 8, 340–354 CrossRef CAS.
- L. Zhao, Y. Liu, R. Xing and X. Yan, Supramolecular photothermal effects: a promising mechanism for efficient thermal conversion, Angew. Chem., Int. Ed., 2020, 59, 3793–3801 CrossRef CAS.
- L. Chen, Y. Zhao, X. Sun, J. Jiang, F. Wu and K. Wang, Synthesis, singlet oxygen generation and DNA photocleavage of β,β′-conjugated polycationic porphyrins, J. Porphyrins Phthalocyanines, 2019, 23, 655–663 CrossRef CAS.
- S. Zhu, S. Yao, F. Wu, L. Jiang, K. L. Wong, J. Zhou and K. Wang, Platinated Porphyrin as a New Organelle and Nucleus Dual-targeted Photosensitizer for Photodynamic Therapy, Org. Biomol. Chem., 2017, 15, 5764–5771 RSC.
- Y. Zheng, S. Zhu, L. Jiang, F. Wu, C. Huang, Z. Li, K.-L. Wong, Z. Xu and K. Wang, Synthesis, Singlet Oxygen Generation, Photocytotoxicity and Subcellular Localization of Azobisporphyrins as Potentially Photodynamic Therapeutic Agents in Vitro Cell Study, J. Porphyrins Phthalocyanines, 2017, 21, 122–127 CrossRef CAS.
- L. Chen, D. Liu, M. Wu, H.-F. Chau, K. Wang, Y.-H. Fung, K.-L. Wong, Z. Wang and F. Wu, Photodynamic and Photothermal Synergistic Behaviors of Triphenylamine-porphyrin nanoparticles for DNA Interaction, Cellular Cytotoxicity and Localization, Nanotechnology, 2020, 31, 315101 CrossRef.
- X. Zhu, W. Feng, J. Chang, Y. W. Tan, J. Li, M. Chen, Y. Sun and F. Li, Temperature-Feedback Upconversion Nanocomposite for Accurate Photothermal Therapy at Facile Temperature, Nat. Commun., 2016, 7, 10437 CrossRef CAS.
- L. Yue, H. Li, Q. Sun, J. Zhang, X. Luo, F. Wu and X. Zhu, Red-Emissive Ruthenium-Containing Carbon Dots for Bioimaging and Photodynamic Cancer Therapy, ACS Appl. Nano Mater., 2020, 3, 869–876 CrossRef CAS.
- K. Hayashi, N. Miki, H. Ozaki, S. Ozaki, M. Abe, T. Matsumoto, T. Kori and K. Ishimura, Photostable Iodinated Silica/Porphyrin Hybrid Nanoparticles with Heavy-Atom Effect for Wide-Field Photodynamic/Photothermal Therapy Using Single Light Source, Adv. Funct. Mater., 2014, 24, 503–513 CrossRef CAS.
- D. Jaque, L. Martínez Maestro, B. Del Rosal, P. Haro-Gonzalez, A. Benayas, J. L. Plaza, E. Martín Rodríguez and J. García Solé, Nanoparticles for photothermal therapies, Nanoscale, 2014, 6, 9494–9529 RSC.
- N. S. Abadeer and C. J. Murphy, Recent Progress in Cancer Thermal Therapy Using Gold Nanoparticles, J. Phys. Chem. C, 2016, 120, 4691–4716 CrossRef CAS.
- S. Yao, L. Chen, F. Jia, X. Sun, H. Su, H. Liu, L. Yang, Z. Wang, F. Wu and K. Wang, Platinated porphyrin tailed with folic acid conjugate for cell-targeted photodynamic activity, J. Lumin., 2019, 214, 116552 CrossRef CAS.
- M. Yang, J. Deng, D. Guo, J. Zhang, L. Yang and F. Wu, A Folate-Conjugated Platinum Porphyrin Complex as a New Cancer-Targeting Photosenitizer for Photodynamic Therapy, Org. Biomol. Chem., 2019, 17, 5367–5374 RSC.
- M. Yang, J. Deng, D. Guo, Q. Sun, Z. Wang, K. Wang and F. Wu, Mitochondria-targeting Pt/Mn porphyrins as efficient photosensitizers for magnetic resonance imaging and photodynamic therapy, Dyes Pigm., 2019, 166, 189–195 CrossRef CAS.
- F. Wu, M. Yang, J. Zhang, S. Zhu, M. Shi and K. Wang, Metalloporphyrin-indomethacin conjugates as new photosensitizers for photodynamic therapy, J. Biol. Inorg. Chem., 2019, 24, 53–60 CrossRef CAS.
- S. Yao, Y. Zheng, L. Jiang, C. Xie, F. Wu, C. Huang, X. Zhang, K.-L. Wong, Z. Li and K. Wang, Methylene violet 3RAX-conjugated porphyrin for photodynamic therapy: synthesis, DNA photocleavage, and cell study, RSC Adv., 2018, 8, 4472–4477 RSC.
- F. Wu, L. Yue, H. Su, K. Wang, L. Yang and X. Zhu, Carbon Dots@Platinum Porphyrin Composite as Theranostic Nanoagent for Efficient Photodynamic Cancer Therapy, Nanoscale Res. Lett., 2018, 13, 357 CrossRef.
- J. Qiu, Q. Xiao, X. Zheng, L. Zhang, H. Xing, D. Ni, Y. Liu, S. Zhang, Q. Ren and Y. Hua, Single W18O49 Nanowires: a Multifunctional Nanoplatform for Computed Tomography Imaging and Photothermal/Photodynamic/Radiation Synergistic Cancer Therapy, Nano Res., 2015, 8, 3580–3590 CrossRef CAS.
- N. Kotagiri, G. P. Sudlow, W. J. Akers and S. Achilefu, Breaking the Depth Dependency of Phototherapy with Cerenkov Radiation and Low-Radiance-Responsive Nanophotosensitizers, Nat. Nanotechnol., 2015, 10, 370–379 CrossRef CAS.
- M. Yang, S. Cao, X. Sun, H. Su, H. Li, G. Liu, X. Luo and F. Wu, Self-Assembled Naphthalimide Conjugated Porphyrins Nanomaterials with D–A Structure for PDT/PTT Synergistic Therapy, Bioconjugate Chem., 2020, 31, 663–672 CrossRef CAS.
- M. Lismont, L. Dreesen and S. Wuttke, Metal–Organic Framework Nanoparticles in Photodynamic Therapy: Current Status and Perspectives, Adv. Funct. Mater., 2017, 27, 1606314 CrossRef.
- J. Zhang, X. Zhu, A. Zhong, W. Jia, F. Wu, D. Li, H. Tong, C. Wu, W. Tang, P. Zhang, L. Wang and D. Han, New platinum(II) one-armed Schiff base complexes for blue and orange PHOLEDs applications, Org. Electron., 2017, 42, 153–162 CrossRef CAS.
- L. Minai, D. Yeheskely-Hayon and D. Yelin, High levels of reactive oxygen species in gold nanoparticle-targeted cancer cells following femtosecond pulse irradiation, Sci. Rep., 2013, 3, 2146–2152 CrossRef.
- L. Yang, H. Li, D. Liu, H. Su, K. Wang, G. Liu, X. Luo and F. Wu, Organic Small Molecular Nanoparticles Based on Self-Assembly of Amphiphilic Fluoroporphyrins for Photodynamic and Photothermal Synergistic Cancer Therapy, Colloids Surf., B, 2019, 182, 110345 CrossRef CAS.
- F. Wu, L. Chen, L. Yue, K. Wang, K. Cheng, J. Chen, X. Luo and T. Zhang, Small-Molecule Porphyrin-Based Organic Nanoparticles with Remarkable Photothermal Conversion Efficiency for in Vivo Photoacoustic Imaging and Photothermal Therapy, ACS Appl. Mater. Interfaces, 2019, 11, 21408–21416 CrossRef CAS.
- C. Laura, S. S. Wen, E. Gülçin and S. Amitav, A Surfactant-Free Direct Access to Porphyrin-Crosslinked Nanogels for Photodynamic and Photothermal Therapy, Bioconjugate Chem., 2018, 29, 4149–4159 CrossRef.
- D. Zhang, M. Wu, Y. Zeng, L. Wu, Q. Wang, X. Han, X. Liu and J. Liu, Chlorin e6 Conjugated Poly(dopamine) Nanospheres as PDT/PTT Dual-Modal Therapeutic Agents for Enhanced Cancer Therapy, ACS Appl. Mater. Interfaces, 2015, 7, 8176–8187 CrossRef CAS.
- J. F. Lovell, C. S. Jin, E. Huynh, H. Jin, C. Kim, J. L. Rubinstein, W. C. W. Chan, W. Cao, L. V. Wang and Z. Gang, Porphysome nanovesicles generated by porphyrin bilayers for use as multimodal biophotonic contrast agents, Nat. Mater., 2011, 10, 324–332 CrossRef CAS.
- C. S. Jin, J. F. Lovell, J. Chen and G. Zheng, Ablation of Hypoxic Tumors with Dose-Equivalent Photothermal, but Not Photodynamic, Therapy Using a Nanostructured Porphyrin Assembly, ACS Nano, 2013, 7, 2541–2550 CrossRef CAS.
- N. Alifu, A. Zebibula, J. Qi, H. Zhang, C. Sun, X. Yu, D. Xue, J. W. Y. Lam, G. Li, J. Qian and B. Z. Tang, Single-Molecular Near-Infrared-II Theranostic Systems: Ultrastable Aggregation-Induced Emission Nanoparticles for Long-Term Tracing and Efficient Photothermal Therapy, ACS Nano, 2018, 12, 11282–11293 CrossRef CAS.
- C. Chen, X. Ni, S. Jia, Y. Liang, X. Wu, D. Kong and D. Ding, Massively Evoking Immunogenic Cell Death by Focused Mitochondrial Oxidative Stress using an AIE Luminogen with a Twisted Molecular Structure, Adv. Mater., 2019, 31, e1904914 CrossRef.
- C. Chen, H. Ou, R. Liu and D. Ding, Regulating the Photophysical Property of Organic/Polymer Optical Agents for Promoted Cancer Phototheranostics., Adv. Mater., 2019, 31, 1806331 Search PubMed.
- X. Ni, X. Zhang, X. Duan, H. L. Zheng, X. S. Xue and D. Ding, Near-Infrared Afterglow Luminescent Aggregation-Induced Emission Dots with Ultrahigh Tumor-to-Liver Signal Ratio for Promoted Image-Guided Cancer Surgery, Nano Lett., 2019, 19, 318–330 CrossRef CAS.
- T. Bura, N. Leclerc, S. Fall, P. Leveque, T. Heiser, P. Retailleau, S. Rihn, A. Mirloup and R. Ziessel, High-performance solutionprocessed solar cells and ambipolar behavior in organic field-effect transistors with thienyl-BODIPY scaffoldings, J. Am. Chem. Soc., 2012, 134, 17404–17407 CrossRef CAS.
- Y. F. Xiao, F. F. An, J. X. Chen, J. Yu, W. W. Tao, Z. Yu, R. Ting, C. S. Lee and X. H. Zhang, The Nanoassembly of an Intrinsically Cytotoxic Near-Infrared Dye for Multifunctionally Synergistic Theranostics, Small, 2019, 15, 1903121 CrossRef.
- J. Zhang, C. Li, X. Zhang, S. Huo, S. Jin, F. F. An, X. Wang, X. Xue, C. I. Okeke, G. Duan, F. Guo, X. Zhang, J. Hao, P. C. Wang, J. Zhang and X. J. Liang,
In vivo tumor-targeted dual-modal fluorescence/CT imaging using a nanoprobe co-loaded with an aggregation-induced emission dye and gold nanoparticles, Biomaterials, 2015, 42, 103–111 CrossRef CAS.
- L. Gai, H. Lu, B. Zou, G. Lai, Z. Shen and Z. Li, Synthesis and spectroscopic properties of bodipy dimers with effective solid-state emission, RSC Adv., 2012, 2, 8840–8846 RSC.
- D. Wang, M. M. S. Lee, G. Shan, R. T. K. Kwok, J. W. Y. Lam, H. Su, Y. Cai and B. Z. Tang, Highly Efficient Photosensitizers with Far-Red/Near-Infrared Aggregation-Induced Emission for In Vitro and In Vivo Cancer Theranostics, Adv. Mater., 2018, 30, e1802105 CrossRef.
- D. Wang, H. Su, R. T. K. Kwok, X. Hu, H. Zou, Q. Luo, M. M. S. Lee, W. Xu, J. W. Y. Lam and B. Z. Tang, Rational design of a water-soluble NIR AIEgen, and its application in ultrafast wash-free cellular imaging and photodynamic cancer cell ablation, Chem. Sci., 2018, 9, 3685–3693 RSC.
- A. Kamkaew, S. H. Lim, H. B. Lee, L. V. Kiew, L. Y. Chung and K. Burgess, BODIPY dyes in photodynamic therapy, Chem. Soc. Rev., 2013, 42, 77–88 RSC.
- P. Yuan, Z. Ruan, W. Jiang, L. Liu, J. X. Dou, T. W. Li and L. F. Yan, Oxygen self-sufficient fluorinated polypeptide nanoparticles for NIR imaging-guided enhanced photodynamic therapy, J. Mater. Chem. B, 2018, 6, 2323–2331 RSC.
- G. Ulrich, R. Ziessel and A. Harriman, The chemistry of fluorescent bodipy dyes: versatility unsurpassed, Angew. Chem., Int. Ed., 2008, 47, 1184–1201 CrossRef CAS.
- H. Lu, J. Mack, Y. Yang and Z. Shen, Structural modification strategies for the rational design of red/NIR region BODIPYs, Chem. Soc. Rev., 2014, 43, 4778–4823 RSC.
- K. Pu, J. Mei, J. V. Jokerst, G. Hong, A. L. Antaris, N. Chattopadhyay, A. J. Shuhendler, T. Kurosawa, Y. Zhou and S. S. Gambhir, Diketopyrrolopyrrole-Based Semiconducting Polymer Nanoparticles for In Vivo Photoacoustic Imaging, Adv. Mater., 2015, 27, 5184–5190 CrossRef CAS.
- D. Gudeika, J. V. Grazulevicius, G. Sini, A. Bucinskas, V. Jankauskas, A. Miasojedovas and S. Jursenas, New derivatives of triphenylamine and naphthalimide as ambipolar organic semiconductors: experimental and theoretical approach, Dyes Pigm., 2014, 106, 58–70 CrossRef CAS.
- H. Huang, D. Wang, Y. Zhang, Y. Zhou, J. Geng, U. Chitgupi, T. R. Cook, J. Xia and J. F. Lovell, Axial PEGylation of Tin Octabutoxy Naphthalocyanine Extends Blood Circulation for Photoacoustic Vascular Imaging, Bioconjugate Chem., 2016, 27, 1574–1580 CrossRef CAS.
- R. Hu, A. Qin and B. Z. Tang, AIE polymers: synthesis and applications, Prog. Polym. Sci., 2019, 100, 101176 CrossRef.
- Y. Tetsuji, T. Yasuhiko and I. Yoshito, Distribution and Tissue Uptake of Poly(ethylene glycol) with Different Molecular Weights after Intravenous Administration to Mice, J. Pharm. Sci., 1994, 83, 601–606 CrossRef.
- S. J. Li, Y. J. Fu, C. Y. Li, Y. F. Li, L. H. Yi and J. Ou-Yang, A near-infrared fluorescent probe based on bodipy derivative with high quantum yield for selective detection of exogenous and endogenous cysteine in biological samples, Anal. Chim. Acta, 2017, 994, 73–81 CrossRef CAS.
- T. Feng, X. Ai, G. An, P. Yang and Y. Zhao, Charge-Convertible Carbon Dots for Imaging-Guided Drug Delivery with Enhanced in Vivo Cancer Therapeutic Efficiency, ACS Nano, 2016, 10, 4410–4420 CrossRef CAS.
- H. S. Jung, J. H. Lee, K. Kim, S. Koo, P. Verwilst, J. L. Sessler, C. Kang and J. S. Kim, A Mitochondria-Targeted Cryptocyanine-Based Photothermogenic Photosensitizer, J. Am. Chem. Soc., 2017, 139, 9972–9978 CrossRef CAS.
Footnote |
† Electronic supplementary information (ESI) available. See DOI: 10.1039/d0qm00536c |
|
This journal is © the Partner Organisations 2021 |
Click here to see how this site uses Cookies. View our privacy policy here.