DOI:
10.1039/D0QM00493F
(Research Article)
Mater. Chem. Front., 2021,
5, 396-405
Temperature-activated PRP–cryogel for long-term osteogenesis of adipose-derived stem cells to promote bone repair†
Received
16th July 2020
, Accepted 19th October 2020
First published on 19th October 2020
Abstract
Platelet-rich plasma (PRP) is widely used for wound healing with fairly good biocompatibility. However, it suffers from the burst release of growth factors as well as the activation with exogenous activators. In this study, we encapsulated PRP in a cryogel to address both these issues. As the cryogel was prepared in a subzero condition and could load PRP with tough covalent-bond crosslinkers, the activity of the autologous activators in the pristine PRP could be effectively saved and the release rate of the growth factors could be effectively reduced. At 37 °C, the remaining autologous activators could activate PRP without the need of exogenous activators to continuously release growth factors for more than 24 days. The prepared PRP–cryogel could then promote the long-term osteogenesis of adipose-derived stem cells (ADSCs). As a bone tissue engineering strategy, the combination of PRP, cryogel, and ADSCs provides temperature-activated PRP and slow-release growth factors for the long-term osteogenesis of ADSCs to enhance bone repair in rats.
Introduction
Platelet-rich plasma (PRP) has been widely used in surgical treatments, such as bone repair, due to its wound-healing effect.1,2 It is a concentrate of platelets from autologous blood, releasing growth factors including transforming growth factor β (TGF-β) and platelet-derived growth factor (PDGF) to promote the proliferation and differentiation of cells, particularly the stem cells.3 The autologous sources offer a gentle treatment modality without the risk of immunogenic reactions. Therefore, PRP is promising for accelerating the restoration treatment.4 However, the activated PRP usually suffers from a burst release of growth factors and a rapid clearance at the target site within 1 h, because of which multiple injections are required for time-consuming treatment to repair the bone defect.5 Meanwhile, the activation of PRP needs the addition of exogenous activators such as calcium chloride and thrombin,6 which have chances to cause blood clotting in a clinical setting. Strategies that can control the release of growth factors7,8 and do not necessitate the addition of exogenous activators are desirable to address these issues.
The encapsulation of PRP in biocompatible scaffolds such as a hydrogel is a rational way to alleviate the burst release of growth factors.9,10 Actually, PRP itself can form a gel state via thrombin activation.11 Nevertheless, the PRP–thrombin gel is not sufficiently stable to get rid of the fast release of growth factors, let alone the addition of exogenous thrombin.12 When PRP is loaded in firm hydrogels made of chitosan,13 collagen,14 or gelatin,15 the burst release can be alleviated, but the addition of exogenous activators is still inevitable. Fresh blood to prepare PRP actually contains autologous activators. Hypothermic handling during centrifugation can effectively archive the vitality of autologous activators, which can be induced at 37 °C.16,17 Most hydrogels are prepared under room temperature or even at higher temperatures,18 and they are not competent to be the scaffolds of autologous-activators-containing PRP due to the requirement of low-temperature preservation. Fortunately, a cryogel is a kind of hydrogel prepared under subzero conditions,19 which is perfect to store the autologous-activators-containing PRP. Furthermore, the gel networking of most cryogels is developed via the cryogelation of polymeric precursors.20 Polymerization provides a firm crosslinked network with covalent bonding.21 The covalent-linking bond is more resistant to body clearance than coordinate or hydrogen bond,22 which gives an opportunity for the long-term release of growth factors from the encapsulated PRP. Therefore, we assume that the encapsulation of PRP in the cryogel can be a promising strategy to address both the issues of the burst release of growth factors and the addition of exogenous activators.
Hydrogel is also a good scaffold for the culture of therapeutic cells.23,24 Among all the therapeutic cells, mesenchymal stem cells (MSCs) have much better capability of proliferation as progenitor cells to promote bone repair.25 Meanwhile, MSCs can also be autologously obtained in a less invasive procedure to support cell treatment. Bone-marrow-derived MSCs (BMSCs) are the original MSC sources, and they have been extensively proven to be helpful with regard to bone regeneration.26 However, the gain of BMSCs requires bone marrow aspiration and the content of BMSCs in the bone marrow is low. Recently, adipose-derived stem cells (ADSCs) have been considered as a potential candidate for the cell treatment of bone defects because of more abundant availability.27 They are capable of inducing osteogenesis upon osteogenic induction with lower immunogenicity and carcinogenic risk, although osteogenesis preformation is not as good as that in BMSCs.28
In this contribution, we combined PRP, cryogel, and ADSCs for bone tissue engineering, formulating a strategy with temperature-activated PRP and slow-release growth factors for the long-term osteogenesis of ADSCs to promote bone repair in rats (Scheme 1). The autologous-activators-containing PRP can be effectively sealed in cryogels during subzero cryogelation and then autonomously activated at the bone defect site due to temperature recovery to 37 °C. The covalent-bond-linked network of the cryogel is relatively stable upon biodegradation, which guarantees the slow and long-term release of growth factors. The persistently released growth factors subsequently promote the proliferation and osteogenesis of ADSCs seeded in the cryogel, repairing bone defects. This integrated strategy is a well-designed bone tissue engineering technique with the exclusion of exogenous activators, long-term release of growth factors, and utilization of cost-effective ADSCs, which provides a rational way to improve bone tissue engineering.
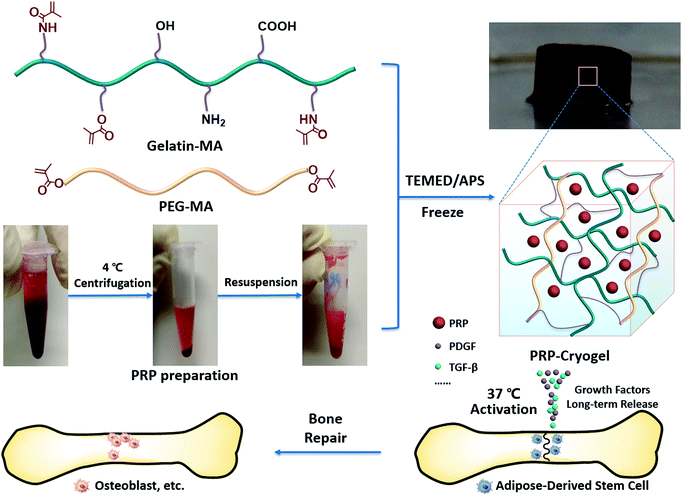 |
| Scheme 1 Schematic illustration of the fabrication of PRP–cryogel and its application in a rat bone defect model. PRP is concentrated from fresh blood using centrifugation at 4 °C to preserve the autologous activators and then dispersed in water containing gelatin–MA (methacryloyl-modified gelatin) and PEG–MA (methacryloyl-modified polyethylene glycol). Cryogelation occurs, affording the PRP–cryogel (PRP-encapsulated cryogel). After recovery to 37 °C, the loaded PRP is activated to sustainably release growth factors, promoting the proliferation and osteogenesis of ADSCs seeded in the cryogel, further repairing the bone defect. | |
Results and discussion
Preparation and characterization of PRP–cryogel
The preparation procedure of the PRP–cryogel has been described in detail in the Experimental section. Briefly, the fresh blood for PRP preparation was collected from the cardiac tissue of adult rats through a syringe (Fig. S1, ESI†). The intracardiac blood sample was then centrifuged at 4 °C to concentrate the PRP-containing autologous activators, which was then resuspended in the supernatant as a stock PRP solution with a fixed platelet concentration for further study. Different preparation methods majorly contribute toward the therapeutic effect of extractive PRP.29 Therefore, the compositions of the prepared PRP were tested to define the prepared PRP with our protocol, including platelets, white blood cells, and red blood cells. As shown in Fig. S2 (ESI†), whole blood contains a high concentration of red blood cells (6746.0 ± 652.53 × 109 L−1) and low concentrations of white blood cells (10.586 ± 0.84 × 109 L−1) and platelets (549.60 ± 70.91 × 109 L−1). After extraction, most red blood cells (277.20 ± 40.52 × 109 L−1) were excluded, but the white blood cells (44.492 ± 1.57 × 109 L−1) and platelets (3100.93 ± 378.91 × 109 L−1) were condensed in the prepared PRP.
The polymeric precursors, namely, gelatin–MA and PEG–MA, were dissolved in water to prepare the cryogel mixture. Different amounts of PRP solution were added into the cryogel mixture to obtain PRP–cryogel at different concentrations of PRP via the polymerization activation of DOPA–MBA (DOPA: dopamine; MBA: N,N′-methylenebisacrylamide) crosslinker with the addition of the APS/TEMED initiator under −20 °C. The PRP–cryogels were referred to as PRP-1–cryogel, PRP-2–cryogel, PRP-3–cryogel, PRP-4–cryogel, and PRP-5–cryogel with gradually increasing concentration of the loaded PRP. Gelatin–MA, derived from collagen, is degradable with the RGD peptide sequence to ensure the cell adhesion and biocompatibility of the cryogel. PEG–MA enables the hydration and nutrient transport properties. DOPA–MBA can further enhance the cell adhesion interactions. Fourier-transform infrared (FTIR) spectroscopic analysis was carried out to confirm successful polymerization. As evident from Fig. S3 (ESI†), the polymeric precursor of PEG–MA has a characteristic peak at 1723 cm−1 for carbonyl in the ester group; gelatin–MA has a peak at 1640 cm−1 for carbonyl in the amide group; the prepared cryogel has two peaks at 1722 and 1654 cm−1 for carbonyl in both ester and amide groups, which indicated the successful combination of PEG and gelatin in the cryogel. The peak of carbonyl in the amide group is apparently shifted from 1640 to 1654 cm−1 because of the breakage of the α,β-unsaturated ketone, further confirming the successful polymerization and preparation of the hydrogel.
As shown in Fig. 1A, the color of the cryogel without PRP is light brown. After PRP encapsulation, the color of PRP-1–cryogel turns brownish red. When the amount of PRP goes higher, the color becomes blood red (PRP-5–cryogel).
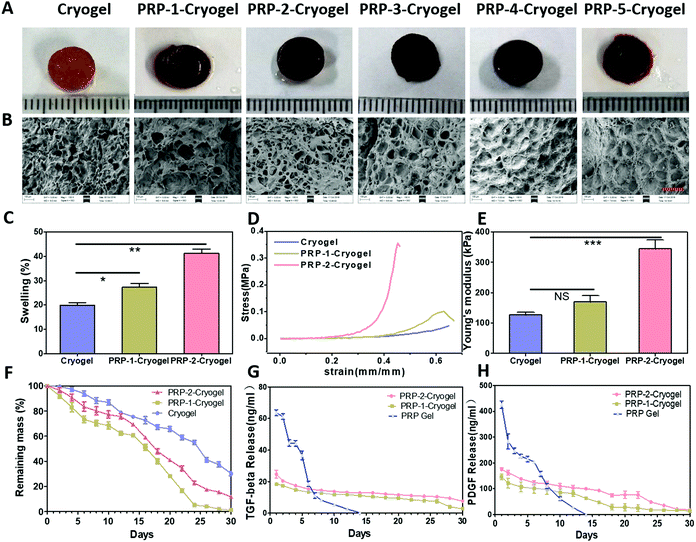 |
| Fig. 1 Characterization of the cryogels and the release of growth factors from the cryogels. Photographs (A) and scanning electron microscopy (SEM) images (B) of freeze-dried samples of cryogel, PRP-1–cryogel, PRP-2–cryogel, PRP-3–cryogel, PRP-4–cryogel, and PRP-5–cryogel. All the samples were coated with gold for SEM observations. The scale bar is 200 μm. The water-swelling degree (C, n = 3; *p < 0.05, **p < 0.01), strain vs. stress curve (D, n = 3), Young's modulus (E, n = 3; NS: no significance; p > 0.05, ***p < 0.001), remaining mass percentages in PBS within 30 days (F, n = 3) of the cryogel, PRP-1–cryogel, and PRP-2–cryogel after the equilibrium of water content. The TGF-β (G, n = 5) and PDGF (H, n = 5) release from PRP-1–cryogel, PRP-2–cryogel (37 °C activation), and PRP gel (thrombin and CaCl2 activation). | |
The microstructures of the prepared cryogels were studied via SEM after freeze-drying (Fig. 1B). The cryogel without PRP has a big pore size with micro-sheet structures, and no obvious crosslinked fibers were observed. With PRP encapsulation, microfibers were blended with the big pore structure (PRP-1–cryogel and PRP-2–cryogel). We hypothesized that the microfibers came from the loaded PRP, which can also form gel structures. However, the pores of the cryogels were mostly filled when the PRP concentration was higher (PRP-3–cryogel, PRP-4–cryogel, and PRP-5–cryogel). As the microstructures are important for cell culture, we further emphasized on studying PRP-1–cryogel and PRP-2–cryogel.
The water-swelling degree of the PRP-encapsulated cryogel is obviously increased compared with that of the free cryogel. Initially, the swelling degree of the cryogel is around 20%, which is enhanced to around 40% for PRP-2–cryogel (Fig. 1C). The enhancement of the water-swelling degree can be mainly ascribed to the microfiber structure obtained from the PRP. The larger swelling degree can provide more circulation of the fluid inside and outside the gels, facilitating the growth factor release from PRP and nutrition supply of cells seeded in the gels. Meanwhile, the stiffness of PRP-2–cryogel is also improved to resist stronger tensile stress (Fig. 1D). The stiffness of PRP-1–cryogel exhibits a marginal change due to the low encapsulation of PRP. The Young's modulus of PRP-2–cryogel is more than two times that of the cryogel (Fig. 1E). Because of the high stiffness of PRP-2–cryogel, it can perform better as a scaffold to support the regeneration of defective bones.
As a carrier, the decomposition rate of the cryogel should be sufficiently slow to ensure the long-term release of growth factors. The decomposition of cryogels was studied in PBS solution. As shown in Fig. 1F, the mass loss rate of the cryogel is low in PBS, remaining above 20% mass after 30-day soaking. After PRP encapsulation, the stability of PRP-1–cryogel and PRP-2–cryogel decreased because of the relatively low stability of PRP. However, the remaining mass of PRP-1–cryogel and PRP-2–cryogel is still sufficiently high after 20-day soaking in PBS, particularly for PRP-2–cryogel. The low decomposition rate of these cryogels can be ascribed to the crosslinked covalent bonds, which should be advantageous for the slow release of growth factors. Subsequently, we studied this advantage by continuously testing the concentrations of the released growth factors, namely, TGF-β and PDGF. The PRP gel—directly formed by mixing PRP with exogenous activators such as thrombin and CaCl2 (Fig. S4, ESI†)—was prepared as the control group to investigate the release rate of growth factors from the PRP–cryogels. The released TGF-β and PDGF were quantified with the corresponding immunoassay kit. The PRP gel released a burst of both TGF-β and PDGF on the first 3 days, and they could only maintain a higher concentration of TGF-β and PDGF within 8 days. However, after temperature activation at 37 °C, both PRP-1–cryogel and PRP-2–cryogel could steadily release TGF-β within 26 days in PBS (Fig. 1G), which also confirmed that PRP–cryogel could be activated without the addition of exogenous activators. PRP-2–cryogel could steadily release PDGF within 22 days, while the time for PRP-1–cryogel was 14 days (Fig. 1H). Obviously, PRP-2–cryogel with more loaded PRP has better performance on long-term growth factor release than PRP-1–cryogel. These results indicated that the combination of PRP and cryogel can address the issues of burst release of growth factors as well as the addition of exogenous activators in vitro.
To qualify whether PRP is preactivated, the activation conditions of platelets in the as-prepared PRP, inactivated PRP-2–cryogel, and 37 °C-activated PRP-2–cryogel were detected by measuring the percentage of CD63-positive platelets via flow cytometry. As shown in Fig. S5 (ESI†), the activation percentages of CD63-positive platelets in the as-prepared PRP, inactivated PRP-2–cryogel, and 37 °C-activated PRP-2–cryogel were 9.5%, 33.0%, and 73.1%, respectively. These results indicated that only a small amount of platelet was activated during the preparation of PRP–cryogel, and a majority of the platelet was activated via temperature activation.
Biocompatibility evaluation for PRP–cryogel
ADSCs were selected as the source of bone regenerative cells due to their abundant availability. ADSCs were isolated from the inguinal adipose tissue of adult rats and further cultured in flasks for 10 days before utilization (Fig. S6, ESI†). Then, they were removed into cryogels to evaluate the biocompatibility via morphological observation, live/dead cell staining, and cell counting kit-8 (CCK-8) assay. The encapsulated PRP was automatically activated during the culture process at 37 °C. After the 3-day culturing of ADSCs in the cryogel, PRP-1–cryogel, and PRP-2–cryogel, the samples were first studied under SEM (Fig. 2A). The cultured ADSCs huddled in the pores of the cryogel, while they spread out well in PRP-1–cryogel and PRP-2–cryogel, indicating the better culture environments of PRP-1–cryogel and PRP-2–cryogel with the assistance of the release growth factors from the encapsulated PRP. Fig. 2B shows the live/dead cell staining of ADSCs cultured in the gels; spots of red signals were observed on day 1 for ADSCs cultured in the cryogel, while a negligible red signal was observed for both PRP-1–cryogel and PRP-2–cryogel. The slight toxicity of the cryogel was possibly caused by the residual DOPA–MBA; PRP encapsulation could eliminate this toxicity and improve the survival environment in the cryogel. When the culture time was 3 and 7 days, the green signals of the living cells were increased, indicating the effective proliferation of ADSCs in the three gels. The green living cells almost covered the entire visual field of imaging after 7-day culture in PRP-1–cryogel and PRP-2–cryogel. The survival rate of ADSCs was also quantified by calculating the ratio of green living cells to red dead cells; PRP-2–cryogel showed the best biocompatibility for ADSCs (Fig. 2C). Live/dead cell staining can only indicate the conditions of ADSCs in the visual field of microscopy. CCK-8 assay was further used to study the comprehensive biocompatibility of cryogels for ADSCs (Fig. 1D). The cell viability of the cultured ADSCs in PRP-2–cryogel was considerably higher than those in the cryogel and PRP-1–cryogel. The live/dead cell staining and CCK-8 assay were also studied for ADSCs cultured in PRP-3–cryogel, PRP-4–cryogel, and PRP-5–cryogel with more encapsulated PRP (Fig. S7, ESI†); all the survival rates and viabilities of the ADSCs were better than those for the cryogel, but not as high as those in PRP-2–cryogel, which can be attributed to the severe blocking of the cryogel pores. All these results confirm that the PRP-encapsulated cryogels show good biocompatibility, particularly PRP-2–cryogel with a moderate amount of encapsulated PRP.
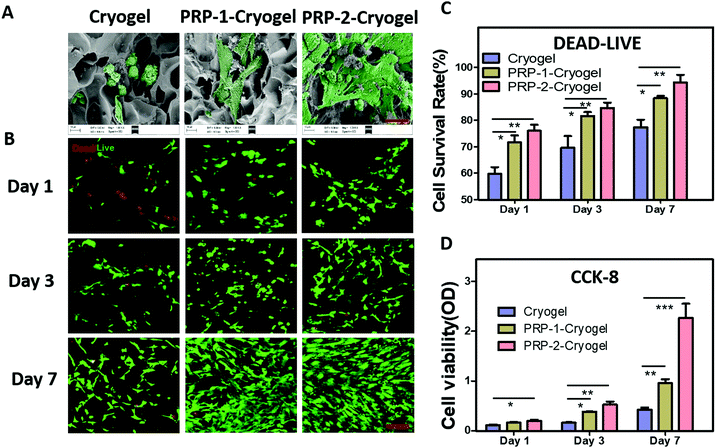 |
| Fig. 2 Biocompatibility of the PRP–cryogels. (A) SEM images of cells seeded on the scaffolds. The morphologies of ADSCs on different cryogels were observed under SEM after culturing for 3 days; the green spots represent the ADSCs. The cells cultured on the PRP-2–cryogel scaffolds were uniformly distributed within all the porous scaffolds, resembling a spider network on day 3. The scale bar is 20 μm. (B) PRP–cryogel exhibited excellent cell compatibility as verified by the live/dead cell staining assay. Live/dead cell staining for ADSCs on day 1, day 3, and day 7. The live cells are shown in green and the dead cells are shown in red. The scale bar is 100 μm. (C) Quantitative cell viability of ADSCs based on the live/dead cell staining assay. The rate is the ratio of green living cells to red dead cells (n = 3; *p < 0.05, **p < 0.01). (D) ADSC proliferation on different scaffolds detected by the CCK-8 assay on day 1, day 3, and day 7 (n = 6; *p < 0.05, **p < 0.01, ***p < 0.001). | |
Osteogenesis of ADSCs in PRP-encapsulated cryogel
In this section, as the encapsulated PRP in the prepared PRP–cryogel can be automatically activated at 37 °C, all the PRP–cryogel samples were directly used as scaffolds to promote osteogenesis without the need of any exogenous activators. The details are as follows.
The expression of alkaline phosphatase (ALP) can promote the maturation and calcification of cells. It is a mark of early osteogenesis. Therefore, the ALP activities of ADSCs were measured to determine the degree of early osteogenesis. As evident from Fig. 3A, the ALP activity of ADSCs cultured in the cryogel was gradually increased from day 3 to day 14, confirming that the culture in the cryogel could motivate the osteogenesis capacity of ADSCs. PRP encapsulation could further increase the ALP activity of ADSCs; its value for PRP-2–cryogel was twice that for the cryogel. This increase in the ALP activity can be mainly attributed to the differentiation of ADSCs with gene expression transformation. We subsequently detected the ALP-related gene expression level in cultured ADSCs via a qPCR analysis (Fig. 3B). The cryogel could relatively increase the ALP-related gene expression level on day 14. PRP-1–cryogel performed better than the cryogel. In PRP-2-cryogel with an appropriate amount of PRP, ADSCs expressed a dramatically increased level of ALP-related gene.
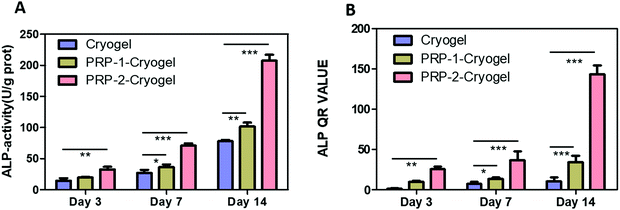 |
| Fig. 3 ALP activity and real-time qPCR results of the ALP-related gene expression implied that the PRP–cryogel could induce osteogenesis in vitro. (A) ALP activities of ADSCs cultured on scaffolds on day 3, day 7, and day 14 (n = 3; *p < 0.05, **p < 0.01, ***p < 0.001). (B) ALP-related gene expression levels of ADSCs after culturing in different scaffolds. The entire study employed an osteogenic inducer (50 μg mL−1L-ascorbic acid-1-phosphate, 10 mM β-glycerophosphate, and 100 nM dexamethasone). *p < 0.05, **p < 0.01, ***p < 0.001. | |
Osteopontin (OPN) plays a very important role in tissue repair. The expression level of the OPN-related gene was also studied through qPCR analysis (Fig. S8, ESI†) after culturing ADSCs in the three cryogels with osteogenesis-inducing supplements. Similar to the ALP-related gene expression on day 14, the OPN-related gene expression of ADSCs in the cryogel was low, but the situation was better in PRP-1–cryogel due to PRP encapsulation and growth factor release; further, the expression level was higher in PRP-2–cryogel with a proper amount of PRP. The expression of the OPN protein was then visualized via immunofluorescence analysis. Based on the CLSM data shown in Fig. S9 (ESI†), no green signals were observed neither on day 7 nor on day 14 to indicate the OPN expression of ADSCs when cultured in the cryogel without an osteogenic inducer. The OPN expression levels were gradually increased when cultured in PRP-1–cryogel and PRP-2–cryogel; the signals were slight but visible. With the assistance of the osteogenic inducer, the expressions of OPN were apparently increased for all the groups. Nonetheless, the ADSCs cultured in PRP-2–cryogel showed the highest OPN expression level.
Overall, bone repair relies on the osteogenesis efficiency. The calcification of ADSCs was then studied via Alizarin Red S staining to indicate osteogenesis degree (Fig. 4). ADSCs showed negligible calcification after culturing in the three cryogels on day 14 (Fig. 4A). After further culturing for another 7 days, the calcifications were improved for ADSCs cultured in all the three cryogels, and the calcium nodule area ratio was apparently increased from cryogel to PRP-1–cryogel and PRP-2–cryogel (Fig. 4C), indicating the osteogenesis promotion capacity of the scaffold comprising PRP and cryogel. However, since the osteogenesis of ADSCs is rather weak when they were not in an environment of bone defects, the calcium nodule area of the cultured ADSCs was too low to get firm results. Therefore, the osteogenic promotion capacity of PRP–cryogels was exhibited more clearly with the assistance of the osteogenesis inducer (Fig. 4B and D). On day 14, the ADSCs cultured in the cryogel had few calcium nodule areas, while the ones cultured in PRP-1–cryogel and PRP-2–cryogel formed dense calcium nodule areas. On day 21, all the ADSCs cultured in the three cryogels exhibited obvious calcium nodule areas, while the ADSCs in PRP-2–cryogel performed the best. Actually, in the in vitro study, the calcium nodule area ratio was apparently increased from cryogel to PRP-1–cryogel and PRP-2–cryogel even though the calcification effect is not good for all the groups without osteogenic induction. This could indicate the calcification promotion effect of PRP in cryogels. Meanwhile, the calcification trend is the same as the groups with osteogenic induction. The difference is that the calcium nodule area in the groups with osteogenic induction is much higher than that in the ones without osteogenic induction. This indicated that osteogenic induction played a very important role in the calcification process. ADSCs are the most promising to generate adipose cells, which are harmful for the process of bone repair. Therefore, the adipogenic capacity of ADSCs in the three cryogels were also studied to evaluate the risk factors. Fortunately, Oil Red O staining showed that no adipose cells were generated when the ADSCs were cultured in the three cryogels (Fig. S10, ESI†).
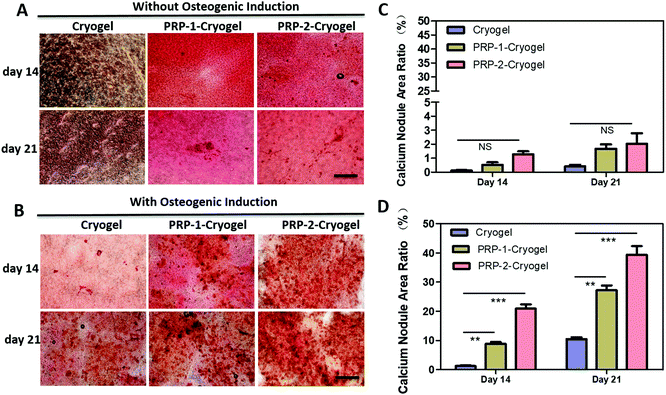 |
| Fig. 4 Osteoblastic ability of different cryogels indicated by Alizarin Red S staining. Alizarin Red S staining (A and B) and calcium nodule area ratio (C and D) of ADSC cells cultured on different cryogels for 14 and 21 days without (A and C) and with (B and D) osteogenic inducer (magnification: 4×; scale bar, 1 mm), n = 3; NS: no significance, **p < 0.01, ***p < 0.001. | |
Repair of bone defects in vivo
Rats with two skull defects were used to further characterize the bone repair capacity of the PRP–cryogel (Fig. S11, ESI†). As shown in Fig. 5A, 3D reconstructions from the micro-CT images of the bone defects were shown to confirm the repair of skull defects. The skull defects on the right-hand side with colored rings were treated with cryogel, cryogel/ADSC, PRP-2-cryogel, and PRP-2-cryogel/ADSC, while the ones on the left-hand side were set as the control without any further treatment. New bone generation was observed in all the groups after 8 weeks without the addition of any exogenous PRP activators. The control group showed minimal bone formation within the defect, while the defects treated with cryogel, cryogel/ADSC, PRP-2–cryogel, or PRP-2–cryogel/ADSC consistently exhibited the regeneration of newer tissues. The statistical analysis of the maxillary bone repair was then carried out to clearly study the treatment efficiency. There is an increasing tendency of the quantified BV/TV (Fig. 5B) and BMD (Fig. 5C) and a decreasing tendency of trabecular spacing (Fig. 5D) within the defect area treated with the cryogel, cryogel/ADSC, PRP-2–cryogel, and PRP-2–cryogel/ADSC, respectively. All the aspects including the cryogel, ADSC, and PRP could facilitate bone repair. With the assistance of the long-term release of PRP, PRP-2–cryogel showed better bone repair than cryogel/ADSC. When they were integrated together as PRP-2–cryogel/ADSC, the treatment effect was the best.
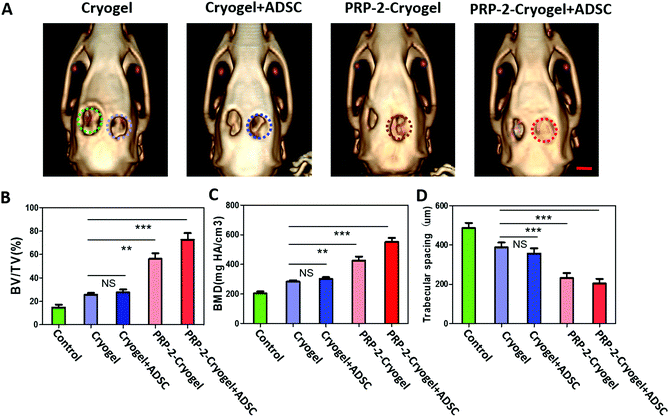 |
| Fig. 5
In vivo bone repair and computerized tomography (CT) analysis. (A) 3D reconstructions from the micro-CT images of bone defects were shown after 8-week treatments including cryogel, Cryogel/ADSC, PRP-2–cryogel, and PRP-2–cryogel/ADSC. All the holes on the left-hand side were the control groups, and the holes on the right-hand side were the experimental groups. The colored rings show the treated bone defects. CT analysis of the rats indicated that the PRP–cryogel could repair calvarium bone defects in vivo. The scale bar is 5 mm. (B–D) Statistical analyses of the maxillary bone defect areas (T-test). The ordinates of the statistical charts were the quantified numbers of pixels for the defect area's bone volume/tissue volume (BV/TV) (B), bone mineral density (BMD) (C), and trabecular spacing (D). All the data are shown as the mean ± standard deviation (n = 5 per group; NS: no significance, **p < 0.01, ***p < 0.001). | |
Tissue generation was further evaluated by the histological analysis of an 8-week tissue slice. Collagen fibers (blue area in Masson's staining) could be observed in all the groups. Osteoid (red area in Masson's staining, which represents bone maturation) could only be seen in the treated groups rather than in the control group. According to hematoxylin and eosin (H&E) and Masson's staining protocols (Fig. 6), a large visible area of osteoid and dense connective tissue were observed in the bone defect treated with PRP-2–cryogel and PRP-2–cryogel/ADSC. This is strong evidence that the slowly released PRP can apparently promote bone repair.
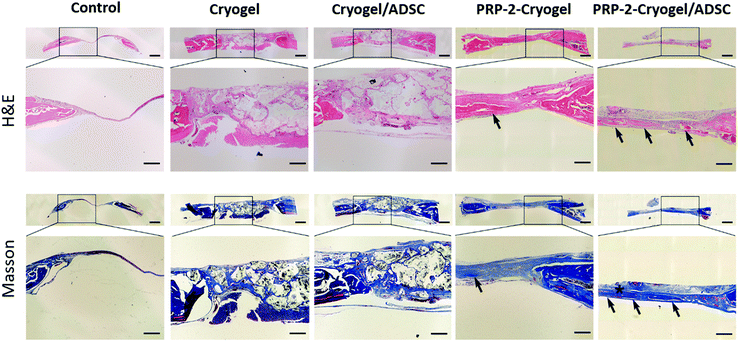 |
| Fig. 6 H&E and Masson's staining of a calvarium recovering at 8 weeks between the different groups. The blue area indicated by the black arrow is the new bone tissue, and the red area indicated by the black asterisk is the mature bone (the upper panel is at a magnification of 4× and scale bar is 1 cm; the lower panel is at a magnification of 10× and the scale bar is 400 μm). | |
Experimental
Preparation of PRP–cryogel
The concentrations of gelatin–MA, PEG–MA, and DOPA–MBA crosslinker solutions were 33.3, 33.3, and 0.66 mg mL−1, respectively. The three solutions were uniformly mixed at 50 °C to prepare the cryogel mixture. When the temperature of the mixture drops to room temperature, the PRP stock solutions (0, 20, 40, 60, 80, or 100 μL) containing platelets (3100.93 ± 378.91 × 109 L−1), white blood cells (44.492 ± 1.57 × 109 L−1), and red blood cells (277.20 ± 40.52 × 109 L−1) were dispersed into different volumes (100, 80, 60, 40, 20, or 0 μL) of deionized water and they were then added into the cryogel mixture to get different prepolymer solutions at room temperature. Finally, 3 μL of 10% APS and 1 μL TEMED were quickly added into the prepolymer solution and the final mixture was put into a −20 °C freezer overnight to form the different cryogels (cryogel, PRP-1–cryogel, PRP-2–cryogel, PRP-3–cryogel, PRP-4–cryogel, and PRP-5–cryogel). The cryogels were gently shaken at 90 rpm in PBS at 4 °C to eliminate the APS/TEMED initiator. Dopamine was sterilized by filtration; gelatin–MA was irradiated under UV light in a biosafety hood for 30 min for sterilizing. The whole synthesis needs to be completed quickly under sterile conditions.
Bone defect experiment in vivo
Soft tissue dissection after a scalp incision was used to expose the calvarium. The periosteum was scraped off to expose the underlying bone. A trephine drill (Salvin Dental Specialties) was used to create two circular critical-size defects (diameter: 8.0 mm) with an intermittent irrigation of the site with PBS. The calvarium was excised and discarded while maintaining the dura. The rats were randomized into four groups (n = 5 each). First, the ADSCs were cultured on the hydrogels to prepare the hydrogels with ADSCs; then, cryogel, cryogel/ADSC, PRP-2–cryogel, or PRP-2–cryogel/ADSC (washed with PBS three times and activated at 37 °C) was placed on the right-hand-side defect site and immobilized with sutures to the surrounding soft tissue. The wound and incision were closed, and the animals were well cared for until recovery. The wounds with no treatment were used as the control group. The rats were individually raised for 8 weeks under standardized conditions (50% relative humidity; 25 °C). All the animal experiments were carried out under the approval of the Experimental Animal Center of the Southern Medical University under production license SCXK (Guangdong) 2016-0041 and operation license SYXK (Guangdong) 2016-0167.
CT analysis
After being raised for 8 weeks, the rats were anesthetized and supinely fixed on the bed of an animal PET/CT instrument from Inveon (Siemens, Germany). The graphics were obtained in the field of view of 7 × 14 cm2 at a horizontal height of the bone defect wound surfaces. Images were reconstructed and analyzed with the MicroView software (GE Healthcare). Defect margins were established to delineate a standard region of interest per animal. A threshold value (constant for all the groups) was selected, and the BMD and BV values were measured by using the Bone Analysis tool. The graphics were performed in triplicate. All the results are expressed as the mean ± standard deviation of the mean.
Conclusions
The results of this study demonstrated that the cryogel could encapsulate PRP with activity-remaining autologous activators, realizing temperature activation without the addition of exogenous activators, long-term release of growth factors, acceleration of osteogenesis of ADSCs, and bone generation in a rat model. After loading in the cryogel, PRP could be activated at 37 °C and the growth factors were continuously released at a constant concentration for more than 20 days. With a proper amount of PRP and ADSC seeding, PRP-2–cryogel dramatically enhanced the repair of skull defects in rats. Our work has demonstrated, for the first time, that the cryogel was the perfect carrier for PRP to address the issues of burst release of growth factors as well as the addition of exogenous activators. We expect that the developed strategy will be beneficial for bone defect treatment.
Conflicts of interest
There are no conflicts to declare.
Acknowledgements
We thank the National Natural Science Foundation of China (U1601221, 31922043), Science and Technology Projects of Guangzhou City (201804020035), National Natural Science Foundation of China (31572343) and Guangdong Province Science and Technology Projects (2017B030314038), Foundation for Basic and Applied Basic Research of Guangdong (2019A1515110241) and Key Research and Development Program of Guangzhou Regenerative Medicine and Health Guangdong Laboratory (2018GZR110104002).
References
- R. E. Marx, E. R. Carlson, R. M. Eichstaedt, S. R. Schimmele, J. E. Strauss and K. R. Georgeff, Platelet-rich plasma: Growth factor enhancement for bone grafts, Oral Surg. Oral Med. Oral Pathol. Oral Radiol. Endod., 1998, 85, 638–646 CrossRef CAS.
- C. A. Carter, D. G. Jolly, C. E. Worden Sr, D. G. Hendren and C. J. M. Kane, Platelet-rich plasma gel promotes differentiation and regeneration during equine wound healing, Exp. Mol. Pathol., 2003, 74, 244–255 CrossRef CAS.
- E. Lucarelli, A. Beccheroni, D. Donati, L. Sangiorgi, A. Cenacchi, A. M. Del Vento, C. Meotti, A. Z. Bertoja, R. Giardino, P. M. Fornasari, M. Mercuri and P. Picci, Platelet-derived growth factors enhance proliferation of human stromal stem cells, Biomaterials, 2003, 24, 3095–3100 CrossRef CAS.
- R. Simman, A. Hoffmann, R. J. Bohinc, W. C. Peterson and A. J. Russ, Role of Platelet-Rich Plasma in Acceleration of Bone Fracture Healing, Ann. Plast. Surg., 2008, 61, 337–344 CrossRef CAS.
- X. Liu, Y. Yang, X. Niu, Q. Lin, B. Zhao, Y. Wang and L. Zhu, An in situ photocrosslinkable platelet rich plasma – Complexed hydrogel glue with growth factor controlled release ability to promote cartilage defect repair, Acta Biomater., 2017, 62, 179–187 CrossRef CAS.
- D. A. Lansdown and L. A. Fortier, Platelet-Rich Plasma: Formulations, Preparations, Constituents, and Their Effects, Oper. Techn. Sport. Med., 2017, 25, 7–12 CrossRef.
- G. Irmak, T. T. Demirtaş and M. Gümüşderelioğlu, Sustained release of growth factors from photoactivated platelet rich plasma (PRP), Eur. J. Pharm. Biopharm., 2020, 148, 67–76 CrossRef CAS.
- H. S. Yang, J. Shin, S. H. Bhang, J.-Y. Shin, J. Park, G.-I. Im, C.-S. Kim and B.-S. Kim, Enhanced skin wound healing by a sustained release of growth factors contained in platelet-rich plasma, Exp. Mol. Med., 2011, 43, 622–629 CrossRef CAS.
- E. Jain, S. Sheth, A. Dunn, S. P. Zustiak and S. A. Sell, Sustained release of multicomponent platelet-rich plasma proteins from hydrolytically degradable PEG hydrogels, J. Biomed. Mater. Res., Part A, 2017, 105, 3304–3314 CrossRef CAS.
- W. Pan, C. Dai, Y. Li, Y. Yin, L. Gong, J. O. A. Machuki, Y. Yang, S. Qiu, K. Guo and F. Gao, PRP-chitosan thermoresponsive hydrogel combined with black phosphorus nanosheets as injectable biomaterial for biotherapy and phototherapy treatment of rheumatoid arthritis, Biomaterials, 2020, 239, 119851 CrossRef CAS.
- R. Landesberg, M. Roy and R. S. Glickman, Quantification of growth factor levels using a simplified method of platelet-rich plasma gel preparation, J. Oral Maxillofac. Surg., 2000, 58, 297–300 CrossRef CAS.
- E. Jain, N. Chinzei, A. Blanco, N. Case, L. J. Sandell, S. Sell, M. F. Rai and S. P. Zustiak, Platelet-Rich Plasma Released From Polyethylene Glycol Hydrogels Exerts Beneficial Effects on Human Chondrocytes, J. Orthop. Res., 2019, 37, 2401–2410 CrossRef CAS.
- T. R. Nimal, G. Baranwal, M. C. Bavya, R. Biswas and R. Jayakumar, Anti-staphylococcal Activity of Injectable Nano Tigecycline/Chitosan-PRP Composite Hydrogel Using Drosophila melanogaster Model for Infectious Wounds, ACS Appl. Mater. Interfaces, 2016, 8, 22074–22083 CrossRef CAS.
- C.-C. Wu, W.-H. Chen, B. Zao, P.-L. Lai, T.-C. Lin, H.-Y. Lo, Y.-H. Shieh, C.-H. Wu and W.-P. Deng, Regenerative potentials of platelet-rich plasma enhanced by collagen in retrieving pro-inflammatory cytokine-inhibited chondrogenesis, Biomaterials, 2011, 32, 5847–5854 CrossRef CAS.
- Y.-H. Kim, H. Furuya and Y. Tabata, Enhancement of bone regeneration by dual release of a macrophage recruitment agent and platelet-rich plasma from gelatin hydrogels, Biomaterials, 2014, 35, 214–224 CrossRef CAS.
- L. Du, M. Yong, L. Xin, P. Shi and Z. Hu, A Novel and Convenient Method for the Preparation and Activation of PRP without Any Additives: Temperature Controlled PRP, BioMed Res. Int., 2018, 2018, 1–12 Search PubMed.
- C. Carola, R. Alice, G. Brunella, M. Erminia, P. Loredana, M. Giulia, K. Elizaveta, M. Maurilio and F. Giuseppe, Platelet-Rich Plasma: The Choice of Activation Method Affects the Release of Bioactive Molecules, BioMed Res. Int., 2016, 2016, 1–7 Search PubMed.
- N. A. Peppas and A. R. Khare, Preparation, structure and diffusional behavior of hydrogels in controlled release, Adv. Drug Delivery Rev., 1993, 11, 1–35 CrossRef CAS.
- Y. Liu, K. Xu, Q. Chang, M. A. Darabi, B. Lin, W. Zhong and M. Xing, Highly Flexible and Resilient Elastin Hybrid Cryogels with Shape Memory, Injectability, Conductivity, and Magnetic Responsive Properties, Adv. Mater., 2016, 28, 7758–7767 CrossRef CAS.
- M. Zinggeler, J.-N. Schönberg, P. L. Fosso, T. Brandstetter and J. Rühe, Functional Cryogel Microstructures Prepared by Light-Induced Cross-Linking of a Photoreactive Copolymer, ACS Appl. Mater. Interfaces, 2017, 9, 12165–12170 CrossRef CAS.
- K. Yue, G. Trujillo-de Santiago, M. M. Alvarez, A. Tamayol, N. Annabi and A. Khademhosseini, Synthesis, properties, and biomedical applications of gelatin methacryloyl (GelMA) hydrogels, Biomaterials, 2015, 73, 254–271 CrossRef CAS.
- S. Khetan, M. Guvendiren, W. R. Legant, D. M. Cohen, C. S. Chen and J. A. Burdick, Degradation-mediated cellular traction directs stem cell fate in covalently crosslinked three-dimensional hydrogels, Nat. Mater., 2013, 12, 458–465 CrossRef CAS.
- N. J. Steinmetz, E. A. Aisenbrey, K. K. Westbrook, H. J. Qi and S. J. Bryant, Mechanical loading regulates human MSC differentiation in a multi-layer hydrogel for osteochondral tissue engineering, Acta Biomater., 2015, 21, 142–153 CrossRef CAS.
- L. Bian, M. Guvendiren, R. L. Mauck and J. A. Burdick, Hydrogels that mimic developmentally relevant matrix and N-cadherin interactions enhance MSC chondrogenesis, Proc. Natl. Acad. Sci. U. S. A., 2013, 110, 10117–10122 CrossRef CAS.
- A. I. Caplan, Mesenchymal stem cells, J. Orthop. Res., 1991, 9, 641–650 CrossRef CAS.
- F. S. Loffredo, M. L. Steinhauser, J. Gannon and R. T. Lee, Bone Marrow-Derived Cell Therapy Stimulates Endogenous Cardiomyocyte Progenitors and Promotes Cardiac Repair, Cell Stem Cell, 2011, 8, 389–398 CrossRef CAS.
- M. O. Kilinc, A. Santidrian, I. Minev, R. Toth, D. Draganov, D. Nguyen, E. Lander, M. Berman, B. Minev and A. A. Szalay, The ratio of ADSCs to HSC-progenitors in adipose tissue derived SVF may provide the key to predict the outcome of stem-cell therapy, Clin. Transl. Med., 2018, 7, 5 Search PubMed.
- X. Xie, Y. Wang, C. Zhao, S. Guo, S. Liu, W. Jia, R. S. Tuan and C. Zhang, Comparative evaluation of MSCs from bone marrow and adipose tissue seeded in PRP-derived scaffold for cartilage regeneration, Biomaterials, 2012, 33, 7008–7018 CrossRef CAS.
- J. Etulain, H. A. Mena, R. P. Meiss, G. Frechtel, S. Gutt, S. Negrotto and M. Schattner, An optimised protocol for platelet-rich plasma preparation to improve its angiogenic and regenerative properties, Sci. Rep., 2018, 8, 1513 CrossRef.
Footnotes |
† Electronic supplementary information (ESI) available. See DOI: 10.1039/d0qm00493f |
‡ Equal contribution. |
|
This journal is © the Partner Organisations 2021 |