Influence of glucose on the human serum albumin–flavone interaction and their antioxidant activity
Received
31st August 2012
, Accepted 9th October 2012
First published on 10th October 2012
Abstract
High levels of glucose in diabetics can react with plasma proteins through a non-enzymatic process. Herein, the influence of glucose on the interaction between flavones and human serum albumin (HSA) was investigated, as well as the effect of glucose on the antioxidant potential of a flavone–HSA system. It looks like the interactions of HSA and flavones with more hydroxyl groups on ring A are more easily affected by glucose. Flavones act as hydrogen bond donors with HSA by means of interacting with glucose. With increased incubation time in air (from 1 to 23 days), the affinities of HSA for multi-hydroxyl flavones on ring A were obviously higher than for non- or mono-hydroxyl flavones on ring A. HSA significantly masked the DPPH radical scavenging potential of 7,8-dihydroxyflavone and baicalein. However, glucose obviously enhances the DPPH scavenging potential of a baicalein–HSA system. Moreover, glucose slightly weakens the DPPH scavenging potential of a 7,8-dihydroxyflavone–HSA system.
Introduction
Flavonoids are the major polyphenols present in a wide variety of plant sources.1–5 Their structure is represented by a benzene ring (A), condensed with a heterocyclic six-membered pyran or pyrone ring (C), in which the 2 or 3 position carries a phenyl ring (B) as a substituent. Over 10
000 flavonoids have been separated and identified from plants, most of which are divided into subclasses, including anthocyanidins, flavanones, flavonols, flavones and isoflavones.6,7 Flavonols are the most widespread flavonoids in plants. The most prominent flavonols in foods are quercetin and kaempferol. Flavones are much less common than flavonols in fruits and vegetables. The prominent flavones in foods are baicalein, chrysin, luteolin and apigenin. Polyphenols, especially flavonoids in food produced by plants, have attracted great interest since the 1990s due to growing evidence of their beneficial effect on human health.8,9
Diabetes has become a great problem in the world. Diabetes is characterized as a high level of glucose in the blood. This glucose can react with plasma proteins through a non-enzymatic process.10 Non-enzymatic glycation of plasma proteins is an important factor that contributes to the development of the complications of diabetes. Human serum albumin (HSA) is the major target interacting with glucose in the blood. Structural changes associated with the exposure of human serum albumin (HSA) to glucose were reported by Coussons et al.11 Barzegar et al. found that partial denaturation and changes in the structural integrity of HSA are caused by glycation at lower (1 mg ml−1) and higher (5 mg ml−1) concentrations of glucose.12 Moreover, L-Trp has a lower affinity for glycated HSA than for the non-glycated form. The secondary structure of advanced glycation end products of HSA (AGE-HSA) derived from glucose at 20 mmol L−1 contains a high α-helical content and elicits the maximum expression of the receptor.13
Recently, polyphenol–protein interactions have attracted wide attention.14–16 Polyphenols in blood are bound to plasma proteins to some degree. The polyphenol–protein interaction is reversible in that the polyphenol–plasma protein complex can dissociate and release the free polyphenols. Polyphenols and their metabolites rapidly exchange between free and bound forms within the circulation. The reversible binding to plasma proteins may have consequences for the delivery of polyphenols and their metabolites to cells and tissues.17
It has been suggested that increasing glycation can influence the ability of plasma proteins to bind to small molecules.18,19 The degree of binding to albumin may have consequences for the rate of the clearance of metabolites and for their delivery to cells and tissues.17 The conventional view is that the cellular uptake is proportional to the unbound concentration of metabolites. The progress made to probe the flavone–protein interaction (in solution phase) is sufficient to provide enough information at a molecular level, but certainly not enough to explain the anti-oxidation property of the flavone–protein complex in vitro or in vivo.20 However, how glucose influences the interaction between flavones and HSA and the antioxidant potential of polyphenols is not clear. Herein, the influence of glucose on the interaction between flavones and HSA was investigated, as well as the effect of glucose on the 1,1-diphenyl-2-picrylhydrazyl (DPPH) free radical scavenging potential of the flavone–HSA system. Flavone, 6-hydroxyflavone, 6-methoxyflavone, 3-hydroxyflavone, 7-hydroxyflavone, 7,8-dihydroxyflavone, chrysin and baicalein (Fig. 1) were tested.
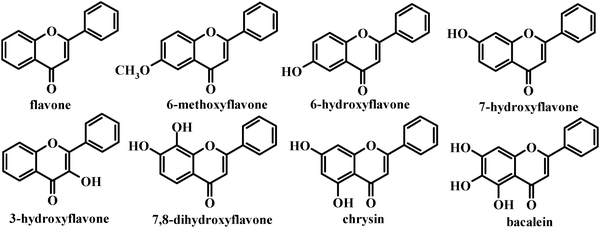 |
| Fig. 1 Chemical structures of the flavones in this study. | |
Materials and methods
Apparatus and reagents
The fluorescence spectra were recorded on a JASCO FP-6500 fluorometer (Tokyo, Japan). Flavone, chrysin, and baicalein (99.5%) were obtained commercially from Wako Pure Chemical Industries (Osaka, Japan). 7,8-Dihydroxyflavone, 7-hydroxyflavone, 6-hydroxyflavone, 6-methoxyflavone, and 3-hydroxyflavone were purchased from TCI Chemical Industries (Tokyo, Japan). The working solutions of flavones (1.0 × 10−3 mol L−1) were prepared by dissolving each flavone in methanol. All other reagents and solvents were of analytical grade and all aqueous solutions were prepared using newly double-distilled water.
Fluorescence spectra
The working solutions of HSA (1.0 × 10−5 mol L−1) were incubated with 100 mmol L−1 glucose at 37 °C in air for 0 to 23 days. A 3.0 mL working solution of HSA or HSA pre-incubated with glucose was transferred to a 1.0 cm quartz cell. The solutions were then titrated with successive addition of 3.0 μL flavone solution (1.0 × 10−3 mol L−1). Titrations were performed manually by using trace syringes. In each titration, the fluorescence spectrum was collected with the working solution of HSA or HSA pre-incubated with glucose. The fluorescence spectra were recorded in the wavelength range 310–450 nm upon excitation at 280 nm when HSA or HSA pre-incubated with glucose was titrated with flavones. The slit widths, scan speed and excitation voltage were kept constant within each data set and each spectrum was the average of three scans. The results of the time course experiments for the equilibration are not given here. Each fluorescence intensity determination was repeated and found to be reproducible within experimental errors. Each flavone was tested with three HSA or HSA pre-incubated with glucose samples and the average binding constants and the number of binding sites were obtained.
DPPH radical scavenging activity
The working solutions of flavones (1.0 × 10−3 mol L−1) were diluted ten times with HSA solution (1.0 × 10−5 mol L−1), MilliQ water, MilliQ water with glucose (100 mmol L−1) or HSA solution (1.0 × 10−5 mol L−1) with glucose (100 mmol L−1) to obtain tested samples. Antioxidant activities of the above samples were measured on the basis of the scavenging DPPH free radical. One milliliter of sample was added to 1 mL DPPH solution (0.2 mmol L−1 in ethanol) to make a free radical source. The decrease in absorbance at 517 nm was measured after 30 min. The DPPH radical scavenging activity was calculated using the following formula:
DPPH radical scavenging activity (%) = (1 − A0/A1) × 100 |
where A0 is the absorbance of the control, and A1 is the absorbance of the samples. Each experiment was repeated three times and found to be reproducible within experimental errors.
Results and discussion
Influence of glucose on the fluorescence quenching of flavones
The intensities of HSA fluorescence decreased remarkably with the addition of flavones. Glucose hardly affects the HSA fluorescence spectrum. Fig. 2 shows the quenching effect of baicalein on the HSA fluorescence spectra in the absence and presence of glucose. Comparing Fig. 2a with Fig. 2b, the quenching effect on HSA fluorescence by baicalein in the presence of glucose was much higher than in the absence of glucose. The quenching percentages of baicalein on the HSA fluorescence spectra in the absence and presence of glucose were 60.34% and 72.66%, respectively.
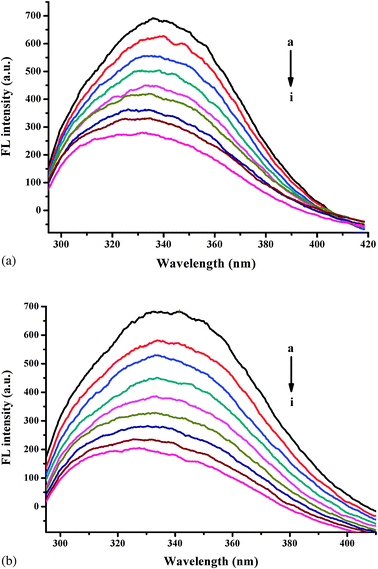 |
| Fig. 2 The quenching effect of baicalein on HSA fluorescence spectra in the absence (a) and presence (b) of glucose. λex = 295 nm; HSA, 10.00 mmol L−1; a–i: 0.00, 1.00, 2.00 ... 8.00 (× 10−6 mol L−1) of baicalein. | |
The binding constants (Ka) and the number of binding sites (n).
The binding constants were calculated according to the double-logarithm equation:21–25 | lg[(F0 − F)/F] = lg Ka + n lg[Q] | (1) |
where F0 and F represent the fluorescence intensities of HSA or HSA pre-incubated with glucose in the absence and in the presence of flavones, Ka is the binding constant, n is the number of binding sites, and [Q] is the concentration of flavones. According to eqn (1) the values of “(F0 − F)/F” can be obtained for each “[Q]”. Then, the linear regression equation between the “lg[(F0 − F)/F]” values and “lg[Q]” values was obtained using Origin 7.5 software. The slope factor is “n” and the intercept refers to “lg Ka”.
Each flavone was tested with three HSA or HSA pre-incubated with glucose samples and the averages of the binding constant and the number of binding sites are summarized in Fig. 3. The values of lg Ka are proportional to the number of binding sites (n) (Fig. 3), which indicates that the eqn (1) used here is suitable to study the interaction between flavones and HSA.21–25
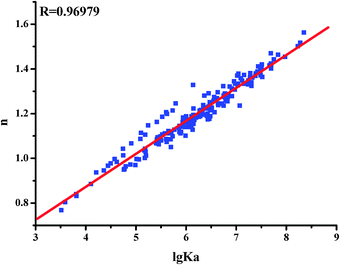 |
| Fig. 3 The relationship between the affinities (lg Ka) and the number of binding sites (n) between flavones and HSA. | |
Effect of glucose on the binding affinities of HSA for flavones
As shown in Fig. 4a, glucose obviously reduces the binding affinities of HSA for 6-hydroxyflavone, 6-methoxyflavone, 3-hydroxyflavone and 7-hydroxyflavone on the first day. As shown in Fig. 4b, the binding affinities of HSA for 6-hydroxyflavone, 6-methoxyflavone, 3-hydroxyflavone and 7-hydroxyflavone were significantly reduced by 1.35 to 25.13 fold. Moreover, glucose hardly influences the binding affinities of HSA for flavone (Fig. 4a). However, glucose obviously improves the binding affinities of HSA for chrysin, 7,8-dihydroxyflavone and baicalein by 1 to 79 times (Fig. 4b), which contain more hydroxyl groups on ring A. It looks like the interactions of HSA and flavones with more hydroxyl groups on ring A are more easily affected by glucose.
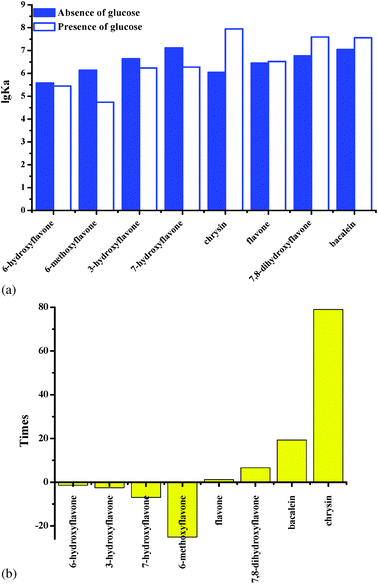 |
| Fig. 4 The influence of glucose on the binding affinities of HSA for the flavones on the first day. | |
The lipophilicity of the flavones under study was assessed by their partition coefficient values (X log P3) according to the PubChem Public Chemical Database. There is a linear relationship between the X log P3 values and lg Ka values (R = 0.68022) of HSA for flavones in the absence of glucose (Fig. 5). Flavones with a higher X log P3 show lower affinities with HSA. However, there is no relationship between the X log P3 values and lg Ka values (R = 0.06817) in the presence of glucose. To further investigate whether or not the hydrogen donor of flavones plays an important role on the flavone–HSA interaction, the relationships of the hydrogen bond donor numbers (data were from the PubChem Public Chemical Database) with the lg Ka values of HSA for flavones in the absence and presence of glucose were determined. The lg Ka values of HSA for flavones in the presence of glucose significantly increased with an increasing number of hydrogen bond donors of flavones (R = 0.75069) (Fig. 6). The flavones with lower hydrogen donor numbers showed a weaker interaction with HSA in the presence of glucose. However, there is no relationship between the hydrogen bond donor numbers and lg Ka values (R = 0.08684) in the absence of glucose. These results indicate that flavones act as a donor of hydrogen bonds with HSA by means of the interaction with glucose.
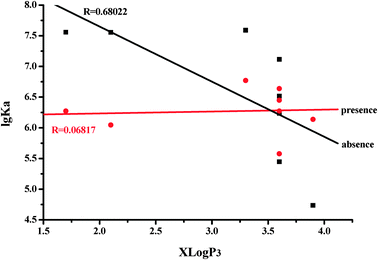 |
| Fig. 5 Relationship between X log P3 values and lg Ka values of the flavone–HSA complex. | |
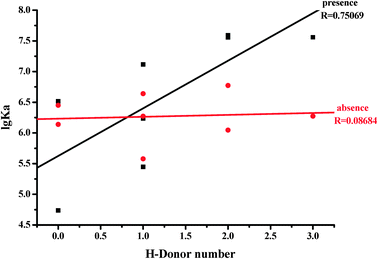 |
| Fig. 6 Relationship between H-donor number and lg Ka values of the flavone–HSA complex. | |
Time course of glucose influencing the flavone–HSA interaction
The binding affinities of HSA for flavones were altered when kept at 37 °C in air from 1 to 23 days. The binding affinity of HSA for 7-hydroxyflavone was hardly changed. The binding affinity of HSA for 7,8-dihydroxyflavone was significantly increased. The binding affinities of HSA for baicalein and chrysin were slightly increased. The binding affinities of HSA for 6-dihydroxyflavone, 6-methoxyflavone, flavone and 3-hydroxyflavone were slightly decreased. As seen from Fig. 7, with increased incubation time in air (from 1 to 23 days), the binding affinities of HSA for multi-hydroxyl flavones on ring A were obviously higher than those of non- or mono-hydroxyl flavones on ring A. We have reported that the hydroxylation on ring A of the flavones increased the binding constants and the number of binding sites between flavones and serum albumins.26 Moreover, it appears that the optimal number of hydroxyl groups introduced to ring A of the flavones is one or two.
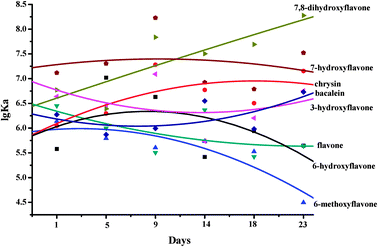 |
| Fig. 7 Time course of glucose influencing the flavone–HSA interaction. | |
As shown in Fig. 8, glucose obviously reduces the binding affinities of HSA for 6-hydroxyflavone, 6-methoxyflavone, 3-hydroxyflavone and 7-hydroxyflavone when kept at 37 °C in air from 1 to 23 days. With increasing time in air, the binding affinity of HSA for 6-methoxyflavone was significantly decreased by 25.13 to 1.43 times. However, the binding affinity between 6-hydroxyflavone and HSA was obviously increased by 1.35 to 6.98 fold. Moreover, the binding affinities of HSA for 3-hydroxyflavone and 7-hydroxyflavone were slightly changed with increasing days at 37 °C in air.
As seen from Fig. 9, glucose significantly enhances the binding affinities of HSA for baicalein when kept at 37 °C in air from 1 to 23 days. Moreover, glucose obviously improves the binding affinities of HSA for chrysin and 7,8-dihydroxyflavone when kept at 37 °C in air from 1 to 5 days. From the 9th day, the binding affinities of HSA for chrysin and 7,8-dihydroxyflavone decreased. Glucose hardly influences the binding affinity of HSA for flavone when kept at 37 °C in air from 1 to 23 days.
The time course of the observed modifications indicated that the form of glycation products changed as HSA was incubated over longer periods of time with glucose.27 Several regions with significant levels of modification are at or near the major drug binding sites on HSA. These results explain why the interaction of flavone with HSA has been observed to vary with the level of glycation for this protein.
HSA masked the DPPH radical scavenging potential of 7,8-dihydroxyflavone and baicalein
The working solutions of flavones (1.0 × 10−3 mol L−1) were diluted ten times with MilliQ water or HSA solution (1.0 × 10−5 mol L−1) to obtain tested samples. The immediate DPPH radical scavenging activities of the tested samples were determined in order to illustrate the effect of HSA on the DPPH scavenging potential of the flavones. The result shows that 7,8-dihydroxyflavone and baicalein exhibit strong DPPH scavenging percentages and 3-hydroxyflavone shows very weak scavenging activity. HSA significantly masked the DPPH radical scavenging potential of 7,8-dihydroxyflavone and baicalein. The DPPH radical scavenging percentages of 7,8-dihydroxyflavone and baicalein are 85.71% and 70.12% in the absence of HSA. However, the DPPH radical scavenging percentages of 7,8-dihydroxyflavone and baicalein reduce to 77.80% and 49.36% in the presence of HSA, respectively.
It looks like the effect of the flavone–protein interaction on the bioavailability of flavones is not equivocal and the interaction of flavones with proteins will weaken the antioxidant capacity of the flavonoids. Smith et al. found that the binding of polyphenols, such as quercetin, fisetin, myricetin and morin, to albumin reduced their pro-oxidant activity.28 Arts et al. showed that the antioxidant capacity of quercetin, rutin and (+)-catechin in blood plasma was not additive.29 Arts et al. further checked the effect of the flavonoid–protein interaction on the antioxidant capacity of flavonoids with the Trolox equivalent antioxidant capacity (TEAC) assay.30 Bae et al. indicated that (−)-epigallocatechin gallate (EGCG) is stable in human serum and HSA stabilized EGCG under aerobic conditions and suggested that the antioxidant property and the binding capacity of HSA contribute to the stabilization of EGCG in human serum.31 Ishii et al. indicated that the galloyl moiety participated in the interaction of EGCG with HSA and that this interaction was of critical importance in preventing EGCG oxidation.32
Influence of glucose on the DPPH radical scavenging potential of the flavone–HSA system
The working solutions of flavones (1.0 × 10−3 mol L−1) were diluted ten times with HSA solution (1.0 × 10−5 mol L−1), MilliQ water, MilliQ water with glucose (100 mmol L−1) or HSA solution (1.0 × 10−5 mol L−1) with glucose (100 mmol L−1) to obtain tested samples. The immediate DPPH radical scavenging activities of the tested samples were determined in order to illustrate the influence of glucose on the DPPH scavenging potential of the flavone–HSA system. Glucose hardly influences the DPPH scavenging potential of flavones in the absence of HSA. However, glucose obviously enhances the DPPH scavenging potential of the baicalein–HSA system. Moreover, glucose slightly weakens the DPPH scavenging potential of the 7,8-dihydroxyflavone–HSA system.
It was reported that the increasing DPPH scavenging percentages of polyphenols slightly decreased with increasing affinities of polyphenol to BSA.33 We also found that the decreasing DPPH scavenging percentages of polyphenols increased with increasing affinities of milk protein–polyphenol complexes.34 Here, it is hypothesized that the antioxidant ability of the flavone–HSA system does not depend on the sum of the antioxidant ability of flavone molecules bound but simply on the number of flavones bound.
Acknowledgements
The authors are grateful for financial sponsorship by National Natural Science Fund (21175155) and China Postdoctoral Science Foundation (20110490474).
References
- H. Kolodziej and A. E. Hagerman, Planta Med., 2011, 77, 1069–1070 CrossRef CAS.
- M. C. Bohin, J. P. Vincken, H. T. W. M. van der Hijden and H. Gruppen, J. Agric. Food Chem., 2012, 60, 4136–4143 CrossRef CAS.
- G. Williamson, F. Dionisi and M. Renouf, Mol. Nutr. Food Res., 2011, 55, 864–873 CAS.
- S. Roowi and A. Crozier, J. Agric. Food Chem., 2011, 59, 12217–12225 CrossRef CAS.
- J. M. Yuan, Mol. Nutr. Food Res., 2011, 55, 886–904 CAS.
- J. N. Fletcher, A. D. Kinghorn, J. P. Slack, T. S. McCluskey, A. Odley and Z. H. Jia, J. Agric. Food Chem., 2011, 59, 13117–13121 CrossRef CAS.
- M. H. Omar, W. Mullen and A. Crozier, J. Agric. Food Chem., 2011, 59, 1363–1369 CrossRef CAS.
- G. Hostetler, K. Riedl, H. Cardenas, M. Diosa-Toro, D. Arango, S. Schwartz and A. I. Doseff, Mol. Nutr. Food Res., 2012, 56, 558–569 CAS.
- M. Claussnitzer, T. Skurk, H. Hauner, H. Daniel and M. J. Rist, Mol. Nutr. Food Res., 2011, 55, S26–S34 CAS.
- K. Nakajou, H. Watanabe, U. Kragh-Hansen, T. Maruyama and M. Otagiri, Biochim. Biophys. Acta, Gen. Subj., 2003, 1623, 88–97 CrossRef CAS.
- P. J. Coussons, J. Jacoby, A. McKay, S. M. Kelly, N. C. Price and J. V. Hunt, Free Radical Biol. Med., 1997, 22, 1217–1227 CrossRef CAS.
- A. Barzegar, A. A. Moosavi-Movahedi, N. Sattarahmady, M. A. Hosseinpour-Faizi, M. Aminbakhsh and F. Ahmad,
et al.
, Protein Pept. Lett., 2007, 14, 13–18 CrossRef CAS.
- K. Bala, J. Gomes and N. K. Gohil, Mol. BioSyst., 2011, 7, 3036–3041 RSC.
- J. D. Trombley, T. N. Loegel, N. D. Danielson and A. E. Hagerman, Anal. Bioanal. Chem., 2011, 401, 1523–1529 CrossRef.
- Y. P. Zhang, S. Y. Shi, X. Q. Chen, W. Zhang, K. L. Huang and M. J. Peng, J. Agric. Food Chem., 2011, 59, 8499–8506 CrossRef CAS.
- X. R. Pan, P. F. Qin, R. T. Liu and J. Wang, J. Agric. Food Chem., 2011, 59, 6650–6656 CrossRef CAS.
- J. B. Xiao and G. Y. Kai, Crit. Rev. Food Sci. Nutr., 2012, 52, 85–101 CrossRef CAS.
- J. B. Xiao, Y. R. Zhao, H. Wang, Y. M. Yuan, F. Yang and C. Zhang,
et al.
, Integr. Biol., 2011, 3, 1087–1094 RSC.
- Y. X. Xie, J. B. Xiao, G. Y. Kai and X. Q. Chen, Integr. Biol., 2012, 4, 502–507 RSC.
- P. Bandyopadhyay, A. K. Ghosh and C. Ghosh, Food Funct., 2012, 3, 592–605 CAS.
- J. B. Xiao, G. Y. Kai, F. Yang, C. X. Liu, X. C. Xu and K. Yamamoto, Mol. Nutr. Food Res., 2011, 55, S86–S92 CAS.
- J. B. Xiao, T. T. Chen, H. Cao, L. S. Chen and F. Yang, Mol. Nutr. Food Res., 2011, 55, 310–317 CAS.
- J. B. Xiao, H. Cao, Y. F. Wang, J. Y. Zhao and X. L. Wei, J. Agric. Food Chem., 2009, 57, 6642–6648 CrossRef CAS.
- J. B. Xiao, Y. R. Zhao, H. Wang, Y. M. Yuan, F. Yang and C. Zhang,
et al.
, J. Agric. Food Chem., 2011, 59, 10747–10754 CrossRef CAS.
- J. B. Xiao, J. L. Huo, F. Yang and X. Q. Chen, J. Agric. Food Chem., 2011, 59, 8484–8490 CrossRef CAS.
- J. B. Xiao, H. Cao, Y. F. Wang, K. Yamamoto and X. L. Wei, Mol. Nutr. Food Res., 2010, 54, S253–S260 CAS.
- O. S. Barnaby, R. L. Cerny, W. Clarke and D. S. Hage, Clin. Chim. Acta, 2011, 412, 1606–1615 CrossRef CAS.
- C. Smith, B. Halliwell and O. Aruoma, Food Chem. Toxicol., 1992, 30, 483–489 CrossRef CAS.
- M. J. T. J. Arts, G. R. M. M. Haenen, H. P. Voss and A. Bast, Food Chem. Toxicol., 2001, 39, 787–791 CrossRef CAS.
- M. J. T. J. Arts, G. R. M. M. Haenen, L. C. Wilms, S. A. J. N. Beetstra, C. G. M. Heijnen and H. P. Voss, J. Agric. Food Chem., 2002, 50, 1184–1187 CrossRef CAS.
- M. J. Bae, T. Ishii, K. Minoda, Y. Kawada, T. Ichikawa and T. Mori, Mol. Nutr. Food Res., 2009, 53, 709–715 CAS.
- T. Ishii, T. Ichikawa, K. Minoda, K. Kusaka, S. Ito and Y. Suzuki,
et al.
, Biosci., Biotechnol., Biochem., 2011, 75, 100–106 CrossRef CAS.
- H. Cao, X. Q. Chen and K. Yamamoto, Anti-Cancer Agents Med. Chem., 2012, 12, 940–948 CrossRef CAS.
- J. B. Xiao, F. F. Mao, F. Yang, Y. L. Zhao, C. Zhang and K. Yamamoto, Mol. Nutr. Food Res., 2011, 55, 1637–1645 CAS.
|
This journal is © The Royal Society of Chemistry 2013 |