Pd–Pt–Ru nanozyme with peroxidase-like activity for the detection of total antioxidant capacity†
Received
26th September 2022
, Accepted 29th November 2022
First published on 9th December 2022
Abstract
The design of highly active nanozymes and the establishment of ultra-sensitive bioassays remain a challenge. Therefore, it is necessary to synthesize highly active nanozymes. In this work, a Pd–Pt–Ru (PPR) nanozyme was prepared by atomic coating of the bimetallic nanozyme Pd–Pt. The steady-state kinetics showed that the PPR nanozyme had excellent peroxidase-like activity. Based on this concept, the as-prepared PPR nanozyme was applied to the detection of ascorbic acid (AA) and hydrogen peroxide (H2O2). The linear ranges for ascorbic acid and hydrogen peroxide were 2–12 μM and 5–40 mM, respectively. The limits of detection (LOD) are 1.13 μM and 2.79 mM, respectively. Ascorbic acid was used as a typical model to assay the total antioxidant capacity (TAC) of foods and several herbs. The Fructus Corni extract showed the highest reducing ability. The corresponding extracts were applied for the green synthesis of silver nanoparticles with a size of 167 nm. This study provides a method for the design of highly active nanozymes and the expansion of their applications.
1 Introduction
Total antioxidant capacity (TAC) is considered as an important index for evaluating the health of the body and a biomarker to measure the antioxidant potential of body fluids.1 The lack of TAC may lead to many diseases such as cancer, arteriosclerosis, diabetes, cardiovascular disease, and arthritis.2,3 Moreover, due to the imbalance between antioxidant capacity and reactive oxygen species, the cells and tissues are decomposed and metabolic function is affected. This imbalance is considered the main inducer of aging, and an increasing number of studies have shown that antioxidants are an important step in preventing aging.4 Recent research has shown that TAC is negatively correlated with chronic noncommunicable diseases (including cancer), which can guide the prognosis and nutritional management of these diseases.5 The reducing substances in the human body mainly come from vegetable, fruit or plant extracts. The consumption of vegetable, fruit, or plant extracts with high antioxidant content may reduce the risk of those diseases. Therefore, the detection of TAC in foods and drinks have clinical significance for disease prevention and prognosis management.
Generally, the oxidation free radical absorption capacity,6 ferric ion reduction,7 1,1-diphenyl-2-trinitrophenylhydrazine,8 and 2,2′-diazo methods9 are employed to assay TAC. Although the detection method represented by the DPPH method is relatively simple to operate, it is only suitable for the detection of phenol or phenol anions. The evaluation of TAC with antioxidants containing a sulfhydryl group remains limited.10 Therefore, the development of accurate methods for the evaluation of TAC is urgently required.
Nanozymes, first reported by Yan et al.,11 are nanomaterials with activity similar to that of enzymes. Compared with enzymes, the nanozymes show the advantages of lower cost, higher stability, longer durability, and easier production. During the past decade, nearly 900 types of nanozymes were reported to possess the ability of mimicking the activity of peroxidase, superoxide dismutase, catalase and oxidase. The peroxidase-like activity of nanozymes is widely used in the analysis of biomarkers.12 In recent years, the detection of antioxidants based on nanozymes is considered to be a promising method to replace conventional analysis due to the advantages of being inexpensive, easy to operate, and universal.13–16 Jia et al.17 developed a hydrogen peroxide and glucose biosensor platform using Co3O4 nanoparticles (NPs) to catalyze H2O2 as a color-emitting substrate. Pedone et al.18 used platinum nanoparticles, which can be combined with hydroxyl radical probes, allowing rapid detection of antioxidant activity in saliva at room temperature. However, the disadvantage of low catalytic activity of nanozymes hinders their practical applications.
The metallic nanozymes with multiple components have attracted considerable attention owing to their efficient catalytic activity in bioassays and therapeutic applications. The nanoparticles composed of Pd and Pt were reported to possess excellent peroxidase-like activity.19 Compared to the Pd nanoparticles, the composition of Pd and Cu endows the PdCu nanoparticles with excellent peroxidase-like activity.20 Generally, multimetallic nanozymes possess a relatively higher peroxidase-like activity than monometallic nanozymes due to the synergistic and electronic effects. However, limited by the uncertain catalytic mechanism, it is still difficult to obtain multicomponent nanozymes with high catalytic performance. Thanks to the scientists' previous work, great progress has been made in the design and synthesis of multimetallic nanozymes with high peroxidase-like activity in the last ten years.21 Xia et al.22 found that the coating of Ir on the surface of Pd nanocubes can improve the affinity of Pd nanocubes to the substrate (H2O2), leading to a significant enhancement in peroxidase-like activity. Liu et al.23 found that alloying Pt and Ru improved the pro-oxidation and anti-oxidation capacities. He et al.24 prepared a PtPdCu trimetallic nanozyme via co-reduction. The synergistic effect between the atoms changes the electronic structure of PdPt, leading to an enhanced affinity for H2O2 and an improvement in the peroxidase-like activity. The theoretical studies found that the adsorption energy between nanozymes and substrates should be suitable to maximize their peroxidase-like activities.25 The trimetallic composition can play a good role in regulating the adsorption energy between the nanozyme and substrates. Therefore, to obtain the high catalytic performance, it is desirable to explore multicomposition metallic nanozymes such as trimetallic nanozymes.
It is well known that dendritic structures can expose more active centers on the surface of the catalyst. To further enhance the catalytic performance of the nanozyme, Ru atoms were employed to coat the surface of Pd–Pt nanodendrites to form a trimetallic PPR nanozyme. The as-prepared PPR nanozyme shows a good peroxidase-like activity. As a proof of concept, the PPR nanozyme was used for the assay of TAC in foods and herbs.
2 Materials and methods
2.1 The synthesis of Pd–Pt nanoparticles
The Pd–Pt nanoparticles were prepared using a co-reduction method. A certain amount of K2PdBr4 (25.2 mg) and K2PtCl6 (48.6 mg) were weighed and dissolved in 5 mL deionized water. After dissolution, 5 mL of Pluronic F127 (10 mg mL−1) and ascorbic acid (0.1 mol L−1) solutions were added to the mixture and reacted for 24 h. After the reaction, the samples were washed with deionized water and placed in 10 mL ethylene glycol for later use.
2.2 The synthesis of the PPR nanozyme
The PPR nanozyme was prepared by atomic coating with Pd–Pt. During the synthesis of the PPR nanozyme, Pd–Pt nanoparticles (1 mL), AA (0.1 g), PVP (0.06 g), and KBr (0.05 g) were added to ethylene glycol solution. The reaction was carried out at 120 °C for 30 minutes and the reaction mixture was heated to 180 °C. Then, the RuCl3 (0.3 mg mL−1, 1.5 mL) solution was added to the cuvette. Finally, the PPR nanozyme was collected via centrifugation. The PPR nanozyme was dispersed in 1 mL of deionized water for later use.
2.3 The peroxidase-like activity study of the PPR nanozyme
For the TMB–H2O2–PPR system, TMB (100 μL) and H2O2 (400 μL) were subsequently added to 2.5 mL of acetic acid buffer solution (HAC–NaAC, pH = 4). Then a 10 μL suspension of PPR nanozyme was added to the mixture. Finally, the mixture was immediately scanned using a UV-vis spectrometer. In kinetic determination, Km reflects the affinity between the nanozyme and substrate, and Vmax is the maximum reaction speed at a saturated substrate concentration. The experiments were performed by changing the concentration of one substrate and maintaining a constant concentration of the other. When the substrate was TMB, the concentration of H2O2 was fixed. When the substrate was H2O2, the TMB concentration was constant. Km and Vmax were calculated according to formulae (1) and (2), fitting the curve to the Michaelis–Menten equation as follows: | v = [(Vmax × [S])/Km] + Vmax | (2) |
where [S] is the substrate concentration, v is the initial reaction rate, ΔA is the change in absorbance, Δt is the change in time, ε is the absorbance coefficient of oxidized TMB (39
000 M−1 cm−1), and l is the length of light through the colorimetric dish (cm).
3 Results and discussion
3.1 The morphology analysis of the PPR nanozyme
The Pd–Pt nanoparticles were characterized using transmission electron microscopy (TEM) (Fig. S1†). According to the TEM analysis (Fig. S1a†), the obtained Pd–Pt nanoparticles possess an urchin-like structure with uniform size and shape. High-resolution transmission electron microscopy (HRTEM) revealed that the surface of the Pd–Pt nanoparticles possessed a dendritic structure (Fig. S1b†). The elemental distribution of the Pd–Pt nanoparticles was characterized (Fig. S1c†). The elemental distribution shows that the Pd–Pt nanoparticles have a shell–core structure. Pd and Pt are present in the core and shell, respectively. The PPR nanozyme was characterized using TEM (Fig. 1). Similar to the Pd–Pt nanoparticles, the PPR nanozyme exhibits an urchin-like structure (Fig. 1a). As shown in the high-resolution TEM (HRTEM) images (Fig. 1b), the branch structure of PPR has many atomic steps. In the dark-field transmission electron microscopy (Fig. 1c) and the corresponding elemental distribution of the PPR nanozyme (Fig. 1d), the PPR nanozyme has a three-layer structure, with a nucleus of Pd, a mesosphere of Pt, and a surface of Ru.
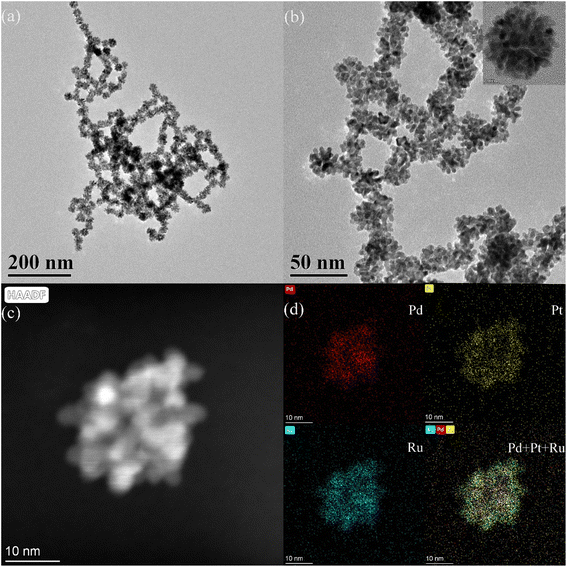 |
| Fig. 1 (a) The TEM pattern of the PPR nanozyme; (b) the HRTEM pattern of the PPR nanozyme; (c) dark field transmission electron microscopy of the PPR nanozyme; (d) the element distribution of the PPR nanozyme. | |
3.2 The valence analysis of the PPR nanozyme
Furthermore, the valence states of each element in Pd–Pt nanoparticles and the PPR nanozyme were analyzed using X-ray photoelectron spectroscopy (XPS) (Fig. 2). As shown in Fig. 2a, the Pd–Pt nanodendrites contain two elements, Pd and Pt. The PPR nanozyme contains three elements: Pd, Pt, and Ru. Elements O and C may be present because of exogenous factors. The composition of the PPR nanozyme characterized by XPS is consistent with the result of TEM-mapping. In the narrow spectrum, the binding energy of Pd and Pt shifts to the high field slightly. In this part, the narrow spectrum of Pt is analyzed. As shown in Fig. 2c, the 4f orbital of Pt splits into two peaks ranging from 68 eV to78 eV (Pt 4f5/2 and Pt 4f7/2). In the Pd–Pt nanoparticles, the characteristic peak of Pt(II) 4f5/2, Pt(II) 4f7/2, Pt(0) 4f5/2 and Pt(0) 4f7/2 are located at 74.76 eV, 71.28 eV, 74.13 eV and 70.76 eV, respectively.26 However, in the PPR nanozyme, the Pt(II) 4f5/2, Pt(II) 4f7/2, Pt(0) 4f5/2 and Pt(0) 4f7/2 peaks are located at 75.23 eV, 71.77 eV, 74.41 eV and 71.03 eV, respectively. The result shows that the coating of Ru gives rise to a slight migration of Pt binding energy to the high field. The migration demonstrates that some electrons are transferred from Pt to Ru atoms during the preparation of the PPR nanozyme, implying that the Ru atom binds to the Pd–Pt nanoparticles through chemical bonds other than absorption.27,28 What is more, in the PPR nanozyme, the characteristic peak of ruthenium (Ru 3p) is located near 462.67 eV and 485.20 eV, which is basically consistent with the 0 valence peak of Ru29 (Fig. 2d).
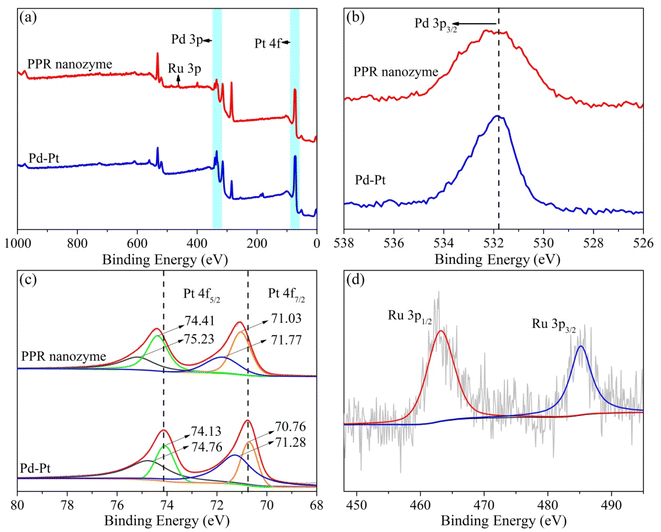 |
| Fig. 2 (a) The XPS full scan of the PPR nanozyme and Pd–Pt and (b–d) narrow spectrum analysis of Pd, Pt and Ru elements. | |
3.3 The peroxidase-like activity of the PPR nanozyme
The peroxidase-like activity of the PPR nanozyme was studied with TMB and H2O2 as the substrates. Oxidized TMB had an absorbance at 652 nm. With the same concentration of nanozyme Pd–Pt nanoparticles and PPR nanozyme (10 mg L−1), the higher the absorbance intensity, the higher the peroxidase-like activity. When the PPR nanozyme, H2O2 and TMB coexist, a strong absorption peak appears at a wavelength of 652 nm. The absorbance intensity of the sample (with TMB and H2O2) catalyzed by Pd–Pt nanoparticles is lower than that of the PPR nanozyme (Fig. 3a). As shown in Fig. 3b, at a reaction time of 60 s, the PPR nanozyme always shows higher peroxidase activity than Pd–Pt nanoparticles. These results show that the addition of Ru to Pd–Pt nanoparticles can improve catalytic activity.
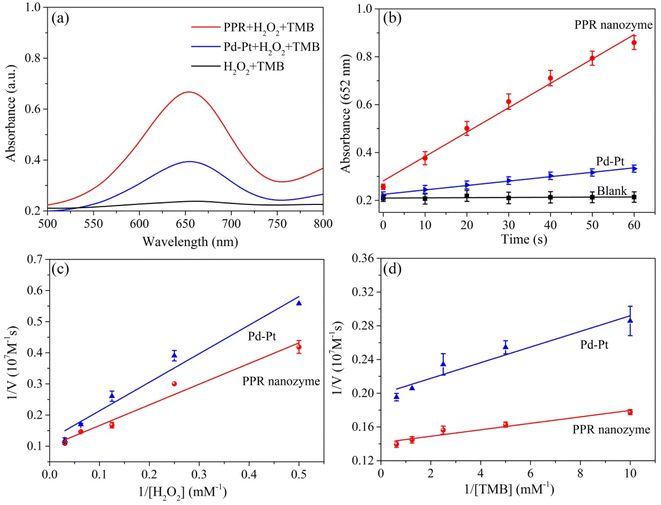 |
| Fig. 3 (a) The catalytic performance of different nanozymes; (b) time-dependent absorbance at 652 nm of reaction systems with varying nanozymes; the double-reciprocal plot in the presence of different concentrations of (c) H2O2 and (d) TMB. | |
To understand the catalytic mechanism, the steady-state kinetics of the PPR nanozyme were investigated. The corresponding double-reciprocal plots were obtained using the Michaelis–Menten reaction (Fig. 3c, d and S2†). It can be seen that as the concentration of H2O2 increased, Vmax gradually increased until stabilization. According to the double-reciprocal plot, the corresponding Km values of Pd–Pt nanoparticles, and the PPR nanozyme for H2O2 are calculated to be 7.53 mM and 6.64 mM, respectively. As shown in Table S1,† compared with typical monometallic or multimetallic nanozymes, PPR nanozymes possess a relatively high affinity for H2O2. The results show that the PPR nanozyme has a relatively high affinity for H2O2 compared to Pd–Pt nanoparticles. The Km values of Pd–Pt nanoparticles and the PPR nanozyme for TMB were detected and calculated to be 0.047 mM and 0.026 mM, respectively. The results show that the Km value of TMB is smaller than that of H2O2, indicating that PPR nanozymes are more likely to adsorb TMB.
High peroxidase-like activity of nanozymes is usually related to ROS generation of reactive oxygen species. The higher the reactive oxygen produced, the higher the peroxidase-like activity.30 Thus, terephthalic acid (TA) was used as a fluorescent probe to detect hydroxyl radicals in the nanozyme and H2O2 systems. As shown in Fig. 4a, the hydroxyl radical content produced by H2O2 and the PPR nanozyme is higher than that of H2O2 and Pd–Pt. In addition, 5,5-dimethyl-1-pyrroline-N-oxide (DMPO) was used as a capture agent to trap the hydroxyl radicals. The product of DMPO and ·OH generates a characterized peak with a ratio of 1
:
2
:
2
:
1, ranging from 3460 to 3560 (G). The characterized peak was recorded using an electron spin response (ESR) detector. The intensity of the characterized peak reflects the hydroxyl radical content produced by H2O2 and the nanozyme. As shown in Fig. 4b, the number of hydroxyl radicals generated by H2O2 and the PPR nanozyme is greater than those generated by H2O2 and Pd–Pt nanoparticles. The results of fluorescence analysis and ESR show that the content of hydroxyl radicals produced by the PPR nanozyme is higher than that of Pd–Pt nanoparticles, which leads to a higher peroxidase-like activity.
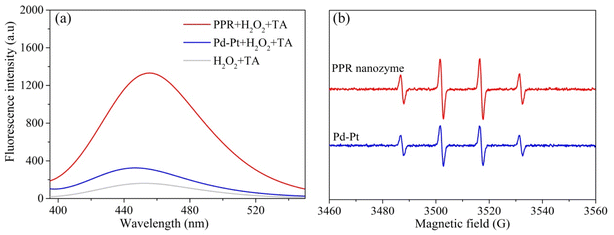 |
| Fig. 4 (a) The reaction between terephthalic acid (TA) and ·OH radicals generated by Pd–Pt and the PPR nanozyme; the concentrations of terephthalic acid and H2O2 were 0.5 mM and 62.5 mM respectively; (b) the ESR spectra of Pd–Pt and the PPR nanozyme in buffer solution (pH = 4); DMPO was used in the ESR analysis. | |
The activity of nanozymes depends on the pH and temperature. Thus, the catalytic performance of the PPR nanozyme at different pH values and temperatures was investigated. As shown in Fig. S3a,† with an increase in the pH value, the catalytic activity of the nanozyme changed. As the pH increases (3.0–9.0), the activity shows a tendency to increase and then decrease. The catalytic activity reaches a maximum value at pH = 4.0. As far as we know, most of the reported nanozymes possess the optimal catalytic performance in the pH range from 3 to 5.31 In fact, under acidic conditions, H2O2 on the surface of the nanoenzyme is more likely to be cleaved into ·OH and participate in the oxidation of TMB. Therefore, a buffer of pH 4.0 was chosen for the reaction. The results show that the nanozyme possessed peroxidase-like activity under acidic conditions (pH = 4.0). From the experimental results of the temperature investigation (Fig. S3b†), it can be seen that the activity of the nanozyme basically remains unchanged as the temperature changes from 25 °C to 100 °C. Nanozymes have excellent thermal stability and potential to replace traditional nanozymes.
3.4 The application of the PPR nanozyme for the assay of TAC
PPR nanozymes can catalyze the oxidation of TMB by H2O2. With the increase of the concentration of H2O2, the color of blue products gradually deepened. Therefore, the PPR nanozyme was used to detect H2O2. As shown in Fig. 5a, with an increase in the H2O2 concentration, the absorbance of the oxidation products at 652 nm gradually increased. The linear interval for the detection of H2O2 is 5–40 mM with an LOD (limit of detection) of 2.79 mM (Fig. 5b). Limited by the peroxidase-like activity of the PPR nanozyme, the sensor performance towards H2O2 stays at the level of millimoles per liter. It is not difficult to find that the LOD towards H2O2 is consistent with the Km (6.64 mM) of the PPR nanozyme to H2O2.
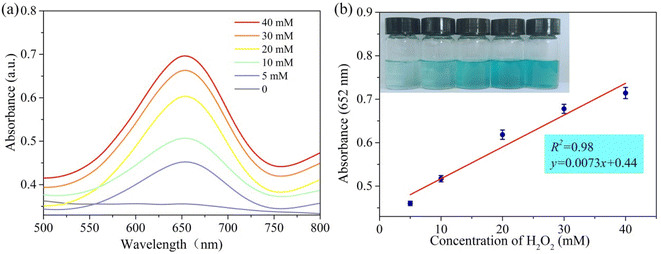 |
| Fig. 5 (a) The absorption spectra of reaction systems in different concentrations of H2O2 at 652 nm; (b) the relationship between absorbance and concentration of H2O2 at 652 nm. | |
The PPR nanozyme catalyzes the oxidation of TMB to H2O2. However, oxidized TMB is reduced by reducing substances (e.g., AA), leading to the fading of the blue color. Therefore, the PPR nanozyme was used for AA detection in this study. As shown in Fig. 6a, when the AA concentration gradually increased, the absorbance of oxidized TMB gradually decreased. The concentration of AA shows a linear relationship with the absorbance intensity at 652 nm (Fig. 6b). The linear range for the detection of AA is 0–12 μM, with an LOD of 1.13 μM, which is comparable to that of other nanocomposite based colorimetric detection of AA (Table S2†). The Na+, K+, Ca2+, Cu2+, Mg2+etc. were selected to evaluate the interference and selectivity of the method developed in this study. The result in Fig. S4† shows that the methods used for the detection of AA possess excellent interference and selectivity. Finally, the method developed in this study was employed to evaluate the TAC of different samples, such as drinks, foods, and extracts of herbs. The result shows that the TAC content of the samples decreased in the order: orange > ice tea > green tea > lemon > tomato (Fig. 6c). The TAC of beverages is higher than that of fresh vegetables, which may arise from the use of concentrated juice and some antioxidant additives in the production of beverages. As shown in Fig. 6d, the result demonstrates that the TAC is higher in Fructus Corni than in Radix Paeoniae Alba, Rhizoma Dioscoreae Bulbiferae, or Folium Eriobotryae. As we know, the extracts of the herbs are always used for the green synthesis of silver nanoparticles (AgNPs). Then, the total antioxidant capacity of Fructus Corni was tested at different temperatures (40–100 °C). In general, the high temperature can improve the release of constituents from plant materials to the solvent.32 Thus, as shown in Fig. 7b, the raised temperature leads to the release improvement of TAC in Fructus Corni. To minimize energy consumption, the extracts from Fructus Corni at 40 °C were further used for the green synthesis of AgNPs (Fig. 7a). The as-prepared AgNPs are nearly spherical with an average size of 167 nm (Fig. 7c).
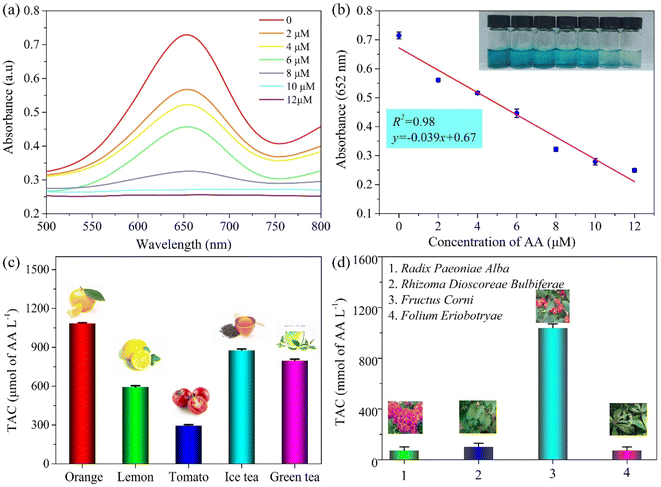 |
| Fig. 6 (a) The absorbance spectra of reaction systems in different concentrations of AA at 652 nm; (b) the linear curve of AA detection based on the PPR nanozyme; (c) the TAC content in foods and drinks; (d) the TAC content in herbs. | |
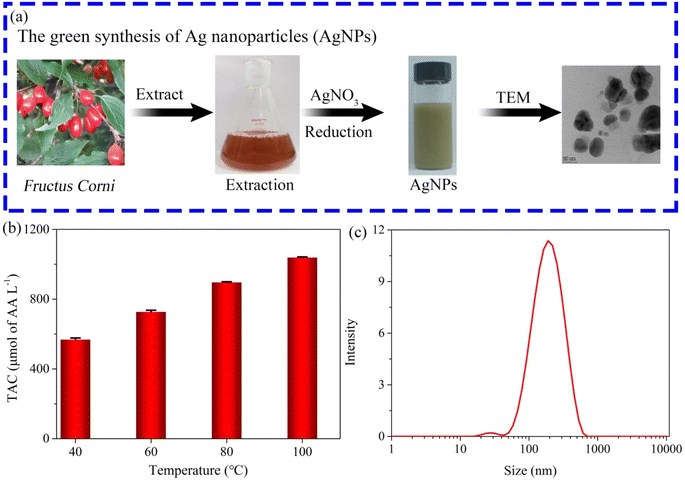 |
| Fig. 7 (a) The process of green synthesis of AgNPs; (b) the TAC content in Fructus Corni at different temperatures; (c) the size distribution of the as-synthesized AgNPs. | |
4 Conclusion
In summary, a PPR nanozyme with excellent peroxidase-like activity was synthesized in this study. The as-prepared PPR nanozymes were characterized as nanodendrites with sandwich structures using TEM, HRTEM, and TEM mapping. According to XPS analysis, the Ru coat binds to the Pd–Pt nanoparticles through chemical bonds rather than adsorption. The PPR nanozyme showed higher peroxidase-like activity than the Pd–Pt nanoparticles, according to the enzyme kinetic data. The high activity is due to the high content of ·OH, which is catalyzed by the PPR nanozyme in the presence of H2O2. The PPR nanozyme was finally employed to assay the TAC of topical foods and extracts of herbs. The TAC content in orange was the highest compared to that in other foods such as ice tea, green tea, lemon, and tomato. The extract of Fructus Corni possessed the highest TAC content compared to other herbs such as Radix Paeoniae Alba, Rhizoma Dioscoreae Bulbiferae, and Folium Eriobotryae. Fructus Corni extracts were successfully applied for the green synthesis of silver nanoparticles (average size of 167 nm). The study not only provides a new idea for the design of high peroxidase-like activity nanozymes, but also provides a method for the rapid detection of the TAC in foods and drinks. This work is of important theoretical and practical value.
Conflicts of interest
The authors declare that they have no affiliations with or involvement in any organization or entity with any financial interest in the subject matter or materials discussed in this manuscript.
Acknowledgements
This work was financially supported by the National Key Research and Development Program of China (2017YFC1703102); the Scientific Research Project of the Education Department of Hunan Province (20C1622 and 22A0318); the Natural Science Foundation of Hunan Province (2020JJ4521 and 2021JJ70115); the Hengyang Science and Technology Planning Project (2020hcjz6717); the Postgraduate Scientific Research Innovation Project of Hunan Province (QL20210230).
References
- E. Niki, Assessment of antioxidant capacity in vitro and in vivo, Free Radicals Biol. Med., 2010, 49, 503–515 CrossRef PubMed.
- K. Hagner and H. Brath, A global view on the development of non-communicable diseases, Prev. Med., 2012, 54, 38–41 CrossRef.
- G. Y Liou and P. Storz, Reactive oxygen species in cancer, Free Radical Res., 2010, 44, 479–496 CrossRef.
- J. Lee, N. Koo and D. B. Min, Reactive oxygen species, aging, and antioxidative nutraceuticals, Compr. Rev. Food Sci. Food Saf., 2004, 3, 21–33 CrossRef.
- Ld. O. Possa, J. V. Hinkelmann, C. Araújo dos Santos, C. A de Oliveira, B. S Faria and H. H. M. Hermsdorff, Association of dietary total antioxidant capacity with anthropometric indicators, c-reactive protein, and clinical outcomes in hospitalized oncologic patients, Nutrition, 202, 90, 111359 CrossRef.
- B. Ou, M. Hampsch-Woodill and R. L. Prior, Development and validation of an improved oxygen radical absorbance capacity assay using fluorescein as the fluorescent probe, J. Agric. Food Chem., 2001, 49, 4619–4626 CrossRef.
- M. Nardini and I. Garaguso, Characterization of bioactive compounds and antioxidant activity of fruit beers, Food Chem., 2020, 305, 125437 CrossRef PubMed.
- W. Brand-Williams, M. E. Cuvelier and C. Berset, Use of a free radical method to evaluate antioxidant activity, Food Sci. Technol., 1995, 28, 25–30 CAS.
- R. Re, N. Pellegrini, A. Proteggente, A. Pannala, M. Yang and C. Rice-Evans, Antioxidant activity applying an improved ABTS radical cation decolorization assay, Free Radicals Biol. Med., 1999, 26, 1231–1237 CrossRef PubMed.
- M. C. Foti, C. Daquino and C. Geraci, Electron-Transfer reaction of cinnamic acids and their methyl esters with the DPPH• radical in alcoholic solutions, J. Org. Chem., 2004, 69, 2309–2314 CrossRef PubMed.
- L. Z. Gao, J. Zhuang, L. Nie, J. B. Zhang, Y. Zhang, N. Gu, T. H. Wang, J. Feng, D. L. Yang, S. Perrett and X. Y. Yan, Intrinsic peroxidase-like activity of ferromagnetic nanoparticles, Nat. Nanotechnol., 2007, 2, 577–583 CrossRef.
- B. Liu, Y. Wang, Y. Chen, L. Guo and G. Wei, Biomimetic two-dimensional nanozymes: Synthesis, hybridization, functional tailoring, and biosensor applications, J. Mater. Chem. B, 2020, 8, 10065–10086 RSC.
- K. Nitinaivinij, T. Parnklang, C. Thammacharoen, S. Ekgasit and K. Wongravee, Colorimetric determination of hydrogen peroxide by morphological decomposition of silver nanoprisms coupled with chromaticity analysis, Anal. Methods, 2014, 24, 9816–9824 RSC.
- S. Lorscheidt and A. Lamprecht, Safety assessment of nanoparticles for drug delivery by means of classic in vitro assays and beyond, Expert Opin. Drug Delivery, 2016, 13, 1545–1558 CrossRef CAS PubMed.
- B. Gonca, H. Akhtar and A. Silvana, Portable nanoparticle-based sensors for food safety assessment, Sensors, 2015, 12, 30736–30758 Search PubMed.
- D. Zhu, B. Liu and G. Wei, Two-Dimensional material-based colorimetric biosensors: a review, Biosensors, 2021, 11, 259 CrossRef CAS.
- H. M. Jia, D. F. Yang, X. N. Han, J. H. Cai, H. Y. Liu and W. W. He, Peroxidase-like activity of the Co3O4 nanoparticles used for biodetection and evaluation of antioxidant behavior, Nanoscale, 2016, 8, 5938–5945 RSC.
- D. Pedone, M. Moglianetti, M. Lettieri, G. Marrazza and P. P. Pompa, Platinum nanozyme-enabled colorimetric determination of total antioxidant level in saliva, Anal. Chem., 2020, 92, 8660–8664 CrossRef CAS.
- E. Y. Kwon, X. F. Ruan, L. M. Wang, Y. H. Lin and D. Du, Mesoporous Pd@Pt nanoparticle-linked
immunosorbent assay for detection of atrazine, Anal. Chim. Acta, 2020, 1116, 36–44 CrossRef CAS.
- Y. He, X. Niu, L. Li, X. Li, W. Zhang and H. Zhao, Microwave-Assisted fabrication of bimetallic PdCu nanocorals with enhanced peroxidase-like activity and efficiency for thiocyanate sensing, ACS Appl. Nano Mater., 2018, 1, 2397–2405 CrossRef CAS.
- Z. R. Wang, R. F. Zhang, X. Y. Yan and K. L. Fan, Structure and activity of nanozymes: Inspirations for de novo design of nanozymes, Mater. Today, 2020, 41, 81–119 CrossRef CAS.
- X. H. Xia, J. T. Zhang, N. Lu, M. J. Kim, K. S. Ghale, Y. Xu, E. McKenzie, J. B. Liu and H. H. Ye, Pd–Ir core–shell nanocubes: a type of highly efficient and versatile peroxidase mimic, ACS Nano, 2015, 9, 9994–10004 CrossRef.
- C. Liu, Y. Y. Yan, X. W. Zhang, Y. Y. Mao, X. Q. Ren, C. Y. Hu, W. W. He and J. J. Yin, Regulating the Pro- and Anti-Oxidant Capability of Bimetallic Nanozymes for Detection of Fe2+ and Protection of Monascus pigments, Nanoscale, 2020, 12, 3068–3075 RSC.
- Y. Y. Mao, F. M. Jia, T. T. Jing, T. T. Li, H. M. Jia and W. W. He, Enhanced multiple enzymelike activity of PtPdCu trimetallic nanostructures for detection of Fe2+ and evaluation of antioxidant capability, ACS Sustainable Chem. Eng., 2020, 9, 569–579 CrossRef.
- X. Shen, Z. Wang, X. Gao and Y. Zhao, Density functional theory-based method to predict the activities of nanomaterials as peroxidase mimics, ACS Catal., 2020, 10, 12657–12665 CrossRef.
- Z. X. Sun, X. F. Li, S. Guo, H. Q. Wang and Z. B. Wu, One-step synthesis of Cl−-doped Pt (IV)/Bi2WO6 with advanced visible-light photocatalytic activity for toluene degradation in air, J. Colloid Interface Sci., 2013, 412, 31–38 CrossRef PubMed.
- J. J. Liu, S. H. Zou, L. P. Xiao and J. Fan, Well-dispersed bimetallic nanoparticles confined in mesoporous metal oxides and their optimized catalytic activity for nitrobenzene hydrogenation, Catal.: Sci. Technol., 2014, 4, 441–446 RSC.
- H. Wang, S. Chen, C. Wang, K. Zhang, D. Liu and Y. A. Haleem, Role of Ru oxidation degree for catalytic activity in bimetallic Pt/Ru nanoparticles, J. Phys. Chem. C, 2016, 120, 6569–6576 CrossRef.
- A. Lewera, W. P. Zhou, C. Vericat, J. H. Chung, R. Haasch, A. Wieckowski and P. S. Bagus, XPS and reactivity study of bimetallic nanoparticles containing Ru and Pt supported on a gold disk, Electrochim. Acta, 2006, 19, 3950–3956 CrossRef.
- X. Y. Ren, D. X. Chen, Y. Wang, H. F. Li, Y. B. Zhang, H. Y. Chen, X. Li and M. F. Huo, Nanozymes-recent development and biomedical applications, J. Nanobiotechnol., 2022, 92 CrossRef PubMed.
- D. Jiang, D. Ni, Z. T. Rosenkrans, P. Huang, X. Yan and W. Cai, Nanozyme: new horizons for responsive biomedical applications, Chem. Soc. Rev., 2019, 48, 3683–3704 RSC.
- X. Peng, M. H. Duan, X. H. Yao, Y. H. Zhang, C. J. Zhao, Y. G. Zu and Y. J. Fu, Green extraction of five target phenolic acids from Lonicerae japonicae Flos with deep eutectic solvent, Sep. Purif. Technol., 2016, 157, 249–257 CrossRef.
|
This journal is © The Royal Society of Chemistry 2023 |