DOI:
10.1039/D4RA02704C
(Review Article)
RSC Adv., 2024,
14, 20032-20047
Beyond graphene: exploring the potential of MXene anodes for enhanced lithium–sulfur battery performance
Received
11th April 2024
, Accepted 4th June 2024
First published on 21st June 2024
Abstract
The high theoretical energy density of Li–S batteries makes them a viable option for energy storage systems in the near future. Considering the challenges associated with sulfur's dielectric properties and the synthesis of soluble polysulfides during Li–S battery cycling, the exceptional ability of MXene materials to overcome these challenges has led to a recent surge in the usage of these materials as anodes in Li–S batteries. The methods for enhancing anode performance in Li–S batteries via the use of MXene interfaces are thoroughly investigated in this study. This study covers a wide range of techniques such as surface functionalization, heteroatom doping, and composite structure design for enhancing MXene interfaces. Examining challenges and potential downsides of MXene-based anodes offers a thorough overview of the current state of the field. This review encompasses recent findings and provides a thorough analysis of advantages and disadvantages of adding MXene interfaces to improve anode performance to assist researchers and practitioners working in this field. This review contributes significantly to ongoing efforts for the development of reliable and effective energy storage solutions for the future.
1. Introduction
MXenes are two-dimensional (2D) transition metal compounds that may transform lithium–sulfur (Li–S) batteries via carbides, nitrides, and carbonitrides.1 These innovative materials have attracted attention due to their unique structure and excellent electrochemical performance, which may help to address Li–S battery issues.2 Their practical implementation has been restricted by the limited electrical conductivity of sulphur and the breakdown of intermediate polysulfides caused by cycling. These 2D materials make good anodes because of their high electrical conductivity.3 The structured layers provide sufficient space for sulphur incorporation, thus effectively addressing the problem of polysulfide dissolution. MXenes are well known for their remarkable mechanical stability and strength, which prolong the life of Li–S batteries.4 The structural flexibility of MXenes allows for the accommodation of variations in Li–S battery volume during charge and discharge.5 Cycle stability and battery longevity are enhanced by this feature. As Li–S battery anodes, MXenes represent a significant advancement in energy storage.6 Because of its excellent electrical conductivity, sulphur retention, and structural stability, this method may increase energy storage by surpassing limitations of conventional Li–S batteries. With the development of battery technology, scientists may soon have workable and durable solutions.7
Lithium–sulfur batteries have recently been the focus of research due to their potential to revolutionise energy storage.8–10 Li–S batteries are anticipated to outperform and function better than Li-ion batteries due to their high energy density.11,12 The widespread use of Li–S batteries has been restricted by many challenges.13 Scientists are employing MXenes to address these problems and optimise the performance of Li–S batteries. Global concerns over the need for effective energy storage systems have been raised by the rapid growth of renewable energy sources, shift to electric cars, and need for dependable power storage solutions.2,14,15 Li–S batteries are a unique energy storage technology that might address several energy storage issues. Li–S batteries are more desirable because of several advantages.13 Consequently, they may have a greater energy density than lithium-ion batteries, enabling the development of more durable and long-lasting energy storage devices.16 Sulphur, an abundant and non-toxic cathode component, makes Li–S batteries more environmentally friendly. They are sustainable and aid the world's transition to greener energy.17 Sulphur outperforms lithium-ion batteries in terms of energy density. However, implementing the principle has been difficult.18 Sulfur's intrinsic insulating qualities prevent electrons from moving freely, which lowers battery performance. Sulphur may produce soluble polysulfides that can migrate away from the cathode as a result of the intricate electrochemical reactions that occur during cycling, which reduce capacity and shorten cycle life.19
MXenes are a class of multilayer, ternary carbides and nitrides that were initially identified in 2011 by selectively etching a MAX phase. By eliminating the “A” layer, which is usually composed of aluminium, a two-dimensional structure was created.20 MXenes have excellent mechanical stability, adjustable surface chemistry, and electrical conductivity. These qualities stimulate the attention of researchers in the energy storage field and other fields.21 The strong conductivity of these materials overcomes the poor conductivity of sulphur, making them perfect anodes for Li–S batteries.22 MXenes improve battery electrochemical performance by accelerating electron transport while acting as anodes. According to the study, MXenes slow down the dissolution of polysulfide in Li–S batteries.23 By selectively interacting with sulphur species, MXenes may be surface modified to enhance polysulfide retention and trapping. The performance and efficiency of Li–S batteries are increased by this tailored surface chemistry.24 The various properties of MXene are shown in Fig. 1.
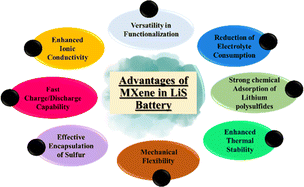 |
| Fig. 1 Imperative properties of MXene. | |
Despite volume changes, MXenes show exceptional structural stability when Li–S batteries are charged and drained. This property enables enhanced cycle stability and longer lifespans for Li–S batteries that use MXenes as the anode material.25 Understanding MXenes in Li–S batteries encourages environmental responsibility and sustainability. Li–S batteries are more affordable and have a higher energy density than lithium-ion batteries when it comes to renewable energy storage, electric vehicle power, and portable device usage.26 MXenes are revolutionizing energy storage, and additional research may improve efficiency and sustainability.27 For lithium-sulfur batteries, a stable lithium metal anode has polysulfide encapsulation: a layered MXene-protected lithium metal anode is an efficient polysulfide blocker.28 It has been reported that Ti2C-based MXenes are effective in enhancing the electrochemical performance of lithium–sulfur batteries. The introduction of the surface chemistry characteristics of MXenes enhances the performance, whereas concerns related to the favourable functionalized surface during the charge and discharge process exist.29 A simple approach for creating 3D S-CNT@MXene cages is further proposed to address challenges in the LiS batteries. Three-dimensional cages with conductive networks in contact with each other can increase sulfur active site accessibility, reduce electrode resistance, and promote reaction rates.30 Tailoring MXene (2D-Ti3C2)-derived TiN with well-defined facets yields an efficient bidirectional electro catalyst for high-performance Li–S batteries.26 Chemical etching is employed to obtain the delaminated Mo2CTx MXene nano sheets that are utilized as sulfur hosts. The amplified shuttle effect and comparatively slow recharge ability of polysulfides are major drawbacks in the practical implementation of lithium–sulfur batteries.31 An MXene/MoS2/SnS@C flower structure used as the functional intercalation of Li–S batteries was designed to enhance the synergistic electrocatalytic processes involved in sulfur conversion. The MXene framework forms a three-dimensional conductive backbone that constrains the morphology of the polysulfides and promotes charge transfer.32 A sample of multi-hetero-structured MXene/NiS2/Co3S4 with rich S-vacancies was fabricated using a hydrothermal and high-temperature annealing process. The MXene sheet not only serves as a mechanical barrier but also enhances the conductivity and adsorption capability of the catalyst NiS2/Co3S4 double active centre, accelerating the conversion of LiPSs.33
This article reviews the latest research on MXene anodes in lithium–sulfur batteries. This study aims to explain the unique features of MXenes that make them suitable anode materials. The features include mechanical stability, chemical compatibility with lithium–sulfur systems, and electrical conductivity. This study critically analyses the volume growth, cycle stability, and potential unfavorable reactions of MXenes that might influence the performance of lithium–sulfur batteries. This article summarizes MXene-based anodes in lithium–sulfur battery systems and discusses future approaches and applications.
2. Lithium–sulfur battery technology
Although cheaper than lithium-ion batteries, lithium–sulfur (Li–S) batteries have gained popularity as energy storage alternatives due to their high theoretical energy density. If you want more energy and less pollution, Li–S batteries are chosen.28 Sulphur, unlike lithium cobalt oxide, is a potential lithium-ion cathode. Removing heavy metals from cathodes reduces health risks, battery manufacturing and disposal environmental effects. Research and development are focused on solving Li–S battery issues and improving their capabilities.34 These innovative batteries combine sulphur and MXenes to store energy efficiently and sustainably via electrochemical reactions.35 Lithium and sulphur react during a Li–S battery's discharge cycle to produce electricity.36 Lithium anodes, generally Li metal or Li-ion intercalation materials, initiate the process, while sulphur functions as the cathode. Li+ ions go from the anode to the cathode via the electrolyte, and this process is reversed during charging. The motion of lithium ions generates battery output.37 At the cathode, intermediate lithium polysulfides (Li2Sx), where x is the number of sulphur atoms in the polysulfide, are created when lithium ions react with sulphur (S8).38 This implies that Li-ion batteries hold energy by lowering Li-ions through a reversible process. During this process, electrons are liberated, producing an electrical current with various uses.39 The electrons produced by sulphur reduction processes may provide an electrical current that can power electronics or operate machines by connecting them to an external circuit.40 The battery's capacity to produce electricity is based on this electron flux. The basic assembly of the Li–S battery is shown in Fig. 2.
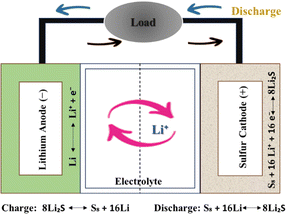 |
| Fig. 2 Basic assembly of lithium sulfur battery. | |
One must reverse the electrochemical events that occur during discharge to recharge a Li–S battery. The charging process's basic operation involves many phases.41 When lithium polysulfides (Li2Sx) receive external electrical energy, they undergo oxidation reactions. Lithium ions are released into the electrolyte, and sulphur molecules (S8) are formed as a consequence of this process.42 The lithium anode's electrolyte concurrently transports lithium ions from the cathode to the anode. These lithium ions are absorbed by the lithium metal or lithium-ion intercalation anode.43 Electrons from the external circuit enter the anode during charging and mix with lithium ions. This procedure returns the lithium ions to their initial condition for the next discharge cycle.44 The anode material in Li–S batteries is made of MXene compounds. In the process of charging, electrons from the external circuit enter the anode and mix with lithium ions. In addition to improving the battery's performance during the next discharge cycle, this procedure fixes typical problems with Li–S batteries by restoring the lithium ions to their initial conditions.45 Sulphur's weak electrical conductivity is a concern with Li–S batteries, whereas the great conductivity of MXenes addresses this issue. Additionally, MXene materials efficiently immobilise Li2Sx produced during discharge and charge operations.46 Even with volume differences from cycling, MXenes are mechanically stable. Due to their mechanical resilience, Li–S batteries have better cycling stability and longevity.47 Li–S batteries with MXene anodes work because of the synergy between lithium ions, sulphur cathodes, and the particular characteristics of MXenes. MXenes improve the performance and solve problems in typical Li–S battery technology.48 Research and development in this sector are constantly changing the operating principles and practical uses of these cutting-edge energy storage technologies.49
3. MXene material properties and synthesis
3.1 Overview of MXene materials
Professor Yury Gogotsi and Professor Michel W. Barsoum discovered MXene, a new 2D material, in 2011 with their colleagues at Drexel University.50 The basic formula of MXene can be expressed as Mn+1XnTz or M1.33XTz (z = 1, 2, 3), where TX shows terminal functional groups, including oxygen, hydroxide, and fluorine; M represents early transition metals, such as Sc and Ti; and X represents carbon or nitrogen elements.51 Generally, MAX-phase substances and their correspondents are used to produce MXene.52 Max phases are layered ternary carbides and nitrides53 with a general formula (Mn+1AXn),54 where ‘M’ represents the transition metal, ‘A’ represents the group IV–V element, and ‘X’ represents either carbon or nitrogen.55 The MXene family did not demonstrate its existence before 2011 but has significantly grown in prominence from the chemistry and application viewpoint,56 and scientific reporting affiliated with MXene nearly doubles each year.57 In recent years, numerous two-dimensional substances have been fabricated, including phosphorene, silicon germanane, hexagonal boron nitride, and transition metal dichalcogenides.58 MXene composed of different metals is shown in Fig. 3.
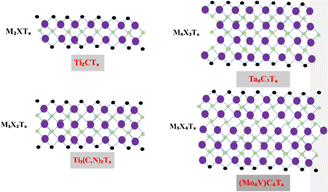 |
| Fig. 3 Two-dimensional structures of MXene with different transition metals. | |
The majority of already known 2D materials until 2011 were poor or not conductors of electricity and exhibited low carrier concentrations.59 MXene materials have numerous fascinating physico-chemical properties,59 such as excellent electrical conductivity (4.52 × 10−4 S m−1);60 a tunable band gap;61 large negative zeta potential;62 good flexibility;63 and chemical,64 constructional,65 optical and magnetic properties, along abundant active catalytic sites.66 MXene materials also have good mechanical properties, and their hardness and strength moderately improve by increasing ‘n’.67 The most abundantly studied MXene is Ti3C2Tx, which exhibits superb versatility, processing ability, and design ability due to its unique physical and chemical properties.68 MXenes possess numerous features, such as high surface area, hydrophilicity and a lesser diffusion barrier, due to the presence of a large number of functional groups.69 The electronic conductivity of MXene materials is affected by the position of metals and functionalities, such as O, F, and OH, present on the surface of MXenes.70
3.2 Synthesis techniques
In general, MXenes can be fabricated using either bottom-up or top-down methods.65 The prevailing procedure in top-down involves acid etching, whereas chemical vapor deposition is the key approach in the bottom-up method,71 which can manufacture good-quality films on substrate.72 Electro catalytic properties were affected when different methodologies modified the structure or surface termination of MXene materials.73 The top-down approach is the famous synthetic method, starting from the Max phase.65 MXene materials are acquired from their precursor MAX phases by chemical exfoliation.51 In the beginning, by mixing the elemental powders of M, A, and X in specific atomic ratios at high temperatures, the formation of an MAX phase and sample densification occurs after hot or cold pressing.74 After the fabrication of the MAX phase, the initial step involves the etching of the 3D Max phase using a potent etchant, typically hydrofluoric acid. M–A bonds are weaker than M–X bonds, allowing for the selective etching of M–A bonds.65 The various methods for the synthesis of the MXene are presented in Fig. 4.
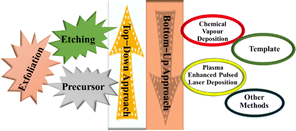 |
| Fig. 4 Different methods for the synthesis of MXene. | |
Etchants are necessary to disrupt the robust chemical bonds that exist between elements A and M in the Max phase.74 Several techniques utilized for the synthesis of MXene have been reported, namely HF etching, in situ etching, molten fluoride etching, non-fluorine etching, and electrochemical etching.75 In 2021, Zamhuri and colleagues pioneered the synthesis of the initial MXene by immersing Ti3AlC2 powder in 50% concentrated hydrofluoric acid (HF) for 2 hours at room temperature. This process led to the comprehensive dissociation of the Max phase.65 Three lattice structures of MXenes, consisting of 3, 5 and 7 atomic layers, represented as M2X, M3X2 and M4X3, respectively, can be derived from the Max phase.70 Confirmation of the conversion from the MAX phase to MXene can be achieved through X-ray diffraction (XRD) and energy-dispersion spectroscopy (EDS).74 In the fabrication of MXene, the method of etching with HF is presently employed, but it is essential to emphasize that larger concentrations of HF can be dangerous75 as corrosive agents, making their handling and disposal harmful.76 Verger et al. (2019) explored the feasibility of using a mixture of hydrochloric acid (HCl) and lithium fluoride (LiF) for etching Max phase materials. This approach generates small amounts of in situ HF.77 Ammonium hydrogen bifluoride (NH4HF2) and ammonium fluoride are commonly employed in the synthesis of Ti3C2 from Ti3AlC2.78 An alternative technique involves combining Ti4AlN3 powder with a specific mixture of molten fluoride salts, such as NaF, KF, and LiF. For half an hour, the mixture is blended at 550 °C.65 A recent work by Li et al. (2021) presented a method for creating a Zn-based MAX phase with a chlorine-terminated, fluorine-free surface. MXene accomplished this by reacting the Lewis acidic molten salt with the MAX phase via a replacement reaction mechanism.79 Electrochemical exfoliation has been extensively used for 2D materials, such as graphene and phosphorene. In a recent study, Chaturvedi et al. (2023) showed how to synthesise Ti2CTx in a three-electrode cell by electrochemical etching.75 The acid exfoliation method for MXene synthesis is depicted in Fig. 5.
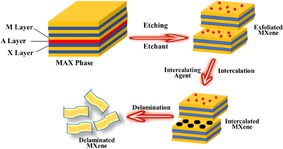 |
| Fig. 5 Synthesis of MXene by employing the acid exfoliation method (top-down approach). | |
It is noteworthy that specific types of MXene, such as MoC and MoN, cannot be synthesised using the top-down approach.80 Achieving precise control over particle lateral size, defects, and tribological properties is possible through bottom-up approaches, where atoms and molecules are assembled to form complex 2D MXene structures.81 Chemical vapour deposition (CVD) is a commonly employed method for MXene synthesis, providing several benefits over traditional top-down approaches.78 Plasma enhancement is utilised in the traditional chemical vapour deposition process to improve material quality and enable synthesis at reduced temperatures. The synthesis methods for MXenes are continuously evolving due to the rapid expansion of the domain.78 MXene synthesis via a bottom-up approach is shown in Fig. 6.
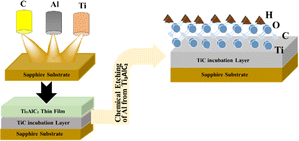 |
| Fig. 6 Synthesis of MXene using the chemical deposition method (bottom-up approach). | |
3.3 Surface functionalization
Functionalized Ti3C2T2 (T = N, O, F, S, and Cl) demonstrated the same metallic conductivity as bare Ti3C2. Among all the Ti3C2T2 units, Ti3C2S2, Ti3C2O2, and Ti3C2N2 have moderate adsorption power, which hinders lithium dissolution and shuttling. This Ti3C2T2 showed excellent electro catalytic activity for Li2S decomposition. Li2S decomposition barrier noticeably decreased from 3.390 eV to ∼0.4 eV using Ti3C2S2 and Ti3C2O2 via fast Li+ diffusivity.25 A boost in the performance of lithium–sulfur batteries is achieved using Ti3C2Tx, where T for the surface termination is –O, –F, and –OH as MXene nanosheet coating, which is a commercial Celgard membrane. Contrarily, the Ti3C2Tx MXene has an ultrathin two-dimensional structure and can form a uniform coating layer with a minimum mass loading of 0.1 mg cm−2 and a thickness of a mere 522 nm. At the expense of the enhanced electric conductivity and the efficient encapsulation of polysulfides, the lithium–sulfur battery.82 Simultaneously, the Li–S battery suffers from lithium polysulfide (LiPS) shuttling influence and a slow kinetics reaction. In this way, nano hybrid N-doped MXene-CoS2 (N-MX-CoS2) is developed through an in situ sulfidation strategy. The MXene-CoS2 chaperoned N-doped separator presents an excellent initial specific capacity of 1031 mA h g−1 at 1 °C, high-rate performance and outstanding cycle stability (0.52% per cycle).83 The simple formation of the thiourea-actuated wrinkled nitrogen and sulfur co-doped functionalized MXene (NSMX) in the separator to enhance ion diffusion and conversion kinetics is now feasible for high-energy LSBs. It should be mentioned that the LSBs with NSMX-modified separators showed a high specific capacity of 1249 mA h g−1. The LSBs delivered an excellent reversible capacity of 600 mA h g−1.84 3D S-CNT@MXene cages lower the electrode resistance and speed up the reaction rate. Consequently, the 3D S-CNT@MXene cage electrode emerges with a superior discharge capacity of 1375.1 mA h g−1. The first benefit lies in its high-rate capacity (910.3 and 557.3 mA h g−1) and outstanding heat transfer stability. Impressively, the composite electrode displays around zero capacity fading (656.3 mA h g−1), indicating the highest cycling stability reported so far among the whole Li–S cells.30 The MXene-based materials along with their different properties are presented in Table 1.
Table 1 MXene-based material and its properties
Material type |
Material synthesis |
Material efficiency |
Optimization of Li–S batteries |
References |
Ti3C2Tx MXene |
Ti3AlC2 treated with LiF/HCl, air-dried, stirred in DMSO, centrifuged, rinsed, sonicated, and dispersed in water |
Multilayered 2D Ti3C2Tx nano sheets used in Ti3C2Tx-PP separators with mass loadings 0.16–0.016 mg cm2 |
Maintained 640 mA h g−1 capacity after 200 cycles at 1 °C with 0.079% decay per cycle |
85 |
3D porous Ti3C2Tx MXene/rGO (MX/G) hybrid aerogel |
GO from Hummers' method, Ti3C2Tx synthesized via LiF/HCl etching, hydrothermal method, and freeze-drying |
Sulfur content ∼45 wt%, higher sulfur loading evaluated |
High capacity of 1270 mA h g−1 at 0.1 °C, extended cycling life up to 500 cycles with 0.07% decay per cycle, and high areal capacity of 5.27 mA h cm−2 |
86 |
Ti3C2 nanosheet/glass fiber composite |
Few-layered Ti3C2 via ultrasonication and vacuum filtration for composite |
Extremely thin, single-layered or few-layered Ti3C2 nanosheet on GF |
Initial discharge capacity of 820 mA h g−1 at 0.5 A g−1 and 721 mA h g−1 after 100 cycles |
87 |
Ti3C2-lithium film anode |
Atomic layers of Ti3C2 prepared by LiF in 6 M HCl, ultrasonication, freeze-drying, rolling and folding for anode |
Low overpotential, well-confined lithium plating |
Overpotential of 32 mV at 1.0 mA cm−2, 1.5% increase in 200 cycles, and flat voltage profiles |
88 |
MXene debris-coated eggshell membrane (MXene/ESM) |
Ti3C2 MXene hydrothermally reacted, ESM treated with HCl, coated with MXene debris |
Enhanced cycling stability compared to polypropylene separator |
Discharge capacity of 1321 mA h g−1 at 0.1 °C, and capacity retention of 74% after 250 cycles at 0.5 °C |
89 |
3D MnO2 nanosheets@delaminated-Ti3C2 (MNSs@d-Ti3C2) aerogel |
d-Ti3C2 via etching, ultrasonic treatment, MnO2 on d-Ti3C2 |
High specific surface area, mesoporous structure, and robust conductive pathway |
Initial discharge capacity of 1140 mA h g−1, 615 mA h g−1 at 2.0 °C, and 0.06% decay per cycle over 500 cycles at 1.0 °C |
90 |
Functionalized Ti3C2T2 MXenes (T = N, O, F, S, Cl) |
Total energy calculations for T-saturated Ti3C2 monolayers |
Reduced Li2S decomposition barrier and fast Li+ diffusivity |
Significantly decreased decomposition barrier and efficient Li+ transport |
25 |
Nanodot-interspersed Ti3C2Tx nanosheet (TCD-TCS) |
Ti3AlC2 treated with HF, dispersed in hydrosol, autoclaved, and freeze-dried |
High surface polar sites and enhanced structural integrity |
Discharge capacity at medium sulfur loading, the high volumetric capacity of 1957 mA h cm−3, and the high areal capacity of 13.7 mA h cm−2 |
91 |
Heterostructures of layered covalent triazine framework on Ti3C2 MXene nanosheets (CTF/TNS) |
Ti3C2 nanosheets via ultrasonic exfoliation, monomer heated, mixture ground, and washed with HCl |
High sulfur loading and efficient electron/ion transport |
Reversible capacity of 1441 mA h g−1, 0.014% decay rate over 1000 cycles, and 94% capacity retention after 100 cycles |
92 |
CO2-oxidized Ti3C2Tx MXenes components |
Ti3C2Tx prepared by LiF/HCl, CO2 oxidation at 900 °C |
High coulombic efficiency and retention of capacity |
Capacity of about 900 mA h g−1 after 300 cycles at 1 °C |
93 |
GO-d-Ti3C2Tx MXene aerogels with 3D reticular structure |
Ti3C2Tx via etching, centrifugation, freeze-drying, sonication, mixed with GO, and autoclaved |
Rapid LiPSs capture, improved capacity and cycling performance |
Discharge capacity of 1039 mA h g−1, 0.048% decay rate per cycle, and the areal capacity of 4.3 mA h cm−2 |
94 |
4. MXene as anode material
MXene, a recently discovered category of 2D materials, has garnered considerable interest in the energy storage sector due to its exceptional characteristics and wide range of uses.95 According to density functional theory (DFT) studies, V2CTx MXene has promising features for use in energy storage applications, such as lithium-ion batteries and supercapacitors.96 When Na ions are intercalated or adsorbed onto the surface of MXene nano sheets, the interlayer spacing between the layers of MXene increases from 7.1 to 10.1 Å.97 This V3C2/graphene heterostructure showcases an increased capacity and fast charge/discharge rates, indicating its promise as a highly effective electrode for ion batteries. This shows great potential, especially in the field of sodium-ion batteries.98 MXenes are recognised for their electrochemical activity, enabling them to exhibit pseudo-capacitance, resulting in a higher capacity than electrodes in double-layer capacitors and faster kinetics than intercalation-type electrodes.99 Garg and colleagues (2020) were among the first to publish the potential applications of MXenes as anodes in lithium-ion batteries. This newly discovered material demonstrates a surface area expansion that is about ten times larger than graphene.97 These applications have been utilised to enhance the performance and stability of separators, electrolytes, and electrodes.100 Several methods have been proposed to develop stable, dendrite-free metal anodes using MXene. The host designs are inspired by MXene, while the substrates are designed to be metalphilic. MXene-modified metal surfaces, MXene array construction, and MXene-decorated separators or electrolytes are all addressed.101 MXene has been extensively studied because it plays a significant role in various emerging composites. This composite anode effectively decreases the resistance to lithium ion movement at the interface between the lithium metal anode and the garnet solid-state electrolyte.102 MXene's surface functionalities allow it to undergo multiple reactions while maintaining its electrical conductivity.103 When O-terminated MXenes react with Mg, Ca, or Al, they decompose into bare MXenes, offering high capacities and excellent rate capabilities. The bare MXenes also demonstrate an outstanding performance.104 MXene has quickly gained recognition as the “next wonder material” following its introduction, setting it apart from other two-dimensional materials in the field.105
4.1 MXene anode in lithium–sulfur batteries
Lithium–sulfur batteries (LSBs) have become a focal point in the energy storage industry due to their remarkably high theoretical energy density and the cost efficiency of their active materials,106 which depend on the sulfur-lithium reversible redox reactions,34 and represent a promising alternative power source compared to existing lithium-ion batteries.107 Due to the natural availability of sulfur, high theoretical specific capacity (1675 mA h g−1), and high energy density (2670 W h kg−1), the lithium–sulfur battery is regarded as one of the most promising electrical energy storage devices.18 The significant volume change in S/Li2S (80%), which may damage the electrode structure, and the lithium dendrites on the anode developing throughout the charge/discharge process might cause the battery to short circuit.2 The limited utilization of active materials and unstable cycling performance are the issues that lithium–sulfur batteries are currently facing.108 There is a further decrease in performance due to the infamous shuttle effect. Porous-structured carbon materials are used as S hosts. Good electrical conductivity is provided by the carbon framework, and the shuttle effect is reduced by the porous structure.109 Carbon-based materials can only offer modest confinement towards lithium polysulfides, resulting in capacity degradation and low rate capability that occur when lithium sulphur batteries increasingly diffuse into the electrolyte.110 Fig. 7 illustrates the lithium metal anode used as a polysulfide blocker for lithium–sulfur batteries.
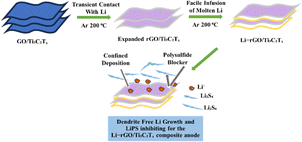 |
| Fig. 7 Representation of lithium metal anode with layered MXene, an effective polysulfide blocker for lithium–sulfur batteries. | |
To address the shuttle issue, Zhao and his colleagues (2020) investigated the lithium nitrate (LiNO3) addition, and electrolyte formulae such as ionic liquid and localized high-concentration electrolytes are also suggested.40 Experimental findings reveal that the Li-rich Li–Mg alloy is a promising anode material for Li–S batteries, as it forms a strong passivation layer on its surface, reducing side reactions.111 The electrochemical characteristics of Li–Mg alloys generated by kinetically controlled vapour deposition or direct alloying show that the tendency for Li dendrite development is greatly decreased on an electrode made of Li–Mg alloy.112 The development of macroscopic pores can be effectively reduced by the alloying process. However, the alloy also exhibits a restriction in delithiation due to diffusion control.113 MXenes are now among the most promising choices for anode materials.114 MXenes can intercalate massive ions and offer extraordinary capacities at high power rates over hundreds of cycles after building composites with graphene, metal oxides, transition metal dichalcogenides, and silicon.103 In a super capacitor, the working electrode created from free standing MXene paper exhibits a remarkable capacitance of approximately ≈490 F g−1 at 1 A g−1, a value that stands as one of the highest reported for super capacitor electrodes based on MXene.115 The cathodes, anodes, and separators of Li–S batteries use MXene-based materials, which have demonstrated the theoretical and experimental significance of high surface polarity and rich surface chemistry in poly-sulfide trapping. They are superior in reducing poly-sulfide shuttling and increasing sulphur utilization.3 Due to their unique properties, MXenes, a new category of 2D transition metal carbides and nitrides, have gained attention as promising materials for lithium–sulfur battery anodes. Their performance analysis requires a sophisticated approach that uses many graphs.116 The following graphs are crucial to comprehending how MXenes impact Li–S battery performance. The cyclic voltammetry graphs depict the relationship between the current and applied voltage throughout a voltage sweep. The electrochemical reactions occurring at the interface between an electrode and an electrolyte can be greatly understood from these curves. When anodes composed of MXene material have cathodic peaks on their CV curve, this usually means that the sulfur species has been converted to lithium sulfide (S8 → Li2S2) and has decreased, while the solid electrolyte interface layer, or SEI layer, has formed. Anodic peaks demonstrate how sulfur is created during the charging of the battery by oxidizing Li2S2.117 A region surrounded by the cyclic loop of CV indicates the electrode's potential specific capacity. The CV plots of pristine and cycled MXene electrodes make it easy to observe variations in the peak locations and peak currents. These differences suggest changes regarding the reversibility along with the kinetics of the process.118
4.2 Electrochemical performance
It is generally established that there is a strong association between material size and electrochemical performance. Smaller sizes result in higher electrochemically active regions and shorter ion diffusion distances. The connection stated greatly improves electrochemical performance.119 MXene and MXene-based nanomaterials show significant promise for energy storage applications.120 This two-dimensional MXene nano sheet serves as an outstanding conductive additive that improves electrochemical stability.121 MXenes, mainly Ti3C2Tx and its derivatives, are particularly effective in improving the electrochemical performances of lithium–sulfur (Li–S) batteries and are potential candidates for next-generation energy in various storage systems. MXenes have an optimal structure for electron conductivity and polysulfide adhesion, which are two of the most critical issues in Li–S battery science.122 A new 3D architectural electrode was created by integrating MXene and carbon nanotubes (CNTs) to overcome the issues of restacking and decreasing the specific capacity observed in MXene as an anode material.123 The utilization of lighter electrode materials frequently leads to an increase in capacity,124 as shown in Fig. 8.
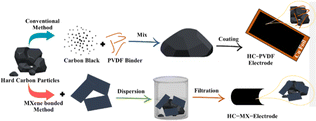 |
| Fig. 8 MXene-bonded hard carbon film as an electrode material. | |
MXenes are used in double-layer and redox-type ion storage, ion transfer control, electrodeposition substrates, batteries, and super capacitors. They increase electrode and electrolyte separator stability and performance.100 The synthesis of over 30 MXenes with controlled layer spacing and superconductivity expanded their use in electrode materials.125 MXene-based electrode materials have increased specific capacity and rate capability; they reduce or even completely stop the growth of dendrites on the metal anodes, extending the life of rechargeable batteries.126 One-component MXene electrodes struggle to attain high specific capacity, efficient ion/electron transport, and stable compatibility in electrochemical environments. Introducing nanomaterials between MXene layers increases electrochemical performance.127 Recent studies have shown that the creation of heterostructure nanocomposite from 2D MXenes and transition metal sulfides (TMS) or transition bimetal sulfides can enhance the specific capacitance, long-term cycling stability, and rate capability of MXene-based electrode materials.62 Compared to pristine TiNb2O7, the TNO@MXene composite has significantly improved lithium storage characteristics, including high reversible capacity (346.4 mA h g−1 at 0.1 °C), cycling stability (92.3% capacity retention after 500 cycles at 10 °C), and superior rate capability.128 The electrochemical efficacy of the Fe–Ti3C2Tx electrode is significantly improved (564.9 mA h g−1 at 50 mA g−1 at −10 °C). Over 500 cycles, the cycling stability of Fe–Ti3C2Tx was determined to be 418.8 mA h g−1 at 200 mA g−1 at −10 °C.129
With a high specific capacitance of 583 F g−1 at 1 A g−1, a decent rate capability of 82.5%, and an outstanding cycle stability of 96.5% at 5 A g−1 for over 5000 cycles, the heterogeneous 2D-layered MoS2/MXene nanohybrid MMX electrode displays a hybrid-type capacitance behavior.130 Fe–Ti3C2Tx electrode exhibits greatly enhanced electrochemical performance (564.9 mA h g−1 at 50 mA g−1 under −10 °C), surpassing that of pristine Fe–Ti3C2Tx (77 mA h g−1). The cycling stability of Fe–Ti3C2Tx for over 500 cycles (418.8 mA h g−1 at 200 mA g−1 under −10 °C). This work is expected to provide a guideline for developing brand-new MXene-based electrode materials with a high capacity for energy storage.129 Perpendicular MXene–Li arrays with tunable MXene walls and constants showed promise for dendritic-free and high-capacity lithium metal batteries due to their high specific capacity (2056 mA h g−1), long cycle life (1700 h), good rate capabilities of up to 2500 cycles (at 20 mA cm−2), and deep stripping and plating capability of up to 20 mA h cm−2.131 The unique two-dimensional sulfur-decorated Ti3C2 MXenes, as well as the self-enhanced kinetic and hybrid energy storage processes, are responsible for their superior electrochemical performance.132 The electrochemical properties in terms of current density, reversible capacity and cycle number are presented in Table 2.
Table 2 Electrochemical characteristics of MXene as anode for lithium battery
Material |
Current density |
Reversible capacity (mA h g−1) |
Cycle number (mA g−1) |
Reference |
Ti2C |
1 |
110 |
80 |
133 |
Ti2C |
1 |
123.6 |
75 |
134 |
Ti3C2 MXene |
— |
84 |
200 |
135 |
Ti3C2 |
200 |
203 |
500 |
136 |
Ti3C2 |
100 |
47.9 |
3000 |
137 |
Porous Ti3C2 |
3500 |
220 |
1000 |
138 |
Ti2C2Tx |
30 |
100 |
50 |
139 |
Ti2CNTx |
310 |
500 |
1000 |
140 |
V2C |
500 |
243 |
500 |
141 |
V4C3 |
1000 |
125 |
300 |
142 |
5. Enhanced battery performance
Energy storage plays a crucial role in shaping our future amidst rapid technological progress. Lithium–sulfur batteries are a promising option for high-energy-density storage systems positioned to revolutionize the electric vehicle and renewable energy sectors.30 Developing suitable anode materials has proven to be a challenging endeavour in the progression of Li–S battery technology.143 MXene, a recent innovation, may increase Li–S battery performance.144 High energy density, cost-effectiveness, and environmental friendliness make lithium–sulfur batteries an attractive energy storage option. However, several challenges have hindered their widespread adoption.145 Low sulfur-based cathode coulombic efficiency and cycle stability are important challenges.146 Li–S systems are not compatible with conventional graphite anodes for Li-ion batteries. MXene is used in energy storage because of its special qualities. These materials are perfect Li–S battery anodes because of their electrical conductivity, mechanical strength, and chemical stability.147 Battery performance significantly improves when MXene is used as the anode.148 The cobalt boride@MXene's interfacial electrical interaction for high-performance lithium–sulfur batteries is illustrated in Fig. 9.
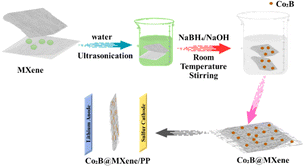 |
| Fig. 9 Interfacial electrical interaction of cobalt boride@MXene for high-performance lithium–sulfur batteries. | |
Overall, Li–S battery performance is enhanced by MXene's higher electrical conductivity.149 MXene permits high current densities for rapid charging and discharging, minimising energy loss and boosting battery power density in contrast to graphite anodes.92 Because of their longer lifespan and quicker charging times, Li–S batteries are gradually outperforming Li-ion batteries.150 The mechanical properties of MXene also enhance the structural stability of Li–S batteries. During cycling, sulphur cathodes expand and contract, resulting in electrode deformation and failure.151 These issues are resolved by MXene's robust mechanical properties, which maintain the anode's structure for the course of the battery's life.152 MXene anodes are recognised for their chemical stability, along with these benefits. When in contact with the reactive elements of the battery, they show reduced susceptibility to degradation.153 In addition, the widespread availability of MXene materials in nature, and their straightforward synthesis methods position them as a cost-effective option for anode materials.154 With the increasing demand for energy storage solutions, the cost-effectiveness of advanced battery technologies, such as Li–S, is becoming crucial for their widespread adoption.155 The cost advantage of MXene can lower Li–S battery prices overall, increasing its competitiveness in the market.156 In conclusion, MXene has proven to be a groundbreaking anode material for lithium–sulfur batteries. All these factors synergistically enhance battery performance: strong electrical conductivity, polysulfide-trapping ability, mechanical strength, and chemical stability.157 Despite a few remaining challenges, such as MXene's compatibility with different sulfur-based cathode materials and the necessity for large-scale production methods, the encouraging findings from research laboratories suggest that MXene is crucial for the progress of Li–S battery technology.158 Anticipate remarkable advancements in lithium–sulfur battery performance as researchers refine and optimise the technology, moving us closer to a sustainable and efficient energy storage solution for the future.159 Fig. 10 depicts the CoSe2-decorated MXenes as the cathode in lithium–sulfur batteries. Current and future batteries require anode materials with good conductivity and capacity. Batteries with sulfur (S) active ingredients in the electrode have an excellent specific capacity of around 1675 mA h g−1 (theoretical). The deposition or dissolution of sulfides (Li2Sm, m = 1, 2, 4, 6, 8) in electrolytes can significantly impact the cycle property and specific density of LSB. To address these problems, numerous efforts have gone into developing anode protection techniques, researching the latest developments, and discovering effective electrode additives.160 Different chemicals, especially carbon-based chemicals, have larger surface areas. These chemicals can be utilized in the electrochemical and lithium sulfide processes, and they have been considered very promising additions. Other strategies, such as doping of N or B, are needed to improve the competences of carbon materials in lithium sulfur batteries.85 In the meantime, the creation of novel additives may improve LSB performance. Several materials have been available recently and are utilized in the anodes of LSB, including metal oxides (MOs), transition metal–organic frameworks (MOFs), and transition metal dichalcogenides (TMDCs). In our growing mobile world, this can significantly improve ease of use and user experience. MXene-based lithium-ion (Li–S) batteries have a high capacity and the potential to be economically viable, which makes them perfect for large-scale systems that store energy.161 Furthermore, it has been shown that metalization materials can enhance Li–S battery performance.22
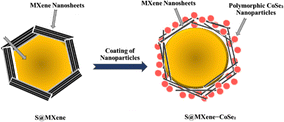 |
| Fig. 10 Representation of CoSe2-decorated MXenes as cathodes in lithium–sulfur batteries. | |
5.1 Mechanistic insights
Lithium–sulfur batteries are considered a potential solution to meet the increasing demand for high-energy-density options in the quest for more effective and environmentally friendly energy storage systems.162 The use of MXene materials has revolutionised this industry. These compounds have improved Li–S battery performance and revealed their mechanisms.163 MXene materials have unique qualities and structures that make them ideal for improving Li–S battery efficiency.164 Finding the mechanics underlying MXene materials in Li–S batteries has revealed electrochemical reactions and has led to better, more lasting energy storage technologies.165 Trapping and immobilising polysulfides (Fig. 11) in Li–S batteries are difficult; therefore, this mechanistic finding is significant. Sulfur-based cathodes create soluble lithium polysulfides that migrate to the anode during cycling, reducing capacity and performance.82 Due to their two-dimensional structure and immense surface area, MXene materials prevent polysulfide diffusion.166 Spectroscopy and microscopy showed that MXene is a polysulfide “sponge”, demonstrating the process. MXene traps polysulfides to improve Li–S battery performance and endurance.166
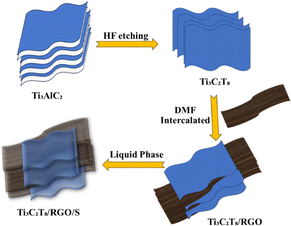 |
| Fig. 11 Depiction of MXene/reduced graphene for trapping sulfur and polysulfides. | |
MXene materials provide excellent electrical conductivity, which is important for Li–S batteries. High current densities for quick charging and discharging require conductivity higher than that of graphite.167 Energy dissipation and battery power density decrease due to this constraint. By facilitating quick charge transfer, MXene materials boost Li–S battery energy production and make them ideal for many applications.168 MXene's remarkable mechanical capabilities are crucial for Li–S battery structural integrity. Sulphur cathodes expand and compress during cycling, deforming and perhaps failing. MXene's superior mechanical strength solves these difficulties.169 Through scanning electron microscopy, MXene-supported anodes have been shown to preserve their structural integrity throughout the battery's lifetime. Understanding how MXene materials retain anode structure is essential for improving Li–S battery performance.170
The chemical stability of MXene materials in Li–S batteries provides significant mechanistic insights. When in touch with the battery's reactive components, MXene resists breakdown better than other anodes.171 Improved chemical stability extends the calendar life, which is important for electric cars and renewable energy storage. Through extensive investigation using multiple analytical techniques, MXene's chemical stability has been shown to affect Li–S battery efficiency.122 The use of MXene materials in Li–S batteries has improved their performance and opened the road for energy storage technological developments.172 Researchers are improving MXene material synthesis, compatibility with other cathode materials, and large-scale manufacturing techniques to commercialize this approach.173 MXene illuminates Li–S battery issues, such as polysulfide migration, poor conductivity, and structural instability.174 These findings have impacted Li–S battery research and development, predicting future advancements. Their energy storage competitiveness and sustainability should improve with this advancement. MXene compounds might improve Li–S batteries and accelerate energy transition.174
5.2 Environmental considerations
Lithium–sulfur batteries have received a lot of attention lately because of their potential to completely change the energy storage industry. There are many environmental and sustainable benefits associated with using MXenes as the anode material in these batteries.174 MXenes have shown a lot of potential for increasing Li–S battery lifespan and efficiency.89 Lithium and sulphur work together to provide a larger potential energy density because Li–S batteries have a higher energy density than regular Li-ion batteries.175 Li–S batteries store more energy in smaller areas. MXenes can boost Li–S battery energy density as anodes. Energy storage systems using Li–S batteries reduce their environmental impact. Sulphur, a key ingredient of Li–S batteries, is cheap and abundant, making it an eco-friendly option.176 Li–S batteries lack harmful heavy elements, such as cobalt, unlike lithium-ion batteries. This reduces environmental and ethical issues related to battery mining and production.177 The environmental impact of Li–S batteries may be reduced with MXenes, which are made of easily accessible components.178 Long-term environmental consequences, durability, and recycling should be considered when assessing batteries. Li–S batteries may last longer than lithium-ion batteries. MXenes improve Li–S battery stability as an anode.179 MXenes may help manage sulphur expansion and contraction, extending battery life, decreasing replacements, and preserving waste.175 Recycling and reuse are essential to sustainability. Due to its lower usage of hazardous and valuable components, Li–S batteries are easier to recycle than lithium-ion batteries.180 MXenes may improve recycling. These 2D materials may be reused to make batteries due to their stability. This reduces environmental effects by avoiding fresh raw material extraction and processing, thereby contributing to a circular economy.181 Safety is essential to sustainability. Due to the decreased thermal runaway danger, Li–S batteries are safer than lithium-ion batteries.182 The thermal stability and flame-retardant qualities of MXenes reduce battery fires and explosions, thereby improving energy storage system safety and sustainability.183
6. MXene stability in Li–S batteries
Stability is crucial to the lifetime and performance of lithium–sulfur batteries using MXene anodes.184 MXene may solve Li–S battery difficulties. However, stability across many charge–discharge cycles remains the major goal.4 Li–S batteries using MXene as the anode dissolve lithium polysulfides (LiPS), causing stability concerns. LiPSs are formed during battery usage and may migrate into the electrolyte, reducing their cycle life and capacity.185 MXene may mitigate this issue. During sulfur-to-lithium sulphide conversion, LiPS production at the anode is decreased by its good electrical conductivity. The large surface area of MXenes collects and immobilises LiPS, preventing them from escaping into the electrolyte.186 MXene has benefits but does not solve LiPS migration and disintegration. A few LiPS may still enter the electrolyte, threatening battery stability.187 Thus, research is developing new electrolyte formulations and separator materials to reduce LiPS migration and improve stability.188 The stability and structural integrity of the MXene anode during charge–discharge cycles are concerns. Li–S reactions cause volume variations in the anode material, which may deteriorate or pulverise it. Due to its endurance and flexibility, MXene may assist in solving these problems.189 Its two-dimensional structure allows it to handle volume fluctuations better than three-dimensional anodes. In rare circumstances, cycle expansion and contraction may destroy structures. Composite materials and new electrode designs are being investigated to improve MXene anode mechanical stability.190 Thermal stability is also important, and Li–S batteries may overheat, causing thermal runaway, which is a serious failure.191 High thermal conductivity makes MXene efficient in heat dissipation. Cell designs, safety features, and sophisticated thermal management systems must be improved to provide Li–S battery thermal stability.192 Long-term storage stability is also significant. MXene-anode Li–S batteries may self-discharge and lose capacity after complicated storage. The problem is LiPS reactivity, which absorbs lithium ions even when the battery is off.193 Increasing Li–S battery stability and reducing self-discharge are persistent problems. MXene is a promising Li–S battery anode.194 For MXene to reach its full potential in Li–S batteries and ensure long-term stability and reliability, collaboration among materials scientists, chemists, and engineers is essential. Overcoming stability issues is crucial for the successful implementation of Li–S batteries with MXene anodes in various applications, such as energy storage and electric vehicles.195 It is crucial to thoroughly investigate the challenges related to MXene stability during cycling in lithium–sulfur batteries when used as an anode material for the advancement of this promising energy storage technology.196 MXenes possess several benefits, such as robust electrical conductivity and the ability to facilitate sulphur growth. However, there are also notable challenges that need to be addressed. MXenes demonstrate the ability to undergo structural and chemical modifications during repeated charge and discharge cycles, leading to enhanced electrochemical stability. Instability can lead to a loss of electrical conductivity and structural integrity.197 Researchers are currently working on enhancing the electrochemical stability of MXenes through modifications to their surface chemistry and structure.198 Understanding the interaction between sulphur and MXenes is essential for optimizing the performance of Li–S batteries. Sulphur reacts with MXenes during cycling, forming undesirable intermediate compounds.199 Lowering the battery's reversible capacity may affect cycle stability. Controlling and optimising sulfur-MXene interactions is difficult.31 MXene nanoparticles may agglomerate, lowering the lithium-ion adsorption surface area and active sites. This may reduce battery capacity and performance. Researchers are using advanced materials engineering to reduce particle aggregation.200 Due to their sensitivity, MXenes in Li–S batteries require careful electrolyte and solvent selection. Certain electrolyte and solvent combinations may damage the MXene anode material, reducing stability and performance. This challenge requires finding acceptable electrolyte systems.201 For practical use, Li–S batteries must function consistently. MXene stability issues connected to long-term performance require an anode material that can withstand many charge–discharge cycles without deteriorating.202 Researchers are using surface functionalization, hybrid nanocomposite design, and improved manufacturing methods to address these challenges.203 Electric cars, renewable energy storage, and portable electronics might benefit from the high energy density and sustainability of Li–S batteries.204
7. Strategies for MXene-based anode improvement
Li–S batteries are inexpensive and have a high theoretical energy density, making them a good energy storage option. Significant difficulties exist, especially with anode materials.205 Li–S battery anodes using MXenes seem promising. The high electrical conductivity, large surface area, and mechanical resilience of MXenes make them excellent for overcoming traditional anodes.129 Researchers have investigated ways to improve MXene-based Li–S battery anodes to maximize their potential.206 Optimal Li–S battery anode performance requires the right sulphur incorporation and MXene compatibility.207 Sulphur species may be linked to MXene surface functional groups. Recent investigations have found ways to link sulphur and MXenes for stable electrochemical reactions. Surface modifications and functionalization generate chemical linkages that prevent sulphur species diffusion during charge/discharge cycles.208 Developing nanostructured composites through the combination of MXenes with other nanomaterials, such as carbon nanotubes, graphene, or metal oxides, provides a synergistic approach.209 These composites exhibit superior mechanical stability, electrical conductivity, and lithium-ion diffusion. They help manage the volume changes that happen during lithium-ion intercalation, minimizing structural damage and enhancing battery longevity.210 Dealing with the production of soluble polysulfides during discharge remains a significant hurdle in Li–S batteries. Polysulfides may migrate from the cathode to the anode, leading to a decrease in capacity over time.211 To solve this problem, scientists have developed surface coatings for MXene-based anodes. The coatings effectively capture and immobilize polysulfides, preventing their migration and reducing the impact of the “polysulfide shuttle” effect. Materials such as polymers or metal oxides, which have a strong attraction to polysulfides, are commonly utilized in these coatings.212 Enhancing the porosity of MXene-based anodes leads to a significant improvement in their performance. High surface area and porosity facilitate increased sulphur loading, improved electrolyte infiltration, and faster ion diffusion.213 Researchers have used various methods to incorporate porous patterns into MXene-based anodes, such as templating, chemical etching, and controlled oxidation. The enhancement of electrochemical activity and battery performance is evident in these structures.214 Choosing the right electrolyte is crucial for the performance of Li–S batteries and significantly affects the behavior of the anode. Scientists have explored tailored electrolyte formulations to enhance their compatibility with MXene-based anodes.215 Electrolytes possessing suitable ionic conductivity, stability, and chemical composition play a crucial role in enhancing anode electrochemical performance while mitigating undesired side reactions.216 There is a growing emphasis on environmental and sustainability considerations in the advancement of Li–S batteries. MXenes are known to be recyclable materials that house valuable transition metals.217 Investigations are currently underway to explore strategies for sustainable MXene production and recycling to minimize the waste and environmental impact of Li–S battery manufacture.218 A crucial phase in the development of MXene-based anodes is converting laboratory research into practical commercial uses.219 Assessing the practicality and reliability of these anodes involves real-world testing, such as pilot-scale production and battery testing. MXenes may be viable Li–S battery anodes due to their unique characteristics. MXenes are improving energy storage technologies in collaboration with current research and development.220 Researchers are making progress towards high-performance, cost-effective, and sustainable Li–S batteries. These batteries will be vital to greener, more efficient energy storage. MXene-based anodes may improve Li–S anodes and boost energy storage technology.221
8. Future directions in advancing lithium–sulfur batteries
Lithium–sulfur batteries provide an abundance of intriguing opportunities and technical difficulties that require further research and development. The significance of developing robust cathode composites made of carbon and investigating hybrid electrolyte methods to progress the creation of long-lasting Li–S batteries. The value of using electrocatalysts and novel electrode materials lies in solving the problems associated with lithium polysulfides. However, Li–S batteries are far more promising compared with these little advancements. This joint endeavour might revolutionize high-capacity, sustainable energy storage technologies by exploring material genomes and integrating machine-learning methods. Significant progress is expected in the development of Li–S batteries using materials based on MXene, in line with these overall objectives. Tackling technical issues includes increasing sulphur loading as well as utilizing and enhancing the stability of the materials for lithium anodes. The development of MXene-based Li–S batteries has accelerated owing to high-throughput material screening and optimization that use computational design and material genome techniques. All things considered, Li–S batteries seem to have a bright future, particularly when the surface functionalization of MXene materials is prioritized. This combination of advanced surface modification methods and the ongoing development of MXene-based composite anodes may lead to promising advancements in energy storage technology. The next mission emphasizes how important it is to continue materials science and engineering research to overcome current obstacles and achieve MXene's full potential in Li–S batteries.
9. Conclusions
This study highlights the significant progress achieved in enhancing the performance of MXene-based anodes for this specific energy storage application. Enhancing and developing MXene interfaces have become essential approaches to solving problems in lithium–sulfur batteries. This study has provided insights into the potential advantages and drawbacks of these novel materials through a detailed analysis of the vast range of MXene modifications and their impact on anode performance. Customized MXene interface designs have been shown to be successful in reducing issues such as electrode instability, polysulfide shuttling, and volume expansion, which eventually improve battery performance. It is now possible to enhance the anode's electrochemical properties by including MXene derivatives, functional additives, and nanocomposites. This leads to a longer cycle life, better cycling stability, and increased utilization of sulphur. Moreover, an improved understanding of MXene's interactions with lithium and sulphur species has made it possible to create strategic interface designs that enhance the control of the charge/discharge process. As with any new technology, there are always challenges. The long-term stability, affordability, and scalability of MXene-based anodes require further investigation. To accelerate the commercialization of MXene interfaces in lithium–sulfur batteries, these problems need to be resolved in future research. In summary, the comprehensive analysis of the MXene interface design provides guidance for further research aimed at optimizing the potential of these materials for the advancement of lithium–sulfur battery technology. The study underscores MXene's potential as the leading option for customized anode creation, hence enhancing the lifespan and performance of lithium–sulfur batteries.
Data availability
Data will be provided on request.
Author contributions
Zeshan Ali Sandhu and Muhammad Asam Raza have supervised the project, Kainat Imtiaz and Adnan Ashraf have collected the data, Areej Tabasum and Sajawal Khan have compile the data, Ali Haider Bhalli has drafted the final version, Umme Farwa has drawn the whole figures while Abdullah G. Al-Sehemi have helped in polishing the final draft and give the financial assistant.
Conflicts of interest
Authors declare that they have no conflict or competing interest.
Acknowledgements
The Deanship of Research and Graduate Studies at King Khalid University is greatly appreciated for funding this work through Large Research Project under grant number RGP2/378/45.
References
- A. VahidMohammadi, J. Rosen and Y. Gogotsi, Science, 2021, 372, eabf1581 CrossRef CAS PubMed.
- Q. Zhao, Q. Zhu, Y. Liu and B. Xu, Adv. Funct. Mater., 2021, 31, 2100457 CrossRef CAS.
- Z. Xiao, Z. Li, X. Meng and R. Wang, J. Mater. Chem. A, 2019, 7, 22730–22743 RSC.
- H. Tang, W. Li, L. Pan, K. Tu, F. Du, T. Qiu, J. Yang, C. P. Cullen, N. McEvoy and C. Zhang, Adv. Funct. Mater., 2019, 29, 1901907 CrossRef.
- Y. Wang, J. Shen, L.-C. Xu, Z. Yang, R. Li, R. Liu and X. Li, Phys. Chem. Chem. Phys., 2019, 21, 18559–18568 RSC.
- J. Balach and L. Giebeler, J. Phys.: Energy, 2021, 3, 021002 CAS.
- L. Zhang, J. Bi, Z. Zhao, Y. Wang, D. Mu and B. Wu, Electrochim. Acta, 2021, 370, 137759 CrossRef CAS.
- Z. Zhang, J. Gou, K. Cui, X. Zhang, Y. Yao, S. Wang and H. Wang, Nano-Micro Lett., 2024, 16, 181 CrossRef CAS PubMed.
- Y. Feng, G. Wang, J. Ju, Y. Zhao, W. Kang, N. Deng and B. Cheng, Energy Storage Mater., 2020, 32, 320–355 CrossRef.
- X. Zhu, M. Ge, T. Sun, X. Yuan and Y. Li, J. Phys. Chem. Lett., 2023, 14, 2215–2221 CrossRef CAS PubMed.
- M. Khan, S. Yan, M. Ali, F. Mahmood, Y. Zheng, G. Li, J. Liu, X. Song and Y. Wang, Nano-Micro Lett., 2024, 16, 179 CrossRef CAS PubMed.
- W.-D. Liu, X. Tang, J.-A. Feng, C.-Y. Zhang, H. Liu, C. Shi, X.-X. Zhao and J.-J. Song, Rare Met., 2024, 43, 455–477 CrossRef CAS.
- Y. Zhang, C. Ma, W. He, C. Zhang, L. Zhou, G. Wang and W. Wei, Prog. Nat. Sci.: Mater. Int., 2021, 31, 501–513 CrossRef CAS.
- B. B. Gicha, L. T. Tufa, N. Nwaji, X. Hu and J. Lee, Nano-Micro Lett., 2024, 16, 172 CrossRef CAS PubMed.
- G. Wang, G. Wang, L. Fei, L. Zhao and H. Zhang, Nano-Micro Lett., 2024, 16, 150 CrossRef CAS PubMed.
- X. Liang, J. Yun, Y. Wang, H. Xiang, Y. Sun, Y. Feng and Y. Yu, Nanoscale, 2019, 11, 19140–19157 RSC.
- X. Liang and L. F. Nazar, 2D Metal Carbides and Nitrides (Mxenes) Structure, Properties and Applications, 2019, pp. 381–398 Search PubMed.
- C. Zhang, L. Cui, S. Abdolhosseinzadeh and J. Heier, InfoMat, 2020, 2, 613–638 CrossRef CAS.
- M. Pandey, K. Deshmukh and S. K. Pasha, in Mxenes and their Composites, Elsevier, 2022, pp. 343–369 Search PubMed.
- Y. Gogotsi and B. Anasori, ACS Nano, 2019, 13, 8491–8494 CrossRef CAS PubMed.
- J. Tian, G. Ji, X. Han, F. Xing and Q. Gao, Int. J. Mol. Sci., 2022, 23, 6329 CrossRef CAS PubMed.
- D. Rao, L. Zhang, Y. Wang, Z. Meng, X. Qian, J. Liu, X. Shen, G. Qiao and R. Lu, J. Phys. Chem. C, 2017, 121, 11047–11054 CrossRef CAS.
- M. Xia, B. Chen, F. Gu, L. Zu, M. Xu, Y. Feng, Z. Wang, H. Zhang, C. Zhang and J. Yang, ACS Nano, 2020, 14, 5111–5120 CrossRef CAS PubMed.
- Y.-H. Liu, L.-X. Li, A.-Y. Wen, F.-F. Cao and H. Ye, Energy Storage Mater., 2023, 55, 652–659 CrossRef.
- D. Wang, F. Li, R. Lian, J. Xu, D. Kan, Y. Liu, G. Chen, Y. Gogotsi and Y. Wei, ACS Nano, 2019, 13, 11078–11086 CrossRef CAS PubMed.
- T. Peng, N. Zhang, Y. Yang, M. Zhang, R. Luo, C. Chen, Y. Lu and Y. Luo, Small, 2022, 18, 2202917 CrossRef CAS PubMed.
- S.-Y. Qiu, C. Wang, Z.-X. Jiang, L.-S. Zhang, L.-L. Gu, K.-X. Wang, J. Gao, X.-D. Zhu and G. Wu, Nanoscale, 2020, 12, 16678–16684 RSC.
- W. Li, Y. Zhang, H. Li, Z. Chen, T. Shang, Z. Wu, C. Zhang, J. Li, W. Lv and Y. Tao, Batteries Supercaps, 2020, 3, 892–899 CrossRef CAS.
- Q. Zhang, X. Zhang, Y. Xiao, C. Li, H. H. Tan, J. Liu and Y. Wu, ACS Omega, 2020, 5, 29272–29283 CrossRef CAS PubMed.
- H. Wang, S.-A. He, Z. Cui, C. Xu, J. Zhu, Q. Liu, G. He, W. Luo and R. Zou, Chem. Eng. J., 2021, 420, 129693 CrossRef CAS.
- Q. Zhu, H.-F. Xu, K. Shen, Y.-Z. Zhang, B. Li and S.-B. Yang, Rare Met., 2022, 41, 311–318 CrossRef CAS.
- Y. Cui, X. Zhou, X. Huang, L. Xu and S. Tang, ACS Appl. Mater. Interfaces, 2023, 15, 49223–49232 CrossRef CAS PubMed.
- Q. Wang, S. Qiao, C. Huang, X. Wang, C. Cai, G. He and F. Zhang, ACS Appl. Mater. Interfaces, 2024, 16, 24502–24513 CrossRef CAS PubMed.
- Y. Chen, T. Wang, H. Tian, D. Su, Q. Zhang and G. Wang, Adv. Mater., 2021, 33, 2003666 CrossRef CAS PubMed.
- R. Deng, M. Wang, H. Yu, S. Luo, J. Li, F. Chu, B. Liu and F. Wu, Energy Environ. Mater., 2022, 5, 777–799 CrossRef CAS.
- T. Z. Hou, X. Chen, H. J. Peng, J. Q. Huang, B. Q. Li, Q. Zhang and B. Li, Small, 2016, 12, 3283–3291 CrossRef CAS PubMed.
- H. Li, Y. Li and L. Zhang, SusMat, 2022, 2, 34–64 CrossRef CAS.
- L. Fang, Z. Feng, L. Cheng, R. E. Winans and T. Li, Small Methods, 2020, 4, 2000315 CrossRef CAS.
- Y. Schütze, R. de Oliveira Silva, J. Ning, J. Rappich, Y. Lu, V. G. Ruiz, A. Bande and J. Dzubiella, Phys. Chem. Chem. Phys., 2021, 23, 26709–26720 RSC.
- M. Zhao, B.-Q. Li, X.-Q. Zhang, J.-Q. Huang and Q. Zhang, ACS Cent. Sci., 2020, 6, 1095–1104 CrossRef CAS PubMed.
- M. Wild and G. J. Offer, Lithium–sulfur batteries, John Wiley & Sons, 2019 Search PubMed.
- X. Yang, X. Li, K. Adair, H. Zhang and X. Sun, Electrochem. Energy Rev., 2018, 1, 239–293 CrossRef CAS.
- T. Z. Hou, W. T. Xu, X. Chen, H. J. Peng, J. Q. Huang and Q. Zhang, Angew. Chem., 2017, 129, 8290–8294 CrossRef.
- H. J. Peng, J. Q. Huang, X. B. Cheng and Q. Zhang, Adv. Energy Mater., 2017, 7, 1700260 CrossRef.
- X. Chen, T. Hou, K. A. Persson and Q. Zhang, Mater. Today, 2019, 22, 142–158 CrossRef CAS.
- M. Zhao, X. Chen, X. Y. Li, B. Q. Li and J. Q. Huang, Adv. Mater., 2021, 33, 2007298 CrossRef CAS PubMed.
- C. Wei, Y. Wang, Y. Zhang, L. Tan, Y. Qian, Y. Tao, S. Xiong and J. Feng, Nano Res., 2021, 14, 3576–3584 CrossRef CAS.
- Y.-H. Liu, C.-Y. Wang, S.-L. Yang, F.-F. Cao and H. Ye, J. Energy Chem., 2022, 66, 429–439 CrossRef CAS.
- Q. Zhao, Q. Zhu, J. Miao, P. Zhang and B. Xu, Nanoscale, 2019, 11, 8442–8448 RSC.
- J. Huang, Z. Li, Y. Mao and Z. Li, Nano Sel., 2021, 2, 1480–1508 CrossRef CAS.
- Y. Wang, Y. Xu, M. Hu, H. Ling and X. Zhu, Nanophotonics, 2020, 9, 1601–1620 CrossRef CAS.
- Y. Wu, Y. Sun, J. Zheng, J. Rong, H. Li and L. Niu, J. Phys.: Energy, 2021, 3, 032009 CAS.
- H. Shao, S. Luo, A. Descamps-Mandine, K. Ge, Z. Lin, P. l. Taberna, Y. Gogotsi and P. Simon, Adv. Sci., 2023, 10, 2205509 CrossRef CAS PubMed.
- M. A. Saeed, A. Shahzad, K. Rasool, F. Mateen, J. M. Oh and J. W. Shim, Adv. Sci., 2022, 9, 2104743 CrossRef CAS PubMed.
- M. Carey and M. Barsoum, Mater. Today Adv., 2021, 9, 100120 CrossRef CAS.
- B. Anasori and Y. Gogotsi, 2D Metal Carbides and Nitrides (MXenes) Structure, Properties and Applications, 2019, pp. 3–12 Search PubMed.
- M. Pogorielov, K. Smyrnova, S. Kyrylenko, O. Gogotsi, V. Zahorodna and A. Pogrebnjak, Nanomaterials, 2021, 11, 3412 CrossRef CAS PubMed.
- Z. Bao, C. Lu, X. Cao, P. Zhang, L. Yang, H. Zhang, D. Sha, W. He, W. Zhang and L. Pan, Chin. Chem. Lett., 2021, 32, 2648–2658 CrossRef CAS.
- M. Naguib, M. W. Barsoum and Y. Gogotsi, Adv. Mater., 2021, 33, 2103393 CrossRef CAS PubMed.
- A. Feng, T. Hou, Z. Jia, Y. Zhang, F. Zhang and G. Wu, Nanomaterials, 2020, 10, 162 CrossRef CAS PubMed.
- A. Bafekry, B. Akgenç, M. Ghergherehchi and F. Peeters, J. Phys.: Condens. Matter, 2020, 32, 355504 CrossRef CAS PubMed.
- S. Nahirniak, A. Ray and B. Saruhan, Batteries, 2023, 9, 126 CrossRef CAS.
- Z.-J. Cao, Y.-Z. Zhang, Y.-L.-S. Cui, B. Li and S.-B. Yang, Tungsten, 2020, 2, 162–175 CrossRef.
- J. Zhu, E. Ha, G. Zhao, Y. Zhou, D. Huang, G. Yue, L. Hu, N. Sun, Y. Wang and L. Y. S. Lee, Coord. Chem. Rev., 2017, 352, 306–327 CrossRef CAS.
- A. Zamhuri, G. P. Lim, N. L. Ma, K. S. Tee and C. F. Soon, Biomed. Eng., 2021, 20, 33 Search PubMed.
- B. Shao, Z. Liu, G. Zeng, H. Wang, Q. Liang, Q. He, M. Cheng, C. Zhou, L. Jiang and B. Song, J. Mater. Chem. A, 2020, 8, 7508–7535 RSC.
- J. Chen, Y. Ding, D. Yan, J. Huang and S. Peng, SusMat, 2022, 2, 293–318 CrossRef CAS.
- K. Hu, H. Wang, X. Zhang, H. Huang, T. Qiu, Y. Wang, C. J. Zhang, L. Pan and J. Yang, Chem. Eng. J., 2021, 408, 127283 CrossRef CAS.
- K. Qu, K. Huang and Z. Xu, Front. Chem. Sci. Eng., 2021, 15, 820–836 CrossRef CAS.
- I. Ali, M. Faraz Ud Din and Z.-G. Gu, Molecules, 2022, 27, 4925 CrossRef CAS PubMed.
- Y. Cheng, W. Lyu, Z. Wang, H. Ouyang, A. Zhang, J. Sun, T. Yang, B. Fu and B. He, Nanotechnology, 2021, 32, 392003 CrossRef CAS PubMed.
- L. Liang, L. Niu, T. Wu, D. Zhou and Z. Xiao, ACS Nano, 2022, 16, 7971–7981 CrossRef CAS PubMed.
- T.-Y. Shuai, Z. Qi-Ni, H. Xu, C.-J. Huang, Z. Zhi-Jie and G.-R. Li, Chem. Commun., 2023, 3968–3999 RSC.
- S. Venkateshalu and A. N. Grace, Appl. Mater. Today, 2020, 18, 100509 CrossRef.
- K. Chaturvedi, V. Hada, S. Paul, B. Sarma, D. Malvi, M. Dhangar, H. Bajpai, A. Singhwane, A. K. Srivastava and S. Verma, Top. Curr. Chem., 2023, 381, 11 CrossRef CAS PubMed.
- L. Verger, C. Xu, V. Natu, H.-M. Cheng, W. Ren and M. W. Barsoum, Curr. Opin. Solid State Mater. Sci., 2019, 23, 149–163 CrossRef CAS.
- L. Verger, V. Natu, M. Carey and M. W. Barsoum, Trends Chem., 2019, 1, 656–669 CrossRef CAS.
- K. Wasnik, M. D. Pawar, L. R. Raphael, A. Pullanchiyodan, M. V. Shelke and P. Raghavan, J. Mater. Res., 2022, 37, 3865–3889 CrossRef CAS.
- L. Liu, M. Orbay, S. Luo, S. Duluard, H. Shao, J. Harmel, P. Rozier, P.-L. Taberna and P. Simon, ACS Nano, 2021, 16, 111–118 CrossRef PubMed.
- L. Huang, L. Ding and H. Wang, Small Sci., 2021, 1, 2100013 CrossRef CAS.
- M. Malaki and R. S. Varma, Adv. Mater., 2020, 32, 2003154 CrossRef CAS PubMed.
- J. Song, D. Su, X. Xie, X. Guo, W. Bao, G. Shao and G. Wang, ACS Appl. Mater. Interfaces, 2016, 8, 29427–29433 CrossRef CAS PubMed.
- C. Yang, Y. Li, W. Peng, F. Zhang and X. Fan, Chem. Eng. J., 2022, 427, 131792 CrossRef CAS.
- J. Feng, W. Liu, C. Shi, C. Zhang, X. Zhao, T. Wang, S. Chen, Q. Li and J. Song, Energy Storage Mater., 2024, 67, 103328 CrossRef.
- N. Li, Y. Xie, S. Peng, X. Xiong and K. Han, J. Energy Chem., 2020, 42, 116–125 CrossRef.
- J. Song, X. Guo, J. Zhang, Y. Chen, C. Zhang, L. Luo, F. Wang and G. Wang, J. Mater. Chem. A, 2019, 7, 6507–6513 RSC.
- C. Lin, W. Zhang, L. Wang, Z. Wang, W. Zhao, W. Duan, Z. Zhao, B. Liu and J. Jin, J. Mater. Chem. A, 2016, 4, 5993–5998 RSC.
- B. Li, D. Zhang, Y. Liu, Y. Yu, S. Li and S. Yang, Nano energy, 2017, 39, 654–661 CrossRef CAS.
- L. Yin, G. Xu, P. Nie, H. Dou and X. Zhang, Chem. Eng. J., 2018, 352, 695–703 CrossRef CAS.
- H. Zhang, Q. Qi, P. Zhang, W. Zheng, J. Chen, A. Zhou, W. Tian, W. Zhang and Z. Sun, ACS Appl. Energy Mater., 2018, 2, 705–714 CrossRef.
- Z. Xiao, Z. Li, P. Li, X. Meng and R. Wang, ACS Nano, 2019, 13, 3608–3617 CrossRef CAS PubMed.
- R. Meng, Q. Deng, C. Peng, B. Chen, K. Liao, L. Li, Z. Yang, D. Yang, L. Zheng and C. Zhang, Nano Today, 2020, 35, 100991 CrossRef CAS.
- D. K. Lee, Y. Chae, H. Yun, C. W. Ahn and J. W. Lee, ACS Nano, 2020, 14, 9744–9754 CrossRef CAS PubMed.
- X. Tang, R. Gan, L. Tan, C. Tong, C. Li and Z. Wei, ACS Appl. Mater. Interfaces, 2021, 13, 55235–55242 CrossRef CAS PubMed.
- R. Liu, W. Cao, D. Han, Y. Mo, H. Zeng, H. Yang and W. Li, J. Alloys Compd., 2019, 793, 505–511 CrossRef CAS.
- S. A. Zahra, B. Anasori, M. Z. Iqbal, F. Ravaux, M. Al Tarawneh and S. Rizwan, APL Mater., 2022, 10 DOI:10.1063/5.0087457.
- R. Garg, A. Agarwal and M. Agarwal, Mater. Res. Express, 2020, 7, 022001 CrossRef CAS.
- P. P. Dinda and S. Meena, J. Phys.: Condens. Matter, 2021, 33, 175001 CrossRef CAS PubMed.
- G. Zhu, H. Zhang, J. Lu, Y. Hou, P. Liu, S. Dong, Y. Zhang and X. Dong, Mater. Today Sustain., 2022, 20, 100226 CrossRef.
- X. Li, Z. Huang, C. E. Shuck, G. Liang, Y. Gogotsi and C. Zhi, Nat. Rev. Chem, 2022, 6, 389–404 CrossRef PubMed.
- C. Wei, Y. Tao, Y. An, Y. Tian, Y. Zhang, J. Feng and Y. Qian, Adv. Funct. Mater., 2020, 30, 2004613 CrossRef CAS.
- J. Wen, L. Huang, Y. Huang, W. Luo, H. Huo, Z. Wang, X. Zheng, Z. Wen and Y. Huang, Energy Storage Mater., 2022, 45, 934–940 CrossRef.
- M. Greaves, S. Barg and M. A. Bissett, Batteries Supercaps, 2020, 3, 214–235 CrossRef CAS.
- Y. Xie, Y. Dall'Agnese, M. Naguib, Y. Gogotsi, M. W. Barsoum, H. L. Zhuang and P. R. Kent, ACS Nano, 2014, 8, 9606–9615 CrossRef CAS PubMed.
- N. Thakur, P. Kumar, D. C. Sati, R. Neffati and P. Sharma, J. Energy Storage, 2022, 50, 104604 CrossRef.
- A. Deshmukh, M. Thripuranthaka, V. Chaturvedi, A. K. Das, V. Shelke and M. V. Shelke, Prog. Energy, 2022, 4, 042001 CrossRef.
- R. Yan, B. Mishra, M. Traxler, J. Roeser, N. Chaoui, B. Kumbhakar, J. Schmidt, S. Li, A. Thomas and P. Pachfule, Angew. Chem., Int. Ed., 2023, 62, e202302276 CrossRef CAS PubMed.
- L. Giebeler and J. Balach, Mater. Today Commun., 2021, 27, 102323 CrossRef CAS.
- Z. Pan, D. J. Brett, G. He and I. P. Parkin, Adv. Energy Mater., 2022, 12, 2103483 CrossRef CAS.
- J. He and A. Manthiram, Energy Storage Mater., 2019, 20, 55–70 CrossRef.
- L. L. Kong, L. Wang, Z. C. Ni, S. Liu, G. R. Li and X. P. Gao, Adv. Funct. Mater., 2019, 29, 1808756 CrossRef.
- Z. Shi, M. Liu, D. Naik and J. L. Gole, J. Power Sources, 2001, 92, 70–80 CrossRef CAS.
- T. Krauskopf, B. Mogwitz, C. Rosenbach, W. G. Zeier and J. Janek, Adv. Energy Mater., 2019, 9, 1902568 CrossRef CAS.
- R. Syamsai, J. R. Rodriguez, V. G. Pol, Q. Van Le, K. M. Batoo, S. F. Adil, S. Pandiaraj, M. R. Muthumareeswaran, E. H. Raslan and A. N. Grace, Sci. Rep., 2021, 11, 688 CrossRef CAS PubMed.
- A. S. Zeraati, S. A. Mirkhani, P. Sun, M. Naguib, P. V. Braun and U. Sundararaj, Nanoscale, 2021, 13, 3572–3580 RSC.
- D. Liu, L. Wang, Y. He, L. Liu, Z. Yang, B. Wang, Q. Xia, Q. Hu and A. Zhou, Energy Technol., 2021, 9, 2000753 CrossRef CAS.
- N. Shpigel, F. Malchik, M. D. Levi, B. Gavriel, G. Bergman, S. Tirosh, N. Leifer, G. Goobes, R. Cohen and M. Weitman, Energy Storage Mater., 2020, 32, 1–10 CrossRef.
- J. Meng, F. Zhang, L. Zhang, L. Liu, J. Chen, B. Yang and X. Yan, J. Energy Chem., 2020, 46, 256–263 CrossRef.
- M. Tao, G. Du, Y. Zhang, W. Gao, D. Liu, Y. Luo, J. Jiang, S. Bao and M. Xu, Chem. Eng. J., 2019, 369, 828–833 CrossRef CAS.
- X. Xu, Y. Zhang, H. Sun, J. Zhou, F. Yang, H. Li, H. Chen, Y. Chen, Z. Liu and Z. Qiu, Adv. Electron. Mater., 2021, 7, 2000967 CrossRef CAS.
- C. Liu, Y. Zhao, R. Yi, H. Wu, W. Yang, Y. Li, I. Mitrovic, S. Taylor, P. Chalker and R. Liu, Electrochim. Acta, 2020, 358, 136923 CrossRef CAS.
- W. Zhao, Y. Lei, Y. Zhu, Q. Wang, F. Zhang, X. Dong and H. N. Alshareef, Nano Energy, 2021, 86, 106120 CrossRef CAS.
- B. Yang, B. Liu, J. Chen, Y. Ding, Y. Sun, Y. Tang and X. Yan, Chem. Eng. J., 2022, 429, 132392 CrossRef CAS.
- Y. Wang, M. Zhou, L.-C. Xu, W. Zhao, R. Li, Z. Yang, R. Liu and X. Li, J. Power Sources, 2020, 451, 227791 CrossRef CAS.
- P. Liu, W. Liu and K. Liu, Carbon Energy, 2022, 4, 60–76 CrossRef CAS.
- C. Zheng, Y. Yao, X. Rui, Y. Feng, D. Yang, H. Pan and Y. Yu, Adv. Mater., 2022, 34, 2204988 CrossRef CAS PubMed.
- F. Liu, S. Jin, Q. Xia, A. Zhou and L.-Z. Fan, J. Energy Chem., 2021, 62, 220–242 CrossRef CAS.
- S. Gong, Y. Wang, P. Zhang, M. Li, Y. Wen, J. Qiu, B. Xu and H. Wang, Energy Fuels, 2023, 37, 3159–3165 CrossRef CAS.
- N. Zhao, Y. Yang, D. Yi, Y. Xiao, K. Wang, W. Cui and X. Wang, Chem. Eng. J., 2021, 422, 130018 CrossRef CAS.
- B. Kirubasankar, M. Narayanasamy, J. Yang, M. Han, W. Zhu, Y. Su, S. Angaiah and C. Yan, Appl. Surf. Sci., 2020, 534, 147644 CrossRef CAS.
- Z. Cao, Q. Zhu, S. Wang, D. Zhang, H. Chen, Z. Du, B. Li and S. Yang, Adv. Funct. Mater., 2020, 30, 1908075 CrossRef CAS.
- S. Sun, Z. Xie, Y. Yan and S. Wu, Chem. Eng. J., 2019, 366, 460–467 CrossRef CAS.
- Y. Feng, K. Wu, S. Wu, Y. Guo, M. He and M. Xue, ACS Appl. Mater. Interfaces, 2023, 15, 3077–3088 CrossRef CAS PubMed.
- J. Lv, H. Jia, G. Chen, Y. Wang, M. Liu, Y. Ning, Y. Wang, L. Yuan, M. Lu and J. Zhang, ACS Appl. Mater. Interfaces, 2022, 14, 46056–46067 CrossRef CAS PubMed.
- P. Lian, Y. Dong, Z.-S. Wu, S. Zheng, X. Wang, S. Wang, C. Sun, J. Qin, X. Shi and X. Bao, Nano Energy, 2017, 40, 1–8 CrossRef CAS.
- C. Yang, Y. Liu, X. Sun, Y. Zhang, L. Hou, Q. Zhang and C. Yuan, Electrochim. Acta, 2018, 271, 165–172 CrossRef CAS.
- T. Zhang, L. Pan, H. Tang, F. Du, Y. Guo, T. Qiu and J. Yang, J. Alloys Compd., 2017, 695, 818–826 CrossRef CAS.
- Q. Zhao, Q. Zhu, J. Miao, P. Zhang, P. Wan, L. He and B. Xu, Small, 2019, 15, 1904293 CrossRef CAS PubMed.
- S. J. Kim, M. Naguib, M. Zhao, C. Zhang, H.-T. Jung, M. W. Barsoum and Y. Gogotsi, Electrochim. Acta, 2015, 163, 246–251 CrossRef CAS.
- F. Du, H. Tang, L. Pan, T. Zhang, H. Lu, J. Xiong, J. Yang and C. J. Zhang, Electrochim. Acta, 2017, 235, 690–699 CrossRef CAS.
- F. Liu, J. Zhou, S. Wang, B. Wang, C. Shen, L. Wang, Q. Hu, Q. Huang and A. Zhou, J. Electrochem. Soc., 2017, 164, A709 CrossRef CAS.
- J. Zhou, S. Lin, Y. Huang, P. Tong, B. Zhao, X. Zhu and Y. Sun, Chem. Eng. J., 2019, 373, 203–212 CrossRef CAS.
- C. Xue, C. Yue and L. Yuan, J. Alloys Compd., 2021, 886, 161152 CrossRef CAS.
- G. Wang, J. Li, Z. Du, Z. Ma and G. Shao, Membranes, 2022, 12, 134 CrossRef CAS PubMed.
- D. K. Lee, C. W. Ahn and J. W. Lee, Electrochim. Acta, 2022, 402, 139539 CrossRef CAS.
- C. Xiong, G. Zhu, H. Jiang, Q. Chen and T. Zhao, Energy Storage Mater., 2020, 33, 147–157 CrossRef.
- S. Srinivasan, M. Jothibas and N. Nesakumar, Energy Fuels, 2022, 36, 4042–4051 CrossRef CAS.
- C. Wen, X. Zheng, X. Li, M. Yuan, H. Li and G. Sun, Chem. Eng. J., 2021, 409, 128102 CrossRef CAS.
- Y. Zhang, Z. Mu, C. Yang, Z. Xu, S. Zhang, X. Zhang, Y. Li, J. Lai, Z. Sun and Y. Yang, Adv. Funct. Mater., 2018, 28, 1707578 CrossRef.
- X. Chen, L. Li, Y. Shan, D. Zhou, W. Cui and Y. Zhao, J. Energy Chem., 2022, 70, 502–510 CrossRef CAS.
- X. Liang, A. Garsuch and L. F. Nazar, Angew. Chem., 2015, 127, 3979–3983 CrossRef.
- F. Zhang, Y. Zhou, Y. Zhang, D. Li and Z. Huang, Nanophotonics, 2020, 9, 2025–2032 CrossRef CAS.
- B. Guan, X. Sun, Y. Zhang, X. Wu, Y. Qiu, M. Wang, L. Fan and N. Zhang, Chin. Chem. Lett., 2021, 32, 2249–2253 CrossRef CAS.
- A. M. Abraham, T. Boteju, S. Ponnurangam and V. Thangadurai, Battery Energy, 2022, 1, 20220003 CrossRef CAS.
- Y. Li and S. Guo, Matter, 2021, 4, 1142–1188 CrossRef CAS.
- T. Li, C. He and W. Zhang, J. Energy Chem., 2021, 52, 121–129 CrossRef CAS.
- S. Huang, Z. Wang, Y. Von Lim, Y. Wang, Y. Li, D. Zhang and H. Y. Yang, Adv. Energy Mater., 2021, 11, 2003689 CrossRef CAS.
- M. Zhao, B.-Q. Li, X. Chen, J. Xie, H. Yuan and J.-Q. Huang, Chem, 2020, 6, 3297–3311 CAS.
- W. Wu, Y. Zhang, Y. Guo, J. Bai, C. Zhang, Z. Chen, Y. Liu and B. Xiao, Appl. Surf. Sci., 2020, 526, 146717 CrossRef CAS.
- W. Wang, L. Huai, S. Wu, J. Shan, J. Zhu, Z. Liu, L. Yue and Y. Li, ACS Nano, 2021, 15, 11619–11633 CrossRef CAS PubMed.
- W. Y. Lieu, C. Lin, X. L. Li, S. Jiang, Y. Li, H. Y. Yang and Z. W. Seh, Nano Lett., 2023, 23, 5762–5769 CrossRef CAS PubMed.
- T. Maihom, J. Sittiwong, M. Probst and J. Limtrakul, Phys. Chem. Chem. Phys., 2022, 24, 8604–8623 RSC.
- A. J. Y. Wong, W. Y. Lieu, H. Y. Yang and Z. W. Seh, J. Mater. Res., 2022, 37, 3890–3905 CrossRef CAS.
- Z. Yin, S. Pan, Q. Cheng, G. Zhang, X. Yu, Z. Pan, H. Rao and X. Zhong, J. Alloys Compd., 2020, 836, 155341 CrossRef CAS.
- D. Chen, Y. Mao, Y. Cao and W. Wang, Can. J. Chem. Eng., 2023, 101, 3719–3732 CrossRef CAS.
- L. Zhang, J. Bi, T. Liu, X. Chu, H. Lv, D. Mu, B. Wu and F. Wu, Energy Storage Mater., 2023, 54, 410–420 CrossRef.
- N. Li, W. Cao, Y. Liu, H. Ye and K. Han, Colloids Surf., A, 2019, 573, 128–136 CrossRef CAS.
- X. Gu and C. Lai, J. Mater. Res., 2018, 33, 16–31 CrossRef CAS.
- S. Grixti, S. Mukherjee and C. V. Singh, Energy Storage Mater., 2018, 13, 80–87 CrossRef.
- C. Du, J. Wu, P. Yang, S. Li, J. Xu and K. Song, Electrochim. Acta, 2019, 295, 1067–1074 CrossRef CAS.
- C. Wen, D. Guo, X. Zheng, H. Li and G. Sun, ACS Appl. Energy Mater., 2021, 4, 8231–8241 CrossRef CAS.
- Y. Huang, R. Field, Q. Chen, Y. Peng, M. S. Walczak, H. Zhao, G. Zhu, Z. Liu and L. Li, Commun. Chem., 2019, 2, 138 CrossRef CAS.
- N. K. Chaudhari, H. Jin, B. Kim, D. San Baek, S. H. Joo and K. Lee, J. Mater. Chem. A, 2017, 5, 24564–24579 RSC.
- Z. Chen, X. Yang, X. Qiao, N. Zhang, C. Zhang, Z. Ma and H. Wang, J. Phys. Chem. Lett., 2020, 11, 885–890 CrossRef CAS PubMed.
- L.-W. Lin, M. Qi, Z.-T. Bai, S.-X. Yan, Z.-Y. Sui, B.-H. Han and Y.-W. Liu, Appl. Surf. Sci., 2021, 555, 149717 CrossRef CAS.
- Y. Dong, S. Zheng, J. Qin, X. Zhao, H. Shi, X. Wang, J. Chen and Z.-S. Wu, ACS Nano, 2018, 12, 2381–2388 CrossRef CAS PubMed.
- Y. Zhao and J. Zhao, Appl. Surf. Sci., 2017, 412, 591–598 CrossRef CAS.
- A. Khan and M. Zohdy, J. Appl. Sci. Technol., 2023, 1, 01–09 Search PubMed.
- W. Y. Lieu, D. Fang, Y. Li, X. L. Li, C. Lin, A. Thakur, B. C. Wyatt, S. Sun, T. Ghosh and B. Anasori, Nano Lett., 2022, 22, 8679–8687 CrossRef CAS PubMed.
- M.-F. Ng, Y. Sun and Z. W. Seh, Energy Adv., 2023, 449–464 RSC.
- D. Chatterjee, D. Das, S. Sahoo, K. A. Raihan, K. Rajavel, S. R. Das and S. Majumder, Mater. Chem. Phys., 2023, 128583 Search PubMed.
- H. Zhang, Y. Zhang, L. Li, H. Zhou, M. Wang, L. Li, X. Geng, B. An and C. Sun, J. Colloid Interface Sci., 2023, 633, 432–440 CrossRef CAS PubMed.
- J.-Q. Huang, W. G. Chong, B. Zhang and X. Ma, Mater. Today Commun., 2021, 28, 102566 CrossRef CAS.
- S. Zhang, N. Zhong, X. Zhou, M. Zhang, X. Huang, X. Yang, R. Meng and X. Liang, Nano-Micro Lett., 2020, 12, 1–13 CrossRef PubMed.
- Y. Yao, S. Wang, X. Jia, J. Yang, Y. Li, J. Liao and H. Song, Carbon, 2022, 188, 533–542 CrossRef CAS.
- H. Li, F. Shao, X. Wen, Y. Ding, C. Zhou, Y. Zhang, H. Wei and N. Hu, Electrochim. Acta, 2021, 371, 137838 CrossRef CAS.
- H. Tang, W. Li, L. Pan, C. P. Cullen, Y. Liu, A. Pakdel, D. Long, J. Yang, N. McEvoy and G. S. Duesberg, Adv. Sci., 2018, 5, 1800502 CrossRef PubMed.
- D. Guo, F. Ming, H. Su, Y. Wu, W. Wahyudi, M. Li, M. N. Hedhili, G. Sheng, L.-J. Li and H. N. Alshareef, Nano Energy, 2019, 61, 478–485 CrossRef CAS.
- J. Zhao, Y. Qi, Q. Yang, T. Huang, H. Wang, Y. Wang, Y. Niu, Y. Liu, S. Bao and M. Xu, Chem. Eng. J., 2022, 429, 131997 CrossRef CAS.
- X. Geng, C. Liu, C. Zhao, Z. Jiang, E. G. Lim, Y. Wang, I. Mitrovic, L. Yang and P. Song, Electrochim. Acta, 2023, 441, 141877 CrossRef CAS.
- K. Liu, Y. Fan, A. Ali and P. K. Shen, Nanoscale, 2021, 13, 2963–2971 RSC.
- Z. Wang, N. Zhang, M. Yu, J. Liu, S. Wang and J. Qiu, J. Energy Chem., 2019, 37, 183–191 CrossRef.
- C. Wei, M. Tian, Z. Fan, L. Yu, Y. Song, X. Yang, Z. Shi, M. Wang, R. Yang and J. Sun, Energy Storage Mater., 2021, 41, 141–151 CrossRef.
- Y. Yao, W. Feng, M. Chen, X. Zhong, X. Wu, H. Zhang and Y. Yu, Small, 2018, 14, 1802516 CrossRef PubMed.
- J. Xia, W. Chen, Y. Yang, X. Guan, T. Yang, M. Xiao, S. Zhang, Y. Xing, X. Lu and G. Zhou, EcoMat, 2022, 4, e12183 CrossRef CAS.
- X. Liang, Y. Rangom, C. Y. Kwok, Q. Pang and L. F. Nazar, Adv. Mater., 2017, 29, 1603040 CrossRef PubMed.
- B. D. Adams, E. V. Carino, J. G. Connell, K. S. Han, R. Cao, J. Chen, J. Zheng, Q. Li, K. T. Mueller and W. A. Henderson, Nano Energy, 2017, 40, 607–617 CrossRef CAS.
- S. Kim, M. Cho and Y. Lee, Adv. Funct. Mater., 2020, 30, 1907680 CrossRef CAS.
- N. Wang, X. Zhang, Z. Ju, X. Yu, Y. Wang, Y. Du, Z. Bai, S. Dou and G. Yu, Nat. Commun., 2021, 12, 4519 CrossRef CAS PubMed.
- X. Li, Z. Chen, A. Li, Y. Yu, X. Chen and H. Song, ACS Appl. Mater. Interfaces, 2020, 12, 48718–48728 CrossRef CAS PubMed.
- X. Gao, Y. Zheng, J. Chang, H. Xu, Z. Hui, H. Dai, H. Wang, Z. Xia, J. Zhou and G. Sun, ACS Appl. Mater. Interfaces, 2022, 14, 15298–15306 CrossRef CAS PubMed.
- X. Zhang, T. Zhang, C. Zhang, J. Xiao, D. Wu, X. Ma and H. Gao, J. Alloys Compd., 2022, 909, 164730 CrossRef CAS.
- H. Aghamohammadi, R. Eslami-Farsani and E. Castillo-Martinez, J. Energy Storage, 2022, 47, 103572 CrossRef.
- Q. Zeng, S. Tian, G. Liu, H. Yang, X. Sun, D. Wang, J. Huang, D. Yan and S. Peng, ACS Appl. Mater. Interfaces, 2022, 14, 6958–6966 CrossRef CAS PubMed.
- J. Li, L. Han, Y. Li, J. Li, G. Zhu, X. Zhang, T. Lu and L. Pan, Chem. Eng. J., 2020, 380, 122590 CrossRef CAS.
- Q. Ma, Z. Zhang, P. Kou, D. Wang, Z. Wang, H. Sun, R. Zheng and Y. Liu, J. Colloid Interface Sci., 2022, 617, 147–155 CrossRef CAS PubMed.
- P. Das and Z.-S. Wu, J. Phys.: Energy, 2020, 2, 032004 CAS.
- T. Rasheed, J. Mater. Chem. A, 2022, 10, 4558–4584 RSC.
- H. A. Tariq, U. Nisar, J. J. Abraham, Z. Ahmad, S. AlQaradawi, R. Kahraman and R. Shakoor, Appl. Surf. Sci., 2022, 583, 152441 CrossRef.
- X. Zhao, Y. Zhang, Y. Hou, Z. Zhao, Y. Gong, Y. Wang, H. Wei and V. G. Pol, Adv. Compos. Hybrid Mater., 2022, 5, 2988–3001 CrossRef CAS.
- Y. Huang, H. Yang, Y. Zhang, Y. Zhang, Y. Wu, M. Tian, P. Chen, R. Trout, Y. Ma and T.-H. Wu, J. Mater. Chem. A, 2019, 7, 11250–11256 RSC.
- A. Sengupta, B. B. Rao, N. Sharma, S. Parmar, V. Chavan, S. K. Singh, S. Kale and S. Ogale, Nanoscale, 2020, 12, 8466–8476 RSC.
- Z. Zhang, H. Ying, P. Huang, S. Zhang, Z. Zhang, T. Yang and W.-Q. Han, Chem. Eng. J., 2023, 451, 138785 CrossRef CAS.
- Y. Wu, D. Liu, D. Qu, J. Li, Z. Xie, X. Zhang, H. Chen and H. Tang, Chem. Eng. J., 2022, 438, 135328 CrossRef CAS.
- X. Tang, D. Zhou, P. Li, X. Guo, B. Sun, H. Liu, K. Yan, Y. Gogotsi and G. Wang, Adv. Mater., 2020, 32, 1906739 CrossRef CAS PubMed.
- T. Ghaed-Sharaf and A. Omidvar, Phys. Chem. Chem. Phys., 2022, 24, 13988–13998 RSC.
- M. Zhang, R. Liang, N. Yang, R. Gao, Y. Zheng, Y. P. Deng, Y. Hu, A. Yu and Z. Chen, Adv. Energy Mater., 2022, 12, 2102493 CrossRef CAS.
- S. Nyamdelger, T. Ochirkhuyag, D. Sangaa and D. Odkhuu, Phys. Chem. Chem. Phys., 2020, 22, 5807–5818 RSC.
- E. Cha, J. H. Yun and D. K. Kim, APL Mater., 2022, 10 DOI:10.1063/5.0070013.
- H. G. Ali, K. Khan, M. B. Hanif, M. Z. Khan, I. Hussain, M. S. Javed, H. A. AL-bonsrulah, M. Mosiałek, M. Fichtner and M. Motola, J. Energy Storage, 2023, 73, 108980 CrossRef.
- Z.-L. Xu, J.-K. Kim and K. Kang, Nano Today, 2018, 19, 84–107 CrossRef CAS.
|
This journal is © The Royal Society of Chemistry 2024 |