DOI:
10.1039/D2AY01701F
(Paper)
Anal. Methods, 2023,
15, 17-26
A rhodamine based chemodosimeter for the detection of Group 13 metal ions†
Received
20th October 2022
, Accepted 15th November 2022
First published on 15th November 2022
Abstract
A new rhodamine derivative, HL-CIN, derived from a reaction between N-(rhodamine-6G)lactam-ethylenediamine (L1) and trans-cinnamaldehyde, is reported here for the colorimetric and fluorogenic sensing of Group 13 trivalent cations, namely Al3+, Ga3+, In3+ and Tl3+. The absorption intensity of the probe increases significantly at 530 nm whereas the fluorescence intensity enhances massively at 558 nm upon interaction with these metal ions. Other relevant metal ions could not impart any noticeable color change or fluorescence enhancement. The quantum yield or fluorescence life time of HL-CIN increases considerably in the presence of these Group 13 metal ions. Different spectral studies such as ESI-mass, FT-IR, 1H and 13C NMR spectra, establish that HL-CIN undergoes hydrolysis in the presence of the trivalent cations and a rhodamine species in its ring opened form (i.e. N-(2-aminoethyl)-2-((6Z)-3-(ethylamino)-6-(ethylimino)-2,7-dimethyl-6H-xanthen-9-yl)benzamide, (L2)) along with cinnamaldehyde are produced. The rhodamine species in its ring opened form (L2) is responsible for the color change and strong increment in the absorbance and fluorescence of HL-CIN with Group 13 cations. Interaction between L1 and these metal ions could not produce the same outcome. It has been used in test paper strips and to detect these cations in real samples.
Introduction
Identification of metal ions is of enormous importance for researchers of various fields such as chemical, biological and environmental sciences as they play key roles in many chemical and physiological processes. Various methods are available for this purpose. Use of fluorescent chemosensors is one of them. This method offers few advantages such as high selectivity, high sensitivity, quick response, simple operation, low cost, etc. For these reasons, fluorescence sensing becomes a method of choice for scientists for the detection of several metal ions, anions, and others. Different fluorophores are being utilized in the construction of chemosensors.1–5 One chemosensor can detect one metal ion, two metal ions or more than two metal ions under similar or different conditions.1–7 It is always beneficial if a chemosensor is able to detect or sense two or more metal ions by producing different signals such as two emission wavelengths as the output as it saves cost and labor. With the increase in the number of metal ions detected by using a single probe, chances of producing different outputs also decrease. In such cases, usually a single output signal is observed. So, multiple ions could not be identified separately in such cases. However, effort has been going on to develop multi-ion sensing systems to get better results. In this regard, it could be mentioned that rhodamine based chemosensors produce strong fluorescence in the presence of suitable metal ions.8 Depending on substitutions, rhodamine derivatives were reported as chemosensors for multi-analyte sensors; particularly few of them are selective for trivalent cations of aluminum, chromium, and iron.9–12 Recently, our group reported a rhodamine based chemosensor for the selective detection of Group 13 cations.13 That was probably the first report of a rhodamine based dye for the identification of Group 13 cations.
Trivalent cations of Group 13 are Al3+, Ga3+, In3+ and Tl3+. These metals are nonessential in the biological domain.14 These metals or their ions are of significant importance in health issues, various fields of applications, etc. Aluminum is present as the most available metal on the earth's surface. It is used in the paper, textile and food industries. It is used as a utensil in domestic and commercial use. Food wrapping is done with this metal. High deposition of this metal can create some nerve related issues such as Parkinson's disease.5 High concentration of aluminum is detrimental to flora and fauna. Although gallium is used as a catalyst,15,16 luminescent material,17,18 phosphor material,19 in solar cells20 and up-conversion emission,21etc., it has potential ability to cause health hazard.22–24 Indium is used in different electronic materials such as computers, television, mobile phones,25,26 coating material,27etc. Indium-contaminated soil is a threat to human health when it is used in food manufacture.28 However, its exposure route to human has not been established yet. It has bad effects on heart, kidney, and liver.29 Thallium is considered as an extremely toxic metal.30 It is used to remove facial hair, to treat fungal infections of the scalp, as a catalyst in the oxidation of hydrocarbons, and epoxidation and polymerization reactions.31 Thus, it is necessary to detect these metal ions to understand their presence and role in different processes.
Rhodamine is one of the widely used fluorophores for the development of chemosensors because of the high molar extinction coefficient, good photostability with respect to other organic fluorophores, large quantum yield, longer excitation and emission wavelength, etc.32 Most of the rhodamine based fluorescent sensors are used for sensing of single, two or three metal ions. These are used to detect three trivalent cations, namely, Al3+, Cr3+ and Fe3+ ions.9–12 To the best of our knowledge, there is only one instance of a report on rhodamine based chemosensors for the detection of Group 13 cations.13
We report here chemodosimetric behavior of a rhodamine based molecule, HL-CIN, towards detection of Group 13 metal ions (Scheme 1). HL-CIN has been obtained from the Schiff base condensation reaction between N-(rhodamine-6G)lactam-ethylenediamine, (L1) and trans-cinnamaldehyde in methanol. Our group is working on the development of rhodamine based chemosensors for quite a time and trying to correlate between metal selectivity and substitution on rhodamine derivatives.33–37 To examine further on the effect of substitution, we chose here trans-cinnamaldehyde to condense with L1 and found to be a selective chemosensor for Group 13 metal ions.
 |
| Scheme 1 Synthetic route to HL-CIN. | |
Experimental section
Material & physical measurements
Details of materials, solvents, instruments, spectral measurement techniques, etc. are given in the ESI.† Quantum yields of HL-CIN and HL-CIN with different Group 13 metal ions were determined by following a published procedure.38
Synthesis of bis(ethylamino)-2′,7′-dimethyl-2-(2-((E)-((E)-3-phenylallylidene)amino)ethyl)spiro[isoindoline-1,9′-xanthen]-3-one (HL-CIN)
L1 was synthesized following a published method.39 A 10 mL of methanolic solution of trans-cinnamaldehyde (31.47 μL, 0.25 mmol) was added to another methanolic solution (10 mL) of L1 (0.114 g, 0.25 mmol) under continuous stirring conditions and stirring was continued for 30 min when a clear solution was obtained. Then the mixture was refluxed for 6 h. It was then cooled to room temperature. The mixture was filtered to separate any precipitate or suspended material and the filtrate was kept in a dark place for few days. On slow evaporation of methanol solvent, yellow crystals of HL-CIN were obtained. Yield: (0.114 g, 80.2%). Anal. calc. for C37H38N4O2: C, 77.87; H, 6.71; N, 9.82; found: C, 77.81; H, 6.65; N, 9.74; 1H NMR (DMSO-d6, 300 MHz) (δ, ppm): 7.94 (d, J = 6 Hz, 1H), 7.78 (d, J = 8 Hz, 2H), 7.57–7.51 (m, 3H), 7.35–7.31 (m, 4H), 6.93 (d, J = 14 Hz, 1H), 6.71 (t, J = 12 Hz, 1H), 6.28 (s, 1H), 6.11 (s, 1H), 5.07 (t, J = 5 Hz, 2H), 4.46 (t, J = 6 Hz, 4H), 3.15 (q, J1 = 6.0 Hz, J2 = 6.9 Hz, 4H), 1.81 (s, 6H), 1.20 (t, J = 7.2, 6H), 1.05 (t, J = 7.2, 4H). 13C NMR (DMSO-d6, 75 MHz) (δ, ppm): 167.08, 163.20, 154.12, 151.11, 148.16, 141.62, 136.02, 133.07, 132.70, 130.42, 128.14, 127.82, 127.47, 118.92, 104.53, 96.14, 65.04, 58.43, 37.41, 18.54, 14.34; ESI-MS+ (m/z): 571.31 [(HL-CIN + H+)]; FT-IR: 1670 cm−1 (
C
O), 1632 cm−1 (
C
N).
Results and discussion
Visual changes in the color of HL-CIN
First changes in the color of HL-CIN have been examined in the presence of various metal ions such as Al3+, Zn2+, Pb2+, Ni2+, Na+, Mg2+, K+, Hg2+, Fe3+, Cu2+, Cr3+,Ga3+, In3+, Tl3+, Cd2+ and Ca2+ ions in 10 mM HEPES buffer in water
:
ethanol (1
:
9, v/v) (pH 7.4) under visible and UV light. Its color alters from colorless to bright pink in the presence of the Group 13 cations viz. Al3+, Ga3+, In3+ and Tl3+ among the metal ions under consideration (Fig. 1). Other metal ions could not produce any change in the color of the probe solution. When viewed under UV light, the same set of trivalent cations is able to produce bright canary yellow coloration of the probe solution. Other cations are unable to do so. Thus, HL-CIN could be a selective probe for these Group 13 cations.
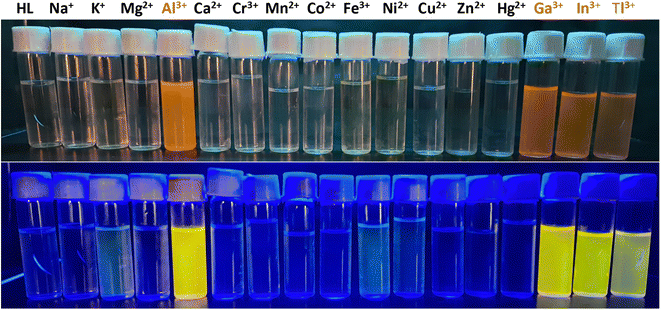 |
| Fig. 1 Images of HL-CIN and HL-CIN in the presence of various metal cations viewed under visible light (upper row) and UV light (lower row). | |
Measurement of UV-vis spectra of HL-CIN (40 μM) has been done in 10 mM HEPES buffer in water
:
ethanol (1
:
9, v/v) (pH 7.4) in the presence of different metal ions. The free chemosensor shows no peak in the region of 450 to 650 nm. Most of the metal ions could not alter its spectral behavior in this range (Fig. S1†). However, in the presence of Group 13 metal ions, a peak emerges at 530 nm in the absorption spectrum of the probe (Fig. 2). With the gradual addition of the metal ion, absorbance at 530 nm increases in the UV-vis spectrum of the probe until the ratio of the probe to metal ion reaches 1
:
1. There is a huge increment in the absorbance of HL-CIN at 530 nm in the presence of the trivalent cations (Table 1). The generation of a pink color and appearance of an absorption peak at 530 nm are attributed to the ring opened form of the rhodamine derivative. Thus, these trivalent Group 13 metal ions are able to increase the absorbance of the probe at 530 nm selectively.
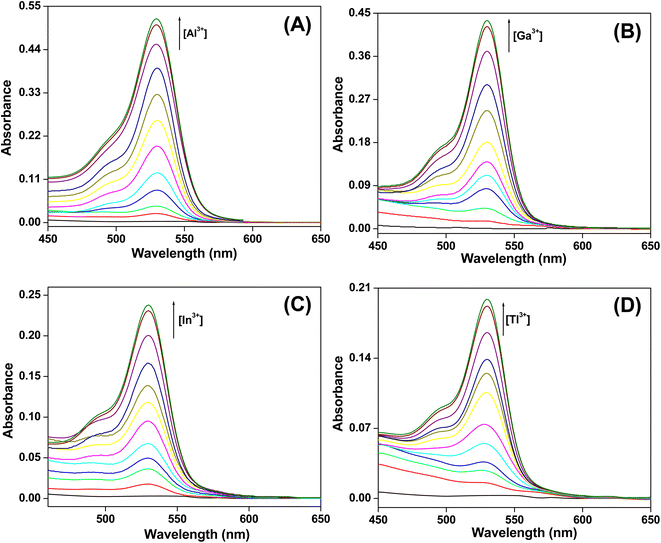 |
| Fig. 2 Absorption spectra of HL-CIN (40 μM) in the presence of 0, 4, 8, …, 40 and 44 μM (A) Al3+, (B) Ga3+, (C) In3+ and (D) Tl3+ in 10 mM HEPES buffer in H2O/ethanol = 1 : 9 (v/v; pH = 7.4) at room temperature. | |
Table 1 Increment in absorbance and fluorescence intensity, quantum yield, life time and LOD values of HL-CIN upon interaction with Group 13 metal ions
Species |
Increment in absorption intensity at 530 nm (fold) |
Increment in fluorescence intensity at 558 nm (fold) |
Quantum yield |
Life-time (ns) |
LOD (×10−8 M) |
HL-CIN
|
— |
— |
0.002 |
3.75 |
— |
HL-CIN + Al3+ |
168 |
263 |
0.495 |
4.06 |
2.8 |
HL-CIN + Ga3+ |
141 |
261 |
0.493 |
3.93 |
2.9 |
HL-CIN + In3+ |
76 |
126 |
0.246 |
3.95 |
5.6 |
HL-CIN + Tl3+ |
64 |
83 |
0.163 |
3.96 |
8.2 |
Fluorescence properties
In the presence and absence of different metal cations such as Al3+, Zn2+, Pb2+, Ni2+, Na+, Mg2+, K+, Hg2+, Fe3+, Cu2+, Cr3+, Ga3+, In3+, Tl3+, Cd2+ and Ca2+, fluorescence spectra of HL-CIN have been obtained in 10 mM HEPES buffer in H2O/EtOH = 1
:
9 (v/v) (pH 7.4) at room temperature. Free HL-CIN does not produce any noticeable peak upon excitation at 495 nm. Among different cations only Group 13 metal cations i.e. Al3+, Ga3+, In3+ and Tl3+ are able to generate an emission band at 558 nm (Fig. 3) and with the increase in the concentration of these cations, the fluorescence intensity at 558 nm increases gradually. After addition of one equivalent each of these metal cations, the increment in fluorescence intensity reaches a saturation point with a significant increment in the intensity. Enhancement in the fluorescence intensity of HL-CIN by Group 13 cations is attributed to the open form of the spirolactam ring of the rhodamine derivative. Other cations could not change the fluorescence intensity of the probe significantly (Fig. 4). Thus, HL-CIN becomes a selective chemosensor for Al3+, Ga3+, In3+ and Tl3+ ions.
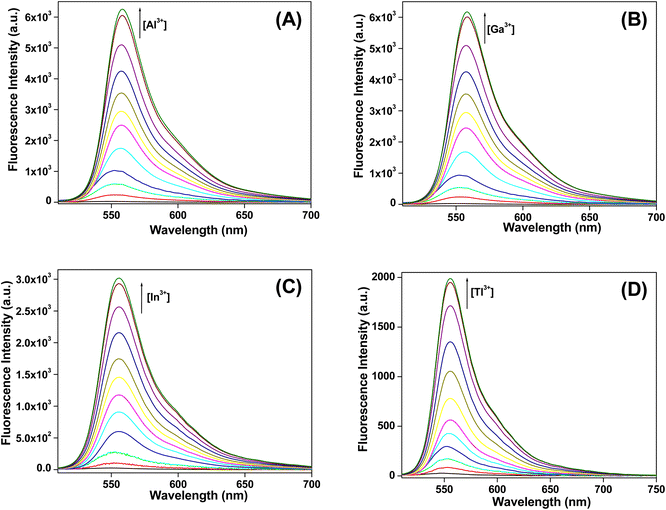 |
| Fig. 3 Fluorescence spectra of HL-CIN (40 μM) in the presence of 0, 4, 8, …, 40 and 44 μM (A) Al3+, (B) Ga3+, (C) In3+ and (D) Tl3+ in 10 mM HEPES buffer in H2O/ethanol = 1 : 9 (v/v; pH = 7.4) at room temperature. | |
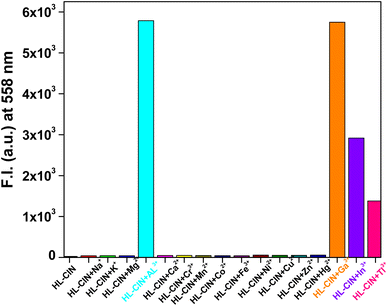 |
| Fig. 4 Fluorescence of HL-CIN (40 μM) in the presence of one equiv. of different metal ions in 10 mM HEPES buffer in H2O/ethanol = 1 : 9 (v/v; pH = 7.4) at room temperature. | |
The quantum yields (φ) of HL-CIN and HL-CIN in the presence of one equivalent of Al3+, Ga3+, In3+and Tl3+ have been measured using rhodamine-6G (standard). The values of the fluorescence quantum yield of HL-CIN and HL-CIN in the presence of one equiv. of the metal ions are given in Table 1. From the table it could be observed that there is a substantial increment in the quantum yield of the probe in the presence of the trivalent metal ions with respect to the free probe. The quantum yield of the probe increases by 247, 246, 123 and 81 fold upon interaction with Al3+, Ga3+, In3+ and Tl3+, respectively.
Time-resolved emission experiment has been done for HL-CIN itself and in the presence of Al3+, Ga3+, In3+ and Tl3+ to study the changes in photophysical properties. The time-correlated single photo counting (TCSPC) method has been followed here for fluorescence life-time measurement. The life time data have been given in Table 1. Decay behaviors HL-CIN and HL-CIN with these cations are given in Fig. S2.† It can be clearly said from the table that the life-time of HL-CIN increases significantly with these cations.
The LOD value is related to the sensitivity of a probe for an analyte. A lower LOD value implies higher sensitivity of a probe. The limit of detection for HL-CIN for these Group 13 metal ions has been determined by the 3σ method.40 Details and related graphs (Fig. S3–S7) are given in the ESI.† The LOD values of the sensor towards these ions are given in Table 1. It may be concluded here that HL-CIN shows significantly high sensitivity towards all of the Group 13 metal cations.
The effect of pH on fluorescence intensity has been investigated for HL-CIN and HL-CIN with Group 13 metal ions in the pH range of 2 to 12 (Fig. S8†). From Fig. S8,† it is observed that the free probe shows high fluorescence intensity under acidic conditions up to pH 5; after that the intensity decreases and in the presence of Group-13 metal cations the fluorescence intensity is significantly high up to pH 9. From this observation it can be concluded that under acidic conditions the spirolactam ring is opened in the rhodamine derivative, therefore, the free probe shows high intensity under acidic conditions. After adding metal ions the intensity is high up to pH 9 due to the existence of a spirocyclic ring opened species. Trivalent cations induce breaking of the imine bond and generation of ring opened species (vide infra). Thus, in the pH range of 5 to 9, high fluorescence intensity is attributed to the presence of M3+ along with HL-CIN.
Mechanism
Rhodamine derivatives are colorless and negligibly fluorescent when the spirolactam ring in them remains in the closed form. When the spirocyclic ring is opened in the presence of external stimuli such as metal ions, they show a pink color and strong fluorescence.8 As HL-CIN remains in colorless and nonfluorescent condition before interaction with Group 13 metals, it indicates that the spirocyclic ring is in the closed state and after interaction with the metal ions, the spiro ring opens up. Enhancement in the fluorescence of HL-CIN induced by the interaction with trivalent cations has been proposed here to occur by the path as depicted in Scheme 2. Metal ions are known to catalyze the hydrolysis of the imine bond of chemosensors on few occasions and to control their fluorescence properties.41–43 The imine bond in HL-CIN is broken in the presence of Group 13 metal ions and a spirocyclic ring opened species (L2) is generated to impart the color change and strong fluorescence.
 |
| Scheme 2 Generation of fluorescent moiety, L2, from HL-CIN induced by trivalent metal ions. The M3+ ion induces the hydrolysis of the chemosensor and opening of the spirolactam ring leading to the generation of a pink color and strong fluorescence enhancement. | |
The increment in the absorbance at 530 nm and fluorescence at 558 nm occurs by the hydrolysis of HL-CIN induced by trivalent metal ions. The free rhodamine derivative, HL-CIN, shows an m/z peak at 571.31 which may be attributed to the presence of [HL-CIN + H+] species (Fig. S9†). Mass spectra of the mass obtained after interaction of HL-CIN with different trivalent cations separately have been recorded and an m/z peak at ∼457.5 for each of the cations has been observed. The mass spectrum of HL-CIN with Al3+ has been given in Fig. S10† as a representative case. The peak at ∼457.5 may be attributed to (L2 + H+) species which is assigned to the hydrolyzed product of HL-CIN.
IR spectral analysis also supports the formation of cinnamaldehyde. The FTIR spectrum of HL-CIN exhibits two important peaks at 1670 and 1632 cm−1 which may be due to the existence of amide and azomethine functionalities, respectively (Fig. S11†). Upon interaction with Al3+, the peak at 1670 cm−1 undergoes a shift to 1640 cm−1. However, a new band in the IR spectrum appears at 1728 cm−1 which indicates the generation of an aldehyde group. The FT-IR spectrum of HL-CIN in the presence of Al3+ has been given in Fig. S12† as a representative case. Upon hydrolysis of HL-CIN, cinnamaldehyde is produced and it shows the band of the aldehyde group. Thus, hydrolysis of HL-CIN induced by trivalent Group 13 cations produces cinnamaldehyde along with L2.
Presence of L2 and occurrence of hydrolysis have further been confirmed by NMR spectral studies. 1H NMR spectra of HL-CIN in the absence and in the presence of trivalent metal ions have been obtained in DMSO-d6 in order to check any changes in the structure of the probe (Fig. 5 and S22†). The peak at 8.12 ppm may be assigned to the peak of an azomethine proton confirming the formation of the Schiff base molecule (Fig. 5A). The peaks at 1.19 and 1.86 ppm are due to the presence of two different types of methyl groups. The three different types of methylene protons give signals at 1.05, 3.14 and 4.47 ppm. The peaks at 6.97 and 6.70 ppm may be assigned to the olefinic protons of the cinnamaldehyde unit. Peaks for various aromatic protons emerge in their usual positions. There are significant changes in the 1H NMR spectrum of HL-CIN in the presence of Al3+ (Fig. 5B). A doublet peak appears at 9.68 ppm which may be attributed to the presence of the proton of the aldehyde group of the cinnamaldehyde. This peak was absent in the case of the free probe. The peaks at 6.98 and 6.86 ppm may be attributed to the presence of the olefinic protons of cinnamaldehyde (Fig. S13†). Peaks of aromatic protons of cinnamaldehyde emerge in the region of 7.51 to 7.74 ppm. This suggests the generation of cinnamaldehyde which is a possible product of hydrolysis of HL-CIN. Generation of L2 is confirmed by the appearance of peaks at 1.18 and 1.86 ppm (methyl protons), 3.13 and 3.25 ppm (methylene protons), 2.89 and 5.09 ppm (NH protons), 2.39 ppm (–NH2 protons) and in the range of 7.51 to 7.74 ppm (aromatic protons) (Fig. S14†). Thus, it has been found that Al3+ catalyzes the hydrolysis of HL-CIN to give fluorescent component, L2 and cinnamaldehyde. The analyses of other 1H NMR spectra of HL-CIN in the presence of Ga3+, In3+ and Tl3+ also reveal the hydrolysis of HL-CIN to give cinnamaldehyde and L2 (Fig. S15–S17†).
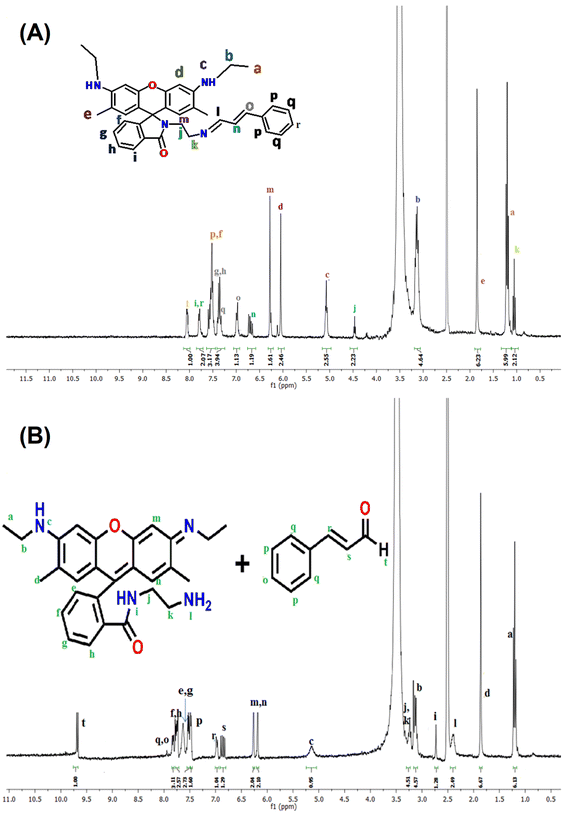 |
| Fig. 5
1H NMR spectra of (A) HL-CIN and (B) HL-CIN in the presence of Al3+ in DMSO-d6. | |
13C NMR spectra of HL-CIN in the absence and in the presence of different trivalent metal ions have been obtained in DMSO-d6 in order to support the proposed mechanism. In the absence of any cation, HL-CIN exhibits peaks at 14.4, 17.1, 37.6 and 56.8 ppm indicating the presence of various methyl and methylene carbons (Fig. S18†). The peak at 64.9 ppm confirms the presence of the spirolactam ring in its closed form. The other important peak at 167.0 ppm indicates the presence of carbonyl carbon while that at 163.5 confirms the existence of azomethine carbon. Peaks for other carbons (aromatic and ethylenic) appear in their usual positions. In the presence of trivalent cations such as Al3+, there are changes in the 13C NMR spectrum of HL-CIN (Fig. S19†). The peak at 64.9 ppm disappears indicating the opening of the spirolactam ring. It is worth mentioning here that the starting material, rhodamine derivative (L1) does show a peak at around 64 ppm in its 13C NMR spectrum confirming the presence of the closed spiro ring(Fig. S20†). Another important peak emerges at 195.1 ppm which indicates the presence of the aldehyde group. This peak was absent in the case of free HL-CIN. The 13C NMR spectrum of the free cinnamaldehyde shows a peak at the same position (Fig. S21†). The study establishes that in the presence of trivalent cations, HL-CIN undergoes hydrolysis to produce cinnamaldehyde and spiroring opened rhodamine derivative, L2. Thus, the trivalent metal ion is able to catalyze the hydrolysis of the probe and to produce the rhodamine derivative responsible for pink coloration and strong fluorescence.
Different solvents have been used to check the effect of solvent on the fluorescence sensing ability of HL-CIN. For this purpose, sensing experiments have been carried out in pure ethanol and acetonitrile. There is no color change of HL-CIN upon addition of the trivalent cations in both the solvents. Absorption spectra of the chemosensor have been obtained in these solvents in the absence and in the presence of Al3+ ions (representative case) (Fig. S23†). It could be clearly observed that there is no change in absorption spectra, particularly in the range of 500–550 nm. This simply signifies that HL-CIN is not able to sense these cations in pure organic solvents.
We have checked the response time for the color change of HL-CIN in the presence of the Group 13 trivalent cations. It has been found that a change in the color from colorless to pink is instantaneous. Absorption spectra of HL-CIN in the presence of Al3+ (representative case) have been recorded with various time intervals (Fig. S24†). It has also been observed that there was almost no change in the absorption spectra upon addition of additional Al3+ into the probe solution. This suggests that the increment in fluorescence occurs mainly because of hydrolysis of HL-CIN.
From the above discussion it is clear that trivalent cations induce hydrolysis of HL-CIN and opening of the spirocyclic ring to produce L2 (Scheme 2). Addition of trans-cinnamaldehyde to the rhodamine unit, L1, to generate HL-CIN (Scheme 1) is necessary to detect all of these Group 13 cations. To verify this assumption, fluorescence spectra of L1 have been measured in the presence of Al3+, Ga3+, In3+ and Tl3+ ions. It has been found that its fluorescence intensity does not change appreciably in the presence of Ga3+, In3+ and Tl3+ (Fig. S25†). However, its intensity increases with the addition of one equivalent Al3+ ions44 but the magnitude is small in comparison to the increment with HL-CIN. The aluminum ion could induce pink coloration with L1 but other cations could not induce any observable color change with L1. Again, L1 is colorless and nonfluorescent before its interaction with Al3+ indicating the existence of the spirolactam ring in its closed state. The fluorescence increment and pink coloration of the probe after its interaction with the metal ion are attributed to the opening of the spirolactam ring.
Applications
Paper strip experiments
The paper strip experiment has been done following published literature9,45,46 in order to find application of HL-CIN. Paper strips soaked with solution of HL-CIN have been dried in air. Then they are treated with solutions containing Al3+, Ga3+, In3+ and Tl3+ separately. These treated papers are dried in air. Images of these strips under visible light as well under UV light are shown in Fig. 6. The paper strip soaked with the probe does not show any color. But the strips treated with different metal salts exhibit a pink color under visible light. The strip soaked with the probe and the strips treated with trivalent cations are blue and yellowish, respectively, in color when viewed under UV light. The changes are in accordance with the results obtained in Fig. 1.
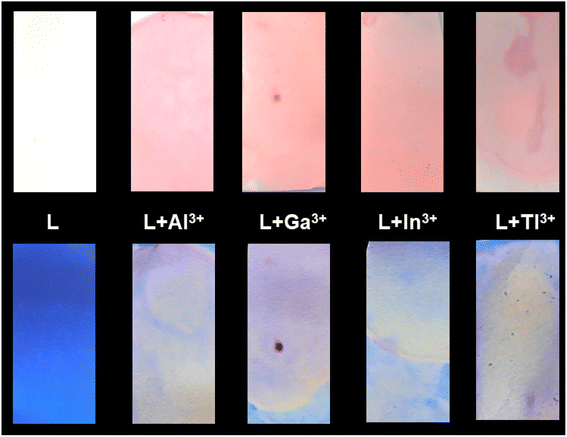 |
| Fig. 6 Color changes of HL-CIN and HL-CIN in the presence of Group 13 metal ions under visible light (upper row) and UV light (lower row). | |
Real sample analysis
River water has been used to examine metal ion identification ability of HL-CIN in a ‘real sample’. Water for this experiment has been collected from River Ganga at Rishikesh, Uttarakhand hoping that many other dissolved materials might be present and interfere with the detection process. The buffer solution has been prepared with this water. Images of HL-CIN and HL-CIN in the presence of Al3+, Ga3+, In3+ and Tl3+ ions in the solution under visible light as well as under UV light are given in Fig. S26.† Under visible light, it is clear that trivalent metal ions produce a pink color while under UV light, the color is bright canary yellow. These results are in harmony with the results presented in Fig. 1.
Waste water from a saloon has been used as a ‘real sample’. In this water, there will be alum, soap, some organic compounds, etc. It is expected that Al3+ ions are present in the waste water. Paper strips with and without treatment with waste water are observed under visible light as well as under UV light (Fig. S27†). The paper strip turns pink on treatment with waste water when viewed under visible light while the pink paper becomes yellow under UV light. These observations indicate that HL-CIN could be used to identify the trivalent metal ion in waste water from a saloon.
Comparative discussion
A comparative discussion has been done with some of the recently reported rhodamine based chemosensors.13,35–37,47–58 Different parameters of these rhodamine based chemosensors are given in Table S1.† In all of the cases, excitation and emission wavelengths are in the visible range. The limit of detection ranges from micromolar order to nanomolar. In the present case, the LOD is in the order of 10−8 M indicating excellent sensitivity. Hydrolysis of the rhodamine based probe does not occur frequently. In this table, it is seen that some of the probes (Table S1,† entries 2, 4, 6, 8) including the present one (Table S1,† entry 17) undergo hydrolysis. However, hydrolysis of the chemosensors occurs in the presence of a metal ion. In the present study, it occurs with Group 13 cations. Thus, HL-CIN could be used to detect any of the Group 13 metal ions. Apart from a previous report (Table S1,† entry 16) of a rhodamine derivative, this probe is able to detect these Group 13 trivalent cations. But the mechanisms are different. In the previous study, cations induce opening of the spirocyclic ring but in the present case, cations induce hydrolysis and subsequent ring opening.
Conclusions
A new rhodamine derivative (HL-CIN) has been obtained by the condensation between N-(rhodamine-6G)lactam-ethylenediamine (L1) and trans cinnamaldehyde and characterized satisfactorily by different methods. It produces pink coloration and a steep increment in absorbance at 530 nm by interacting with Group 13 cations, namely, Al3+, Ga3+, In3+ and Tl3+ ions. Fluorescence intensity of the sensor molecule at 558 nm increases significantly only in the presence of these trivalent cations. It has been seen that its quantum yield and fluorescence life time also enhance on interaction with Al3+, Ga3+, In3+ and Tl3+ ions separately. We have established with the help of ESI-mass, FT-IR, 1H and 13C NMR spectral studies that HL-CIN undergoes hydrolysis in the presence of Group 13 metal ions to give a strongly fluorescent rhodamine species (L2) which is in its ring opened form and cinnamaldehyde. It is a drawback of the present chemosensor that it could not distinguish among Group 13 cations although it can recognize them effectively. It has been confirmed that the interaction of L1 with Al3+, Ga3+, In3+ and Tl3+ does not produce the same results as observed with HL-CIN. This signifies the importance of the Schiff base condensation reaction to generate HL-CIN. From the comparative studies it could be noticed that rhodamine derivatives with the aldehyde unit without any donor atoms in it probably allows the detection of a large number of trivalent metal ions. More examples may help to draw a conclusion in this context. We are developing other rhodamine derivatives to establish this connection between structures of rhodamine based chemosensors and metal ion selectivity.
Author contributions
Sneha Ghosh: formal analysis, investigation, methodology. Partha Roy: conceptualization, resources, supervision, validation, writing – review & editing.
Conflicts of interest
The authors declare that they have no known competing financial interests or personal relationships that could have appeared to influence the work reported in this paper.
Acknowledgements
PR is thankful to SERB, New Delhi for financial support (File no. EEQ/2021/000117 dated 18 February, 2022). SG wishes to thank UGC, New Delhi for providing her a fellowship. The authors are thankful to Professor Tanurima Bhaumik, Jadavpur University, for her helpful discussion on NMR analysis.
References
- F. Wang, K. Wang, Q. Kong, J. Wang, D. Xi, B. Gu, S. Lu, T. Wei and X. Chen, Coord. Chem. Rev., 2021, 429, 213636 CrossRef.
- C. I. David, G. Prabakaran and R. Nandhakumar, Microchem. J., 2021, 169, 106590 CrossRef.
- Y. Yang, C.-Y. Gao, J. Liu and D. Dong, Anal. Methods, 2016, 8, 2863–2871 RSC.
- P. Roy, Coord. Chem. Rev., 2021, 427, 213562 CrossRef.
- P. Roy, Dalton Trans., 2021, 50, 7156–7165 RSC.
- A. Roy, M. Nandi and P. Roy, Trends Anal. Chem., 2021, 138, 116204 CrossRef CAS.
- A. Tarai, Y. Li, B. Liu, D. Zhang, J. Li, W. Yan, J. Zhang, J. Qu and Z. Yang, Coord. Chem. Rev., 2021, 445, 214070 CrossRef.
- X. Chen, T. Pradhan, F. Wang, J. S. Kim and J. Yoon, Chem. Rev., 2012, 112, 1910–1956 CrossRef PubMed.
- S. Paul, A. Manna and S. Goswami, Dalton Trans., 2015, 44, 11805–11810 RSC.
- S. Samanta, T. Ray, F. Haque and G. Das, J. Lumin., 2016, 171, 13–18 CrossRef.
- R. Alam, R. Bhowmick, A. S. M. Islam, A. Katarkar, K. Chaudhuri and M. Ali, New J. Chem., 2017, 41, 8359–8369 RSC.
- D. Das, R. Alam, A. Katarkar and M. Ali, Photochem. Photobiol. Sci., 2019, 18, 242–252 CrossRef CAS PubMed.
- A. Hazra and P. Roy, Anal. Chim. Acta, 2022, 1193, 339378 CrossRef CAS PubMed.
- J. J. Rodríguez-Mercado and M. A. Altamirano-Lozano, Drug Chem. Toxicol., 2012, 36, 369–383 CrossRef.
- Y. Zhang and M. Aly, Appl. Catal., A, 2022, 643, 118795 CrossRef CAS.
- C. Liu, J. Kang, Z.-Q. Huang, Y.-H. Song, Y.-S. Xiao, J. Song, J.-X. He, C.-R. Chang, H.-Q. Ge, Y. Wang, Z.-T. Liu and Z.-W. Liu, Nat. Commun., 2021, 12, 2305 CrossRef CAS PubMed.
- D. Dai, Z. Wang, C. Liu, X. Li, L. Zhang, Z. Xing, Z. Yang and P. Li, ACS Appl. Electron. Mater., 2019, 1, 2551–2559 CrossRef CAS.
- M. Stefanski, P. Głuchowski and W. Strek, Ceram. Int., 2020, 46, 29060–29066 CrossRef CAS.
- Y. Liu, S. He, D. Wu, X. Dong and W. Zhou, ACS Appl. Electron. Mater., 2022, 4, 643–650 CrossRef CAS.
- O. Nwakanma, S. Velumani and A. Morales-Acevedo, Mater. Today Energy, 2021, 20, 100617 CrossRef CAS.
- M. Hedayati, S. Olyaee and S. M. B. Ghorashi, J. Electronic Mater., 2020, 49, 1454 CrossRef.
- J. P. Maher, Annu. Rep. Prog. Chem., Sect. A: Inorg. Chem., 1999, 95, 45–56 RSC.
- V. Kumar, P. Kumar, S. Kumar, D. Singhal and R. Gupta, Inorg. Chem., 2019, 58, 10364–10376 CrossRef PubMed.
- C. Lim, M. An, H. Seo, J. Hyuk Huh, A. Pandith, A. Helal and H.-S. Kim, Sens. Actuators, B, 2017, 241, 789–799 CrossRef.
- K. Zhang, Y. Wu, W. Wang, B. Li, Y. Zhang and T. Zuo, Resour., Conserv. Recycl., 2015, 104, 276–290 CrossRef.
- D. Kim, A. W. H. Lee, J. I. Eastcott and B. D. Gates, ACS Appl. Nano Mater., 2018, 1, 2237–2248 CrossRef CAS.
- R. Suzuki, Y. Nishi, M. Matsubara, A. Muramatsu and K. Kanie, ACS Appl. Nano Mater., 2020, 3, 4870–4879 CrossRef.
- H.-F. Chang, P.-T. Yang, H.-W. Lin, K.-C. Yeh, M.-N. Chen and S.-L. Wang, Environ. Sci. Technol., 2020, 54, 14946–14954 CrossRef.
- C. F. Moyer, U. P. Kodavanti, J. K. Haseman, L. Costa and A. Nyska, Toxicol. Pathol., 2002, 30, 427–434 CrossRef PubMed.
-
R. Blain, in Handbook on the Toxicology of Metals, ed. G. F. Nordberg and M. Costa, Volume II: Specific Metals, Academic Press, 5th edn, 2022, pp. 795–806 Search PubMed.
- G. Genchi, A. Carocci, G. Lauria, M. S. Sinicropi and A. Catalano, Appl. Sci., 2021, 11, 8322 CrossRef.
- Q. Zhang and K. M.-C. Wong, Coord. Chem. Rev., 2020, 416, 213336 CrossRef.
- S. Dey, S. Sarkar, D. Maity and P. Roy, Sens. Actuators, B, 2017, 246, 518–534 CrossRef CAS.
- A. Roy, R. Mukherjee, B. Dam, S. Dam and P. Roy, New J. Chem., 2018, 42, 8415–8425 RSC.
- A. Roy, U. Shee, A. Mukherjee, S. K. Mandal and P. Roy, ACS Omega, 2019, 4, 6864–6875 CrossRef CAS.
- A. Roy, S. Das, S. Sacher, S. K. Mandal and P. Roy, Dalton Trans., 2019, 48, 17594–17604 RSC.
- A. Hazra, P. Ghosh and P. Roy, Spectrochim. Acta, Part A, 2022, 271, 120905 CrossRef CAS PubMed.
- A. M. Brouwer, Pure Appl. Chem., 2011, 83, 2213–2228 CrossRef CAS.
- J.-S. Wu, I.-C. Hwang, K. S. Kim and J. S. Kim, Org. Lett., 2007, 9, 907–910 CrossRef CAS PubMed.
- V. Thomsen, D. Schatzlein and D. Mercuro, Spectroscopy, 2003, 18, 112–114 CAS.
- R. Mehta, P. Kaur, D. Choudhury, K. Paul and V. Luxami, J. Photochem. Photobiol., A, 2019, 380, 111851 CrossRef CAS.
- A. Maji, S. Lohar, S. Pal and P. Chattopadhyay, J. Chem. Sci., 2017, 129, 1423–1430 CrossRef CAS.
- X. Wang and T. Li, Spectrochim. Acta, Part A, 2020, 229, 117951 CrossRef CAS PubMed.
- C. R. Lohani, J.-M. Kim, S.-Y. Chung, J. Yoon and K.-H. Lee, Analyst, 2010, 135, 2079–2084 RSC.
- D. Maity, A. Mukherjee, S. K. Mandal and P. Roy, J. Lumin., 2019, 210, 508–518 CrossRef CAS.
- X.-D. Jiang, H.-F. Yu, J.-L. Zhao, C.-L. Sun, Y. Xie and L.-J. Xiao, Chin. Chem. Lett., 2015, 26, 1241–1245 CrossRef.
- X. Leng, W. Xu, C. Qiao, X. Jia, Y. Long and B. Yang, RSC Adv., 2019, 9, 6027–6034 RSC.
- D. Jiang, X. Xue, M. Zhu, G. Zhang, Y. Wang, C. Feng, Z. Wang and H. Zhao, Ind. Eng. Chem. Res., 2019, 58, 18456–18467 CrossRef.
- C. Yu, L. Jian, Y. Ji and J. Zhang, RSC Adv., 2018, 8, 31106–31112 RSC.
- D. Li, C.-Y. Li, Y.-F. Li, Z. Li and F. Xu, Anal. Chim. Acta, 2016, 934, 218–225 CrossRef PubMed.
- S. Chemate and N. Sekar, Sens. Actuators, B, 2015, 220, 1196–1204 CrossRef CAS.
- S. Mondal, S. K. Manna, S. Pathak, A. Ghosh, P. Datta, D. Mandal and S. Mukhopadhyay, New J. Chem., 2020, 44, 7954–7961 RSC.
- G. Suna, E. Erdemir, L. Liv, S. Gunduz, T. Ozturk and E. Karakuş, Sens. Actuators, B, 2022, 360, 131658 CrossRef CAS.
- K. Huang, Y. Liu, P. Zhao, L. Liang, Q. Wang and D. Qin, Spectrochim. Acta, Part A, 2022, 282, 121688 CrossRef CAS PubMed.
- A. Rai, A. K. Singh, K. Tripathi, A. K. Sonkar, B. S. Chauhan, S. Srikrishna, T. D. James and L. Mishra, Sens. Actuators, B, 2018, 266, 95–105 CrossRef.
- L. Fan, J.-c. Qin, T.-r. Li, B.-d. Wang and Z.-y. Yang, Sens. Actuators, B, 2014, 203, 550–556 CrossRef.
- S. Mabhai, M. Dolai, S. Dey, A. Dhara, B. Das and A. Jana, New J. Chem., 2018, 42, 10191–10201 RSC.
- J. Zhang, Y. Zhou, W. Hu, L. Zhang, Q. Huang and T. Ma, Sens. Actuators, B, 2013, 183, 290–296 CrossRef.
|
This journal is © The Royal Society of Chemistry 2023 |
Click here to see how this site uses Cookies. View our privacy policy here.