DOI:
10.1039/D0QI01153C
(Review Article)
Inorg. Chem. Front., 2021,
8, 201-233
Luminescence response mode and chemical sensing mechanism for lanthanide-functionalized metal–organic framework hybrids
Received
24th September 2020
, Accepted 21st October 2020
First published on 22nd October 2020
Abstract
The luminescence response application in chemical sensing by metal–organic frameworks (MOFs) and their hybrid materials has been reported extensively in recent years. However, the reviews on this topic are mainly focused on the sensing materials (MOFs and their functionalized hybrid materials) and analytes (all types of species). In this comprehensive review, the luminescence response mode and chemical sensing mechanism for lanthanide-functionalized MOF hybrids (abbreviated as LnFMOFH) are systematically summarized. Specifically, ten sections are subdivided, and importantly involve three main topics. Firstly, LnFMOFH and the luminescence responsive chemical sensing for these hybrids are introduced. Secondly, three single luminescence response modes, including “Turn-Off”, “Turn-On” “Turn–Off–On” and dual mode, as ratiometric luminescence (RL) for chemical sensing in LnFMOFH materials are summarized. Thirdly, some types of chemical sensing mechanisms observed in LnFMOFH materials are outlined, which involve the ligand–metal energy transfer (LMET) for the luminescence response in chemical sensing of enhanced or weakened analytes; the fluorescence (Förster) resonance energy transfer (FRET) and photo-induced electronic transfer (PET) mechanism depending on the energy match between the probe and analyte; and some special chemical interactions for their luminescence response upon chemical sensing, such as hydrogen bonding interaction, coordination interaction and other reactions. Finally, the conclusion and prospects are given for this topic. It needs to be noted that pure lanthanide MOFs (lanthanide ions as the framework metal, including mixed lanthanide MOFs) are not the topic of this review and are only mentioned for background knowledge. Herein, LnFMOFH materials in which the lanthanide ions behave only as photoactive species to functionalize MOF units via post-synthetic modification (PSM) by researchers are the emphasis of this review.
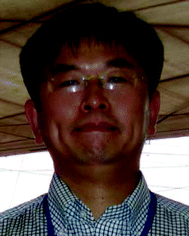 Bing Yan | Dr Bing Yan received his BSc and MSc in Applied Chemistry from the Harbin Institute of Technology in 1992 and 1995, respectively. Then he studied at the Changchun Institute of Applied Chemistry, where he obtained his Ph.D. in Inorganic Chemistry in 1998. In 1998–2001, he was a Research Assistant at City University of Hong Kong and then became Postdoctoral Fellow at Peking University and University of Sherebrook. At the end of 2001, he joined Tongji University as a Professor. His main research interests are focused on rare earth chemistry, hybrid materials, micro-nano solids, photophysical devices and photo-responsive chemical sensing. To date, as the corresponding author, he has published over 500 scientific papers in peer review journals, which have been cited more than 10 000 times in international journals. |
1. Introduction on lanthanide-functionalized MOF hybrids (LnFMOFH)
Metal–organic frameworks (MOFs, also known as porous coordination polymers or PCPs) are a class of porous polymeric molecular materials consisting of metal ions linked together by organic bridging ligands, which belong to a new class of development for the interdiscipline between coordination chemistry and functional materials.1 Due to their structural and functional tunability, the area of MOFs has become one of the fastest growing fields in inorganic chemistry. It mainly involves five categories: (1) synthesis of metal-containing nodes or coordination bonds, (2) linker design and its post-synthetic modification (PSM), (3) symmetry-guided synthesis and structural characterization of MOFs, (4) MOF hybrids or composite research and (5) potential application of MOFs.2 The foundation of MOF chemistry is that the frameworks are assembled by linking molecular units of well-defined shapes by chemical bonds into periodic frameworks.3 An important component of reticular chemistry is the deconstruction of these structures into their underlying nets to facilitate the designed synthesis of materials with targeted porosity, pore size, and functionality.4 Organic ligands endow flexibility and diversity to their chemical structures and functions.5 The synthesis of MOFs has attracted immense attention due to the possibility of obtaining a large variety of aesthetically interesting structures for extensive applications related to porous materials. This includes traditional areas of storage, separation, and catalysis, which are based on the pore size and shape and host–guest interactions. In addition, sensing and biomedical applications and their use as sensor materials are currently intensively investigated.6,7
Lanthanide MOFs (LnMOFs, here means lanthanide ions behave as unique framework metal ions) have attracted much interest due to their wide array of features from the combination of lanthanide ions (Ln3+) with traditional MOFs. Different from transition metals ions, the electrons in the f block of Ln3+ make them capable of having a larger coordination sphere. Based on the hard–soft acid–base theory,8 Ln3+ ions have affinity with relatively hard oxygen-containing linkers compared to other functional groups. Ln3+ display a high coordination number, connectivity, and diverse coordination modes, and therefore exhibit great potential in constructing a wide variety of MOFs. However, compared to traditional transition metal MOFs, LnMOFs have been significantly less studied. The highly connective lanthanides usually lead to the formation of condensed frameworks in the microporous range. Certainly, as other MOF systems, LnMOFs also have practical application value in the fields of separation, catalysis and especially as a very promising class of materials for addressing the challenges in the engineering of luminescent centres.9,10 Lanthanide-bearing phosphors have numerous applications in lighting, optical communications, photonics, and biomedical devices.11 Besides, it should be emphasized that the abundant luminescence response of LnMOFs embody their versatile application in chemical sensing.12,13
Lanthanide-functionalized MOF hybrids (LnFMOFH) mean that some special photoactive Ln3+ ion (such as visible emissive Eu3+, Tb3+, Sm3+ and Dy3+ and near infrared (NIR) emissive Nd3+, Yb3+ and Er3+) are introduced into the MOF host for the functionalization of the MOF units and formation of hybrid material systems. For example, the most common approach to assemble LnFMOFH materials is PSM of MOFs.14 There are a variety of ways to modify an MOF in a post-synthetic manner, and each of these modification forms has the capacity to alter the physical and chemical properties of the framework. The PSM of MOFs was broadly reviewed by Cohen and colleagues.15 For LnFMOFH materials, the effective PSM strategies can be divided into main six areas (Fig. 1) as follows: (a) coordinate PSM, (b) covalent PSM, (c) covalent and coordinate PSM, (d) ion exchange PSM, (e) ion doping (post-synthetic metalation or metal-ion substitution PSM), and (f) encapsulation composition PSM. These post-synthetic methods provide high complexity and functionality for LnFMOFH materials and the desired chemical transformation should not destroy the MOFs under the employed reaction conditions. Subsequently, some new hybrid materials based on Ln3+ and MOFs can be obtained by PSM methods, which still retain the characteristic features of MOFs such as high crystallinity, high surface areas, and a structure comprising highly regular coordination bonding. Among them, coordinate PSM is the first effective approach to introduce Ln3+ ions to achieve the final hybrid materials,16 which uses Ln3+ species to coordinate to the free active groups in MOFs (Fig. 1a). Covalent PSM involves the use of a reagent to modify a component of the MOF in a heterogeneous, post-synthetic manner to form a new covalent bond, providing a powerful and versatile method to introduce a broad range of chemical groups into MOFs (Fig. 1b). However for LnFMOFH materials, considering that Ln3+ is introduced through coordinate PSM, generally covalent PSM is the procedure employed to produce coordinated groups for further Ln3+ coordinate PSM (Fig. 1c). It should be noted that the Ln3+ ion coordination often destroys covalent PSM-derived MOFs, and thus it is very important to consider their stability.17 Besides, covalent-coordinate PSM can be expected to be further employed to construct numerous LnFMOFH materials.18 Ion exchange PSM is mainly appropriate for anionic MOF systems with dimethylammonium (DMA) cations in their porous structure, and thus Ln3+ cations19 can be introduced into the MOF via ionic exchange with DMA (Fig. 1d). Ion doping PSM is easily realized for LnMOFs with a similar ionic radius due to lanthanide contraction.20 In fact, Ln3+ ionic doping can also be extended to other trivalent metal ion MOFs such as Al, Ga, In ions for the same valence substitution (Fig. 1e). In addition, encapsulation composition PSM permits all types of lanthanide compounds (salts, complexes, oxides, etc.) as small guest molecules or nanoparticles (NPs) to be introduced in the MOF host,21 which physically keep the whole luminescent hybrid system stable (Fig. 1f). It is worth noting that the content of the functionalized luminescent Ln3+ in the MOF hosts is limited and small, but it still displays effective luminescence for practical applications.
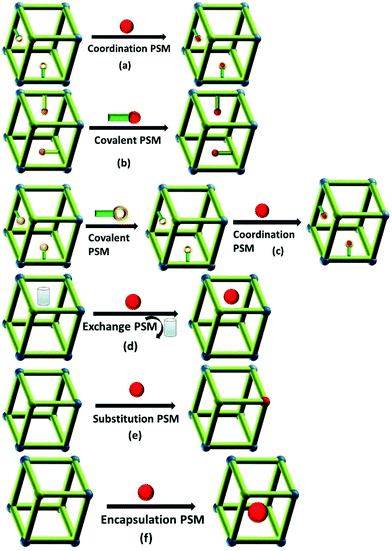 |
| Fig. 1 Scheme for post-synthetic modification (PSM) of MOFs by lanthanide (Ln3+) ions for the corresponding hybrid materials (LnFMOFH), where red sphere represents the lanthanide species used for functionalized MOFs. | |
In this review, the focus is on the luminescence response mode and chemical sensing mechanism of LnFMOFH materials. Specifically, ten sections are subdivided and four main topics are mainly discussed. Initially, LnFMOFH materials and the luminescence responsive chemical sensing using these hybrids are introduced. Secondly, three single luminescence response modes for chemical sensing in LnFMOFH materials are summarized, including “Turn-Off”, “Turn-On”, and “Turn-On–Off–On”, together with another dual luminescence response mode, ratiometric luminescence (RL). Thirdly, some types of chemical sensing mechanisms observed in LnFMOFH materials are outlined, which involve ligand–metal energy transfer (LMET) for the luminescence response upon chemical sensing of enhanced or weakened analytes; the fluorescence (Förster) resonance energy transfer (FRET) and photo-induced electronic transfer (PET) mechanism depending on the energy match between the probe and analyte; and some special chemical interactions for the luminescence response upon chemical sensing, such as hydrogen bonding interaction and coordination interaction and some reaction processes. Finally, the conclusion and prospects are presented for this topic. It needs to be noted that pure lanthanide MOFs (Ln3+ ions as the framework metal ion, including mixed lanthanide MOFs) are not the topic of focus in this review, which will be merely mentioned for introducing background knowledge. The LnFMOFH materials (Ln3+ ions as the only photoactive species with limited concentration to functionalize MOF units by PSM) developed by researchers is the emphasis of this review. This is based on most of the works by my group in recent years.
2. Lanthanide-functionalized MOF hybrids (LnFMOFH) for luminescence response and chemical sensing
2.1 Luminescence response of MOFs, LnMOFs and lanthanide-functionalized MOF hybrids (LnFMOFH)
A series of novel MOF materials have been developed as platforms for photo-functional materials due to the intrinsic optical and photonic properties of the metal ions, organic ligands, and guest species collaboratively assembled and/or encapsulated in their frameworks.22 A scheme of the luminescence possibilities in a porous MOF material is shown in Fig. 2. MOF materials with well-defined spatial arrangements of lumophores can give rise to a number of interesting luminescence performances, mainly involving metal-centered luminescence (MC), ligand-centered luminescence (LC), metal-to-ligand charger transfer (MLCT), ligand-to-metal charge transfer (LMCT), ligand–ligand charge transfer (LLCT), and metal-to-metal charge transfer (MMCT). Besides, the encapsulated guest may produce guest-induced emission and the functionalization of MOFs may lead to a change in the luminescence of the MOF. The resulting emission depends on the nature of the MOF structure, such as the spacing and orientation between linkers, the HOMO–LUMO gap of the linkers versus the accessible states of the metal units, the electronic configuration of the metal ions and the bonding geometry.23 Luminescence from MOFs containing transition metal ions in their frameworks is typically centered on the linker, but can also involve the disturbance of the metal ion in the linkers or even all types of charge transfer (MLCT, LMCT, LLCT and MMCT) between the metal ions and linker.24 Paramagnetic transition metal complexes usually show weak emission, and their partially filled d-orbitals produce d–d ligand-field transitions, which may give rise to strong reabsorption and/or quenching of fluorescence of organic molecules via electron or energy transfer. MOFs of ds partition metal ions without unpaired electrons can exhibit LC luminescence. Firstly, the most common and important mechanism is LC luminescence from the luminescent groups of ligands, typically allowing conjugated organic compounds to absorb in the UV and visible region. Besides, the interaction between different units in MOFs may produce abundant CT luminescence. LLCT emission covers luminescence from orbitals with contributions from multiple ligand molecules or luminescence based on energy transfer from an absorber ligand to an emitter ligand. Typically, the aromatic ligands of MOFs make their frameworks exhibit ligand stacking, which display orbital mixing between ligand molecules due to their π–π interactions. LMCT emission can either result from orbital mixing between metal ions and ligand molecules or from absorbance on ligands followed by energy transfer onto the metal ion, where emission occurs. In LMCT luminescence through orbital mixing, the LUMO is primarily based on the orbitals from the metal ion, with the HOMO primarily composed of ligand orbitals. Transitions between mixed metal/ligand orbitals are responsible for luminescence, with the majority of the HOMO being contributed by metal orbitals, and the majority of the LUMO being contributed by ligand orbitals. Secondly, MC luminescence is most often observed in MOF materials with framework metal or doping f partition (actinide and especially lanthanide) ions, which will be discussed in-depth later.25 Thirdly, other guest species introduced into the MOF host produce luminescence (so-called GI (guest-induced) emission). This involves three aspects as follows: (1) “adsorbed lumophores” through trapping luminescent molecules or NPs in another non-emissive MOF. (2) “Exciplex formation” through π–π interactions between adjacent conjugated linkers or between a linker and a guest molecule to produce an excited complex, which typically exhibits broad, featureless luminescence, and (3) “lumophores bound to the MOF surface” to create multifunctional MOFs.22 Host–guest CT depends primarily on the nature of the guest species. Dyes with conjugated or aromatic moieties can interact with the aromatic ligands of the framework through π–π interactions, resulting in Dexter electronic exchange (DEE) energy transfer mechanisms, which can transfer excitation energy between the host framework and guest species. Moreover, it is worth noting that the functionalization of MOFs through versatile PSM methods may have an influence on the luminescence of MOF systems, so-called FI (functionalization-induced) emission change, which needs to be emphasized.
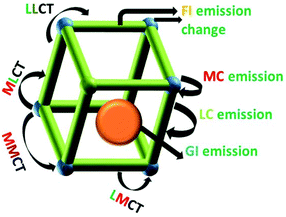 |
| Fig. 2 Representation of the emission possibilities in a porous MOF: MC (metal-centered luminescence), LC (ligand-centered luminescence), MLCT (metal-to-ligand charger transfer), LMCT (ligand-to-metal charge transfer), LLCT (ligand–ligand charge transfer), MMCT (metal-to-metal charge transfer), GI (guest-induced) emission, and FI (functionalization-induced) emission change. The metal centers (blue octahedra) are linked by organic ligands (orange rectangles) with an incorporated guest (red circle). | |
As shown in Fig. 3 (left side) for LnMOF or LnFMOFH materials, molecular photoluminescence can be broadly characterized as fluorescence or phosphorescence. In fluorescence, an electron is excited into some singlet excited state (Sn) and quickly relaxes into the lowest singlet excited singlet state (S1), which is called internal conversion (IC). Emission then occurs when the electron makes the spin-allowed transition from S1 to the ground state (S0). In phosphorescence, an electron is again excited into some singlet state (Sn) and relaxes into the lowest excited singlet state S1; however, it then undergoes intersystem crossing (ISC) to the triplet excited state (T1). Emission is associated with the spin-forbidden transition from T1 to the ground state (S0). For LnMOFs, molecules behave as ligands or linkers to be coordinated to Ln3+. Consequently, by coordinating these Ln3+ cations with conjugated organic ligands capable of transferring energy to Ln3+ through the “antenna effect” after absorbing light energy from the environment, Ln3+ ions can be sensitized and in turn exhibit intense luminescence. Thus, based on the abovementioned points, it can be inferred that by selecting appropriate Ln3+ as well as suitable organic ligands/linkers, desirable MOFs showing excellent luminescent properties can be constructed. Here, Ln3+ served as the central framework metal ion, and thus the luminescence performance depends on the type of Ln3+ ion.26 For inert Ln3+ ions such as La3+, Gd3+, Lu3+ and Y3+, their close shell electronic configurations make their MOF materials display the luminescence of the ligands or linkers. In contrast, for other Ln3+ ions, the “antenna effect” appears between the T1 of the ligands or linkers to the resonant emissive energy levels of the photoactive Ln3+ ions (especially Eu3+ and Tb3+).27 The effective intramolecular energy transfer process within LnMOFs may achieve the strong emission of Ln3+, and its efficiency is generally discussed using the DEE process. This involves quenching of donor fluorescence by transmission of the excited electron of the donor molecule to acceptor molecule via a nonradiative pathway, which his applicable for short range interactions operating between donor and acceptor (typically within 10 Å) by their orbital overlap. Although the luminescence of LnMOFs depends on the energy match between their ligands and Ln3+, when the energy match is suitable for the sensitization of Ln3+, generally the LnMOFs will mainly exhibit the luminescence of Ln3+ because it is the framework ion with a relatively high content.28 On the contrary, for the situation of no energy match between them, the linker luminescence is displayed instead of the Ln3+ luminescence (Fig. 3). Indeed, a high content photoactive Ln3+ in LnMOFs limits their practical application due to their high cost.
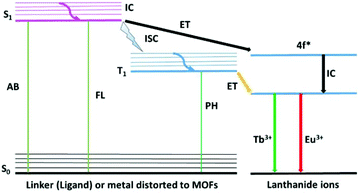 |
| Fig. 3 Schematic representation of energy absorption, migration, emission, and processes in LnMOFs and lanthanide-functionalized MOF hybrids (LnFMOFH). Abbreviations: A = absorption; FL = fluorescence; PH = phosphorescence; IC = internal conversion; ISC = intersystem crossing; ET = energy transfer; IC = internal conversion; S = singlet; and T = triplet. Plain arrows indicate radiative transitions and curve arrows indicate nonradiative transitions. | |
For LnFMOFH materials, luminescent Ln3+ ions are incorporated into the MOF host with a small content and the characteristic emission of Ln3+ is obtained.29 This is identical to the traditional lanthanide-doped phosphors. Similar to pure luminescent LnMOFs, Ln3+ can produce an “antenna effect”, resulting in a pronounced increase in the luminescence intensity through the intramolecular energy transfer process from the linkers to Ln3+. Ln3+ ions show weak absorbance and low quantum yields due to their forbidden electronic transitions, and thus a strongly photo-absorbing linker is introduced to circumvent this problem. In most cases, the important energy transfer occurs from the linker T1 to the Ln3+ resonant emissive state. Thus, it is significant to choose a linker for efficient intersystem crossing within the linker. The energy gap between the linker triplet state and the emitting Ln excited state plays an important role in the transfer of energy from the antenna.30 In addition, it should to be noted that a relative limited content of Ln3+ in LnFMOFH materials often allows the existence of luminescence from the original linkers or MOFs themselves if the functionalized amount of Ln3+ is controlled appropriately. This makes it possible to show multiple center luminescence for the same hybrid system and even realize luminescence color tuning or white luminescence integration.
The interest in multi-color-emitting materials, especially white light-emitting materials, is due to their wide applications in panel displays, room lighting and other optical devices.31 The collaborative properties of the multiple luminescent centers in MOFs are particularly useful to develop multi-color and white-light-emitting materials. Common approaches to realize white light integration in MOFs include: (a) use of monochromatic emitters covering the entire visible spectrum, (b) dichromatic emitters blending blue and yellow or orange light, and (c) trichromatic emitters combining three primary colored (red, green, and blue) components.32 In addition, the regulation and integration of emission colors of luminescent MOFs can also be readily implemented by modulating both chemical factors (such as Ln3+ type and concentration) and physical parameters such as excitation wavelength. Thus far, tremendous efforts have been dedicated to the synthesis of MOFs with tunable luminescence or white light.33 According to the luminescence character of the above main three aspects, these luminescent species show different spectral bands in the visible region. For example, linker-based MOF luminescence is often situated in the short visible region, covering visible to blue to green even covering the wide bands of yellow color. However, the commonly used lanthanide system is Eu3+-based MOFs, whose luminescence is mainly in the red long wavelength region. Thus, it can be expected that luminescent tuning and even white luminescence integration can be realized by adjusting the luminescence center in MOF-based hybrid material systems. This is very useful for display and lighting devices or barcoding.34 Although luminescent MOF-based OLED devices have not been realized, it is theoretically feasible to incorporate nanoscale electroluminescence MOFs between two conductors to achieve these useful electronic devices. Nanoscale luminescent LnFMOFH materials will be expected to be fabricated into thin films for straightforward and instant sensing devices in the future.
2.2 Luminescence response for chemical sensing of lanthanide-functionalized MOF hybrids (LnFMOFH)
Luminescent sensors utilize UV-light to produce a glow in the analyte in the visible region of the spectrum. This excited light produces a signal, which is visible to the naked eye. On the other hand, chemodosimeters offer irreversible recognition of the analyte, providing high analyte selectivity. This recognition process is less affected by external factors. Both MOFs and their lanthanide-functionalized hybrid materials are potential candidates for luminescent sensing of all types of analytes.
Luminescence response and chemical sensing in MOF-based materials.
For an MOF-based material to serve as a useful sensor, it needs possess several properties as follows: (1) it must exhibit a luminescence response (intensity or wavelength) to an interaction with an analyte (“Turn-On” for enhancement or “Turn-Off” for quenching); (2) this response must have some analyte specificity (selectivity for sensing); (3) optimally, the response should be reversible; and (4) the response must be detectable (acceptable limit of detection (LOD)). Detectability makes luminescence more attractive than light absorption since it is more sensitive and even small numbers of photons can be detected using photon counting techniques, particularly in the absence of a large background signal.35 Several potential transduction mechanisms are possible in fluorescent MOFs as follows: (1) solvochromatic shifts, (2) alteration in the electronic structure via changes in the coordination sphere, (3) fluorescence quenching by adsorbed species, and (4) exciplex formation. MOFs with widely varied fluorometric sensing properties have been developed by the design principles of crystal engineering, structure–property correlations, and functionalization, resulting in a large and rapidly growing body of interests. Sensing research involves a number of crucial sensing species.36 Furthermore, new classes of MOF sensory materials utilizing advanced signal transduction by devices based on MOF photonic crystals and thin films have been developed.37
MOF-based materials primarily display special advantages for chemical sensing. Firstly, their high surface area and structural stability afford abundant interactions with guest species and endow them with high sensitivity, which is crucial for efficient sensor development. Secondly, their versatile pore or channel structure and functional position provide them with high selectivity. Benefiting from the porosity of MOFs, various analytes can be selectively recognized through size exclusion, where the orifices of MOFs act as a sieve to permit a specific moiety to enter the cavity present inside MOFs. The entering moiety can easily change the electronic structure of the MOF by developing a coordination with the active sites (acceptor) present in the MOF structure, consequently changing the photophysical behavior of the MOF. Various functionalities can serve as binding sites for MOFs including ions, and Lewis acidic/basic sites can serve as receptors for targeting specific analytes. Further efforts should focus on the construction of porous luminescent MOFs with multifunctional sites to collaboratively induce their preferential and highly sensitive and selective binding with different species. Furthermore, the microporous structure can act as a molecular sieve by blocking oversized molecules, and hence increasing the sensitivity and selectivity of the detection system. If luminescence sensing can be combined with the sieving function of the micropores to allow small molecules to go through, while excluding larger species, it is possible to obtain some highly sensitive sensors. Thirdly, their crystalline framework structure and energy transfer process are beneficial to understand the sensing mechanism. Compared to other porous amorphous materials, the distinct crystalline assemblies of MOF-assisted detectors permit the exploration of the detecting principle of the frameworks based on MOFs at the molecular level. Moreover, the exceedingly tunable nature of MOF structures guarantees the optimization and specific design of MOF-assisted sensors. Fourthly, their multiple luminescent centres (especially for LnFMOFH materials) have potential applications in ratiometric or even intelligent sensing for complicated systems. Different metal ions, mixed ligands or guests having diverse luminescent properties can be hosted in the MOF structure with the aim of realizing self-calibration in the detection systems.38 As sensing materials, MOFs can be applied in the engineering of next-generation MOF-based sensing devices, which rely on the combination of MOF light-harvesting ability, understanding the energy transfer processes within a framework, and application of MOF-based function (catalysis, adsorption, etc.) for sensing enhancement. This approach will certainly initiate extensive research endeavors not only on light-emitting MOFs, but also MOF sensor devices due to the combination of “Turn-On” emission and multicolor luminescence. Fifthly, linkers with carefully designed functionalities can be used as supports for MOFs, resulting in molecular specificity in the host–guest interactions. On the other hand, the detection ability of MOF-based sensors can also be increased by PSM.39
Ion species sensing.
Ionic species are relatively limited to cations and anions in practical fields. For cation sensing, it mainly focuses on the metal ions in aqueous solution systems. Generally, it involves three types of metal ions. The first type plays an important role in living systems, such as Fe3+, Fe2+, Cu2+ and Zn2+. The sensing of Fe3+ is universal and there are numerous probes based on LnFMOFH systems. A luminescence probe to detect Fe3+ in environmental and biological systems mainly depends on the quenching effect on the luminescence of LnFMOFH by metal cations, which originates from three approaches, i.e. the interaction between metal cations and ligands, the collapse of the crystal structure by metal cations, and the cation exchange of the central cations in the framework with targeted cations.39,40 The sensing of Fe2+ and even both Fe2+ and Fe3+ or Fe2+ against Fe3+ is expected to be studied in-depth.40a The research by Yan’ group on probes for the luminescent sensing of Cu2+ by LnFMOFH focused on luminescence quenching.41 Firstly, they used pyrene covalently PSM-functionalized MOFs to change the emission by space limitations within the MOF structure to detect Cu2+ ions.41a Then, they constructed heterostructure microsphere chemosensors using s lanthanide complex and MOFs to cover silica for the detection of Cu2+ in aqueous solution.41b Further, they achieved an Eu3+ PSM MOF conjugated with fluorescein-labelled single-stranded DNA for the detection of Cu2+.41c The second type of species given attention is heavy metal ions, which are harmful to the environment and human health. Among them, cadmium (Cd2+) and mercury (Hg2+) are the most dangerous ions due to their high toxicity and carcinogenicity. The qualitative and quantitative detection of Cd2+ and Hg2+ ions can be considered to be an aspiration of primary importance.42,43 For Cd2+, all types of LnFMOFH materials show a “Turn-On” luminescence response and Cd2+ behaves as a heavy metal ion to enhance the absorption of the linker to transfer effective energy to Ln3+.42 In contrast, Hg2+ shows the “Turn-Off” luminescence quenching effect for lanthanide hybrids due to its opposite influence on the energy transfer process.43 Besides, there are reports on the sensing of other metal ion species using lanthanide hybrids, involving Ag+, Al3+, and Cr3+, which display both “Turn-On” and “Turn-Off” responses for the different control of the energy migration within the whole hybrid system.44,45
For anions, reports on their sensing using LnFMOFH materials are scarce. There are two typical types of anions, the complicated oxysalt group ions and simple halogen ions or pseudohalogen ions. Among the oxysalt group anions, Cr(VI) species, nitrite ions, and phosphate ions are important. Among the common toxic anion pollutants, Cr(VI) species, i.e., CrO42− and Cr2O72−, are the most important, which show an apparent luminescence quenching effect on lanthanide hybrids. This can be ascribed to the interaction of CrO42−/Cr2O72− with Ln3+, which interferes with the efficiency of the antenna effect via a static quenching mechanism.46 In addition, for the nitrite ion, NO2−, which is used a food preservative, its content is of practical significance due to its physiological effect in drinking water or daily foods. Yan's group designed two types of LnFMOFH probes for the detection of NO2−, both of which exhibited luminescence quenching via different mechanisms (LMCT and DEE).47 In addition, some works employed LnFMOFH probes for the sensing of PO43− in simulated biological fluids or water medium and real environment water through a luminescence quenching response.48a,b Besides, Sun et al. reported the ratiometric detection of CO32− based on heterobimetallic Eu/Pt MOFs.48c The simple single atomic anion sensing is popular for F−, where a Tb3+-functionalized ZrMOF hybrid probe showed the “Turn-On” response and exhibited the Lewis acid–base interaction mechanism, different from the common hydrogen bonding effect.48d
Molecule sensing.
Compared to ion species, molecule species are abundant and complicated, involving inorganic and organic molecules. The most common molecules are organic chemicals and pollutants, which are attracting increasing attention due to their environmental and biological threat. In summary, four types of important molecular species are investigated.49–57 Firstly, organic chemicals, which are mostly volatile organic solvents (especially organic amine or benzene). They are utilized in laboratories for practical scientific research and industrial production. However, their precise sensing based on a luminescence response is very difficult. Nowadays, the main focus is limited to some organic solvent molecules, such as acetone, pyridine, tetrahydrofuran, trichloromethane, organic amine and organic aromatics.49 Both volatile organic solvents and their vapors can be detected in the solution and vapor state. Among them, atmospheric benzene homologues (BTEX) are noted for their extensive use. For volatile organic compounds (VOCs) with a similar composition and structure or properties, the unprecedented dual-readout identification scheme can be established.50 Secondly, inorganic or organic pollutants, which have been studied extensively due to their importance in the environmental field.51 Among them, inorganic pollutants such as amine gas and organic pollutants such as formaldehyde are the most popular due to their presence in public and confined spaces. Besides, the special organic or polymeric chemicals used in industrial applications are also worth noting, which is important for the health of workers. However, the direct detection of these chemical products cannot exactly reflect their actual harmfulness, and thus their metabolites tend to be detected. This will be discussed subsequently for biomarkers. Thirdly, some special important types of molecular species in some unique fields have also attracted attention from researchers.52–54 For example, nitroaromatic explosives (NAE) have attracted the most attention due to their serious threat to public safety. The extensive use of explosives for military purposes has also raised concerns about environmental contamination where they are produced and stored. On the other hand, various chemical warfare agents (CWAs), including sarin, chlorine, and mustard gas, are used, which remain a significant global threat. Furthermore, medicines such as antibiotics and pesticides are important molecule species for sensing because they are highly toxic organic compounds and play an extremely crucial role in the medical and agricultural fields. For example, the luminescence-responsive sensing by MOF probes for nitroaromatic explosives, CWAs, drugs and pesticides has been explored,52–55 but there are no reports on LnFMOFH materials to date. Therefore, there is great potential for research in this direction. Fourthly, biomarkers including metabolites. Biomarkers are indices for biological monitoring, which can be considered as a tool to investigate the relationship between exposure to external chemicals and health effects. As incipient biological signals to indicate the toxicological effect of pollutants, biomarkers have been developed and applied in several disciplines. Yan's group performed extensive works on LnFMOFH materials for sensing biomarkers.56,57
Other sensing.
The most extensive sensing research is on thermometers for temperature sensing. Thermographic phosphor thermometry is a non-invasive spectroscopic technique for temperature measurement based on the temperature dependence of the phosphor emission. In the last decade, many Ln3+-based thermometers have been reported, covering a wide temperature range, from cryogenic (T < 100 K) to physiological (298–323 K) values. Among them, MOF-based materials and their multifunctional heater-thermometer nanoplatforms have been employed. The mixed-lanthanide (Ln and Ln′) approach, which is generally based on the dual-emission of Tb3+ and Eu3+ within MOFs, has been used to construct ratiometric thermometers. These materials involve three energy transfer process, namely Tb3+-to-Eu3+, ligand-to-Eu3+, and ligand-to-Tb3+, and display the characteristic transition emission of the Eu3+ and Tb3+ ions. Although most works on MOF-based hybrid thermometry are based on the Tb3+/Eu3+ emission ratio, a few reports explored the emission of an organic chromophore (usually involving an excited triplet state) and Ln3+. The implementation of LnMOF or LnFMOFH materials as ratiometric thermometers in diverse applications has been extensively reviewed in the past decade.58,59 Another important sensing application is focused on the pH value of aqueous media and further utilization. The study of pH changes in living cells is important for revealing the mechanism of cell metabolism and different physiological and pathological processes, as well as the intracellular drug delivery process. pH detection is very important and interesting in luminescence sensing research; however, there are few reports on the use of MOF materials as fluorescent pH sensors because most MOFs cannot resist harsh acid and alkali solutions. Among them, MOFs with pH-dependent fluorescent ligands or pH sensitive fluorescent dyes can be used as pH sensors. Some lanthanide MOFs have been reported for sensing pH value, where their luminescence intensity responds to a chance in pH. This is strongly correlated with the protonation level of the linkers and the ionization constant.60 For LnFMOFH materials as sensors for pH, Lu et al. design a new ratiometric pH sensor based on PSM nanoscale MOFs, which does not require external calibration for the pH range of 5.0–7.2, corresponding to the common pH range of biological fluid. Then, Zhang et al. develop a pH fluorescent probe, which could to distinguish alkaline or even neutral solutions by its fluorescence intensity well. Liu et al. further achieved a broad-range (1.05 to 9.85) pH sensor via the PSM of MOFs.61
Previously, the most widely observed sensing mechanism for analyte detection is electron transfer through the host–guest interactions of the analyte and receptor molecule. This is because of the presence of the lowest unoccupied molecular orbitals (LUMOs) of most analytes at a lower energy level than that of MOFs, making the electron transfer possible from the MOF to the guest molecule. Another important factor affecting the sensing behavior is the transfer of energy between the analyte and MOF (host–guest), whose energy transfer greatly depends on the overlapping region between the absorbance of the analyte and emission of the sensor. Both of the aforementioned methods can give non-emissive or even low-emissive MOFs owing to the non-radiative slackening. On the other hand, a shift in wavelength can be activated by the host materials interacting with particular analytes. Similarly, external stimuli can also shift the emission maximum.
To date, there are mainly four luminescence response modes, including luminescence quenching (“Turn-Off”), luminescence enhancement (“Turn-On”), “Turn-On–Off–On” and ratiometric luminescence (RL) detection. Among them, “Turn-Off” is the most extensive, which is based on luminescence quenching resulting from the interaction between the analyte and the fluorophore, causing energy transfer from the fluorophore to analyte or even collapse of the framework. On the contrary, the luminescent sensing method of “Turn-On” detection is based on a new emission peak evolving from a previously dark background or an increase in luminescence intensity to show the apparent color change. The “Turn-On–Off–On” mode mainly involves some transition metal ions, such as Cu2+, Ag+ and Fe3+, whose co-introduction in LnFMOFH materials sometimes result in a designed effect. On other hand, the multi-center photo-response (especially dual emission) has great application in ratiometric or self-calibrated sensing (Fig. 4).
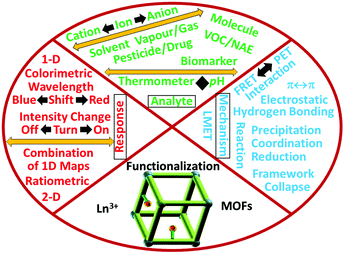 |
| Fig. 4 Scheme for lanthanide-functionalized MOF hybrids for luminescence-responsive chemical sensing, VOC = volatile organic compound and NAE = nitroaromatic explosives. | |
3. “Turn-off” luminescence response chemical sensing for lanthanide-functionalized MOF hybrids (LnFMOFH)
The principle of using MOF-based materials as luminescent probes depends on the variation in their luminescence intensity before and after their interaction with analytes. However, luminescence quenching is the most common phenomenon, which may be caused due to several factors, viz. photoinduced electron transfer (PET), intermolecular charge transfer, excited-state intermolecular proton transfer, excimer/exciplex, and fluorescence (Förster) resonance energy transfer (FRET). PET can be explained by predicting the electron distribution and energy of the LUMO and HOMO. As is known, dynamic quenching occurs owing to the collision between the excited-stated fluorophore and the quencher, whereas static quenching occurs because of the formation of non-emissive intermediates.38c,62,63
For LnFMOFH materials, Yan's group performed extensive works on “Turn-Off” sensing based on luminescence quenching.64 Qu et al. applied a straightforward PSM path to fabricate a hybrid material, Tb3+@MOF-SO3−, which was developed as a luminescence sensor for the detection of a biomarker for benzene, trans,trans-muconic acid (tt-MA), based on luminescence quenching. The luminescence of the Tb3+@MOF-SO3− suspension was totally quenched only by tt-MA (Fig. 5a (top)). Notably, as shown in (Fig. 5b (top)), the emission intensity of Tb3+ ions at 544 nm in an aqueous suspension of Tb3+@MOF-SO3−/tt-MA contrasts sharply with that in an aqueous suspension of Tb3+@MOF-SO3−/other urine chemicals, indicating that the hybrid can serve as a sensor for the selective recognition of tt-MA in aqueous media. Fig. 5a (bottom) shows a gradual decrease in the ligand-based emission and appearance of the typical emission of the Tb3+ ion as the tt-MA concentration increases from 0 to 200 μg mL−1. When the concentration of tt-MA is 5 μg mL−1, nearly half the intensity of the typical Tb3+ emission at 544 nm is quenched. When the tt-MA concentration is 100 μg mL−1, the QE value of the typical Tb3+ emission is up to 92%, although the blue emission band of the ligand can still be observed. Interestingly, under irradiation with a UV lamp, Tb3+@MOF-SO3− test papers showed a visible luminous variation from light green to nattier blue after soaking in various concentrations of tt-MA aqueous solution (from 0 to 100 μg mL−1) (Fig. 5b (bottom)), demonstrating the good response sensitivity of Tb3+ ions to tt-MA. At of low concentrations of tt-MA in the range of 0 to 20 μg mL−1, a certain linear relationship (R2 = 0.97), and a linear curve with Ksv calculated as 0.27932 mL μg−1 were achieved (Fig. 5c (bottom)). The LOD was estimated to be 0.1 μg mL−1 (ppm). Both the depressed emission lifetime of Tb3+ ions and the variation in the intensity ratio I(5D4 → 7F6)/I(5D4 → 7F5) of the Tb3+ ions demonstrate the weak coordination interaction between the tt-MA sites and Tb3+ ions. The strong absorption from 230 to 285 nm was largely overlapped by the absorption band of MOF-SO3− and Tb3+@MOF-SO3−. The competitive absorption and the inefficient LMET can quench the emission of both LC and Tb3+ ions. Moreover, the mechanism of dynamic quenching based on the collision between the excited-state Tb3+@MOF-SO3− and tt-MA is mainly responsible for the quenching effect of tt-MA on the luminescence of Tb3+ ions.64a
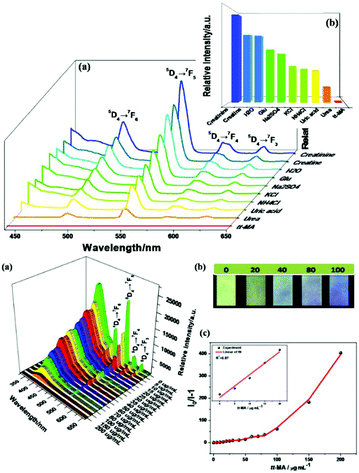 |
| Fig. 5 (Top) Emission spectra of Tb3+@MOF-SO3− in different aqueous solutions of common ingredients in urine (a) and the emission intensities (b) of the main peak at 544 nm assigned to the 5D4 → 7F5 transition of Tb3+ (λex = 291 nm). (Bottom) (a) Emission spectra of Tb3+@MOF-SO3− dispersed in aqueous solutions with different concentrations of tt-MA (λex = 291 nm). (b) Optical images of test paper under 254 nm UV light irradiation after immersion in aqueous solutions with different concentrations of tt-MA. (c) S–V curve of I0/I − 1 versus concentration of tt-MA. Reproduced with permission from ref. 64a Copyright 2018, the American Chemical Society. | |
Qu et al. synthesized a series of Zn2+/Cd2+-based MOFs and further selected {[NH2(CH3)2]4·[Cd6(pdda)4(HTz)1.5(H2O)6]·0.75DMF·3.5H2O}n (CdMOF) to fabricate a hybrid (Tb3+@CdMOF, H2pdda = 3,5-di(2,4-dicarboxylphenyl)pyridine, HTz = 1H-tetrazole) by cation-exchange PSM. The hybrids were demonstrated to exhibit potential for the practical luminescent detection of biomarker dichloroanilines (3,4-DCA and 3,5-DCA) (Fig. 6 (top)).64b Under UV lamp irradiation, 3,4-DCA and 3,5-DCA could induce a visible color change from green to colorless, and differences in the effect of different urine chemicals on the Tb3+@MOF emission were observed (Fig. 6 (top) inset). As shown in Fig. 6a and c (bottom), a gradual decrease in the emission of Tb3+@CdMOF was observed upon an increase in the concentration of 3,4-DCA and 3,5-DCA from 0 to 0.2314 mg mL−1. The corresponding relationship curves for both analytes were nonlinear and corresponded to the first-order exponential equation, suggesting diffusion-controlled quenching processes. At a low content in the range of 0 to 0.0266 mg mL−1, the probe exhibited a good linear correlation (R2 = 0.99) as the concentration of 3,4-DCA and 3,5-DCA increased (Fig. 6b and d (bottom)). The LODs were calculated to be 3.3 ppm and 2.6 ppm, respectively. In addition, the quenching sensitivity of 3,5-DCA to the hybrids was better than that of 3,4-DCA, which may originate from their different host–guest effects. The specific interactions between dichloroaniline and the Tb3+ ion is attributed to the quenching induced by 3,4-DCA and 3,5-DCA on the inter filter effect (IFE). The inefficient LMET can quench the typical Tb3+ ion emissions. When the concentrations of both analytes increased to above 0.0523 mg mL−1, the decrease in the lifetime values confirmed the existence of dynamic quenching for the probe towards 3,4-DCA and 3,5-DCA. The excitation spectra of Tb3+@CdMOF show significant blue-shifts with the appearance of new peaks at about 285 nm and a reduction in the original emission peaks at 300 nm, which give direct proof for the existence of strong static quenching. These results suggest that more than one quenching mechanism exists in the sensing process, i.e. IFE at low concentrations and dynamic and static quenching at high concentrations of 3,4-DCA and 3,5-DCA.
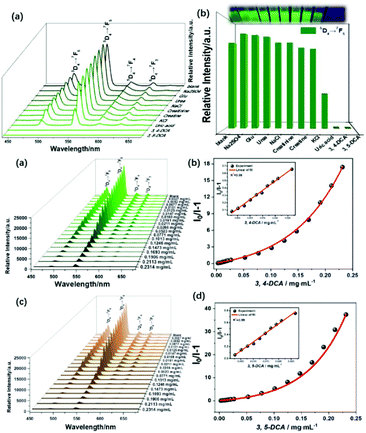 |
| Fig. 6 (Top) Emission spectra (a) and intensities (b) at 545 nm of Tb3+@CdMOF suspensions containing various urine chemicals. Inset: Photograph under 365 nm UV light. (Bottom) Emission spectra S–V curve of I0/I − 1 versus concentration of Tb3+@CdMOF dispersed in DMF with different concentrations of 3,4-DCA (a and b) and 3,5-DCA (c and d). Reproduced with permission from ref. 64b Copyright 2020, The Royal Society of Chemistry. | |
Lian et al. prepared a hybrid Eu3+@HfMOF by coordinate PSM, displaying MPA (methyl phosphoric acid)-induced luminescence quenching, which is due to the MLCT from MPA to Hf6+ and the generated exciplex. It was applied for the detection of ions in wastewater, and with good selectivity, high sensitivity (LOD = 0.4 ppm) and large linear range (10−7–10−3 M).64cFig. 7a (top) exhibits the strong and sharp emissions of the probe. The excitation spectrum shows a blue shift in the wavelength compared with that of the MOF due to the LMET from the ligand to Eu3+ ions. The sensing approach for organic phosphorus chemical warfare agents (OPCWAs) was further supported via luminescence measurement (Fig. 7b (top)), and there was no obvious change in the luminescence intensity except for MPA. In an MPA-containing suspension, the emission of Eu3+ was reduced markedly and quickly, and the difference in the 5D0 → 7F2 emission (ΔI) between the blank and test samples was several times that with other analytes (Fig. 7c (top)). The above evidence suggests that an exciplex may be formed between Eu@HfMOF and MPA. When the excited-state luminescence center collides with the MPA molecules, CT occurs due to the attraction between them, generating a weak fluorescent intermediate exciplex (also called excited-state CT complexes). Considering the strong Brønsted acidity of HfMOF and deprotonation of phosphoric acid, a complete possible mechanism was proposed. Firstly, the strong electron donor oxygen radical anion (R–O−) is generated via the deprotonation of MPA, and CT occurs from the donor to the excited-state Hf12 cluster as a strong electron acceptor, subsequently forming an exciplex. Finally, the ET from HfMOF to Eu3+ is forbidden, resulting in luminescence quenching (Fig. 7 (bottom)).
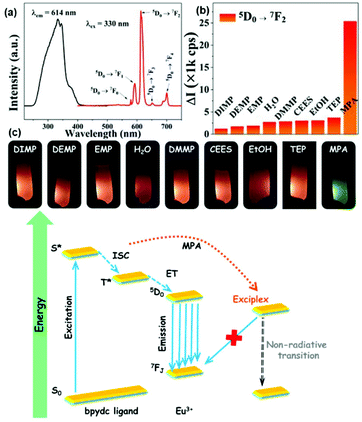 |
| Fig. 7 (Top) (a) Luminescent spectra of Eu3+@HfMOF in the solid state. (b) Histogram of 5D0 → 7F2 emission of Eu@1 in various solutions of OPCWAs; (c) photographs of Eu3+@HfMOF in suspensions with various OPCWAs. (Bottom) Mechanism of the MPA-induced quenching. Reproduced with permission from ref. 64c Copyright 2018, The American Chemical Society. | |
Recently, Qu et al. synthesized an anionic MOF, {[NH2(CH3)2]2·[Cd3.5(bdba)(Hbdba)(H2O)1.5]}n (Cd-MOF), H5bdba = 2,5-bis(3′,5′-dicarboxylphenyl)benzoic acid, where the accessible uncoordinated –COOH groups on Hbdba could be functionalized to form an Ln3+@Cd-MOF hybrid by coordinate PSM. CdMOF showed notable and selective fluorescent sensitization to Tb3+ ions, and thus Tb3+@Cd-MOF can be developed as a luminescent sensor for the detection of the biomarker diphenyl phosphate (DPP) via multiple quenching mechanisms.64d The emission spectra and intensities of Tb3+@Cd-MOF suspensions containing DPP contrasted sharply with that of other urine chemicals (Fig. 8 (top)). Under irradiation from a UV lamp, DPP exclusively induced a visible color change from bright-green to colorless (Fig. 8 (top), inset). As shown in Fig. 8a (bottom), a gradual decrease in the luminescence of Tb3+@Cd-MOF suspensions was observed with an increase in the concentration of DPP from 0 to 0.5226 mg mL−1. When the content of DPP was 0.5226 mg mL−1, there is almost no conspicuous emission detected. Within the full concentration range, the corresponding relationship curve exhibited a good linear correlation (R2 = 0.98) with a gradual increase in the concentration of DPP (Fig. 8b (bottom)). The absorption bands of DPP, H5bdba ligand, Cd-MOF and the hybrid showed the competitive absorption between DPP and Tb3+@Cd-MOF. The concentration-dependent lifetime measurement for Tb3+@Cd-MOF to DPP validated the existence of the dynamic quenching mechanism. Besides, the peak in the excitation spectrum of Tb3+@Cd-MOF displayed a gradual red-shift of 6 nm with a relatively high concentration of DPP (0.5226 mg mL−1), proving the existence of static quenching.
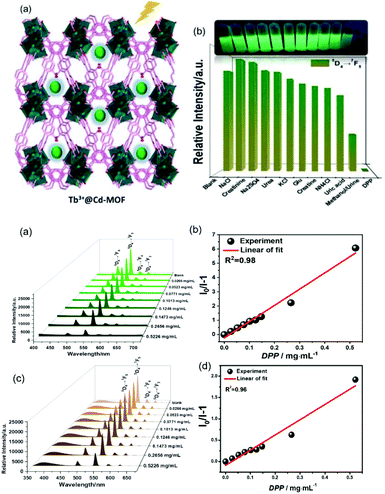 |
| Fig. 8 (Top) (a) Scheme for Cd-MOF containing uncoordinated carbonyl groups, indicating that the pores act as the PSM site for Ln3+-functionalization. (b) Intensities at 544 nm of Tb3+@Cd-MOF suspensions containing various urine chemicals. Inset: Corresponding photographs of different suspensions under 365 nm UV light. (Bottom) (a) Emission spectra of Tb3+@Cd-MOF dispersed in methanol with different concentrations of DPP, and (b) S–V plot of I0/I − 1 versus concentration of DPP. (c) Emission spectra of T Tb3+@Cd-MOF dispersed in reconstituted urine samples with different concentrations of DPP and (d) S–V plot of I0/I − 1 versus concentration of DPP. Reproduced with permission from ref. 64d Copyright 2020, the American Chemical Society. | |
4. “Turn-on” luminescence response chemical sensing for lanthanide-functionalized MOF hybrids (LnFMOFH)
For “Turn-On” luminescence response sensing in LnFMOFH materials, the reported examples are much less than that for the “Turn-Off” mode. Also, some “Turn-On” modes are based on the introduction of a quenching transducer, and then realizing an enhancement in luminescence. Thus, herein, these types of works will be ascribed to the “Turn-On–Off–On” mode and will be separately discussed in the next section. Sun et al. prepared an Ln3+ PSM GaMOF hybrid (Ln = Eu, Tb, Sm, Dy). Upon 314 nm excitation, Eu-GaMOF, Tb-GaMOF and Eu/Tb-GaMOF all exhibited their respective strong sharp emission bands. However, Sm-GaMOF and Dy-GaMOF showed very similar emissions to that of MIL-61, and almost no luminescence of Sm3+ and Dy3+, which could be effectively sensitized by co-PSM Ag+. Besides, single-phase white light emitters based on the resulting 4d–4f heterometallic co-PSM MOFs hybrid could be realized. Furthermore, SmGaMOF showed more highly sensitive and selective sensing towards Ag+.65a Gamonal et al. use two isostructural LnMOFs including an amino group as the sensitive recognition center for NO2 molecules. A gas sensing mechanism exploiting Ln3+ luminescence modulation upon NO2 adsorption was demonstrated. The transfer of energy from the ligands to Ln3+ was strongly dependent on the presence of NO2, resulting in an unprecedented photoluminescent sensing scheme. Thereby, NO2 exposure triggered either a reversible enhancement or a decrease in the luminescence intensity of the Ln3+ ion.65b
Xu et al. assembled a multi-component hybrid material via the integration of EuMOFs and organic dyes (methyl red, MR). The carboxylic acid group of MR offered the possibility for covalent linking to amino group-rich EuMOFs to yield MR@EuMOFs, which contained two emission centers, MR and Eu3+, due to the effective ET from the MOF matrix to the Eu3+ ions and MR. Both emission centers showed pH-induced intensity changes, which can be constructed for monitoring BAs, well-known indicators of food spoilage and alkaline matter.66a They first investigated the sensing behaviors of the modified-MOFs for HI by adding MR@EuMOFs (3 mg) to an aqueous solution of HI (500 μM). As shown in Fig. 9 (top), the luminescence intensity of Eu3+ decreased at 613 nm (FEu: 3-fold) and the maximum emission wavelength of MR increased (FMR: 44-fold), leading to the emission color of the MR@EuMOFs changing from red to blue under a UV lamp. The sensing system also exhibited similar luminescence changes but with different sensitivities for other common BAs because of their different basicities. MR@EuMOFs (40 mg) was solidified into a CMC-Na hydrogel to easily prepare a portable sensor. To avoid the influence of the surroundings and to imitate spoilage in a packaged food system, they used the concentration of HI in an aqueous solution as the detection environment. When the hydrogels were exposed to the HI vapor, the pH increased due to the basicity of HI. Fig. 9a and b (bottom) show that the luminescence intensities of FEu and FMR changed with the HI aqueous solution concentration and the exposure time. Saturation was reached after 25 min for all concentrations, and the corresponding luminescence spectra are given in Fig. 9c (bottom). The emission intensity of Eu3+ decreased, while the MR-based emission increased as the HI concentration increased, and the hydrogel color gradually changed from red to blue-violet, to blue (Fig. 9d (bottom)). The HI concentration was quantitatively analyzed using the response signals, being defined as the ratio of FMR to FEu (Fig. 9e (bottom)). It showed the good linear relationship (R2 = 0.99028) between the response signals and the HI vapor concentration in the range of 0–250 μM, and an LOD of 0.1 μM.
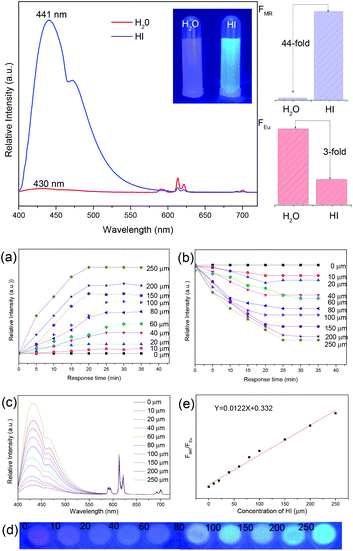 |
| Fig. 9 (Top) Emission spectra of MR@EuMOFs and comparison of FMR and FEu before and after treatment with HI at an excitation wavelength of 370 nm. Inset: Optical photograph under 365 nm UV-light irradiation. (Bottom) Emission intensity of FMR (a) and FEu (b) at different times (0–35 min) upon exposure to HI at concentrations in the range of 0 μM to 250 μM (λex = 370 nm). Concentration-dependent emission spectra (c) and corresponding optical photograph under 365 nm UV-light irradiation (d) of MR@EuMOF sensory hydrogel in the presence of different concentrations of HI in the range of 0 to 250 μm. (e) Linear fitted curve showing FMR/FEuvs. HI concentration, λex = 370 nm. Reproduced with permission from ref. 66a Copyright 2017, WILEY-VCH. | |
Liu et al. used a PSM cation exchange method to prepare a series of Ln3+ (Ln = Eu, Tb, Sm and Dy)-functionalized PbMOFs.66b Remarkably, the rapid and stable fluorescent sensitization of the Eu3+@PbMOF hybrid was observed in the presence of fleroxacin in aqueous solution, indicating that it can be designed to be a “Turn-On” probe for the detection of fleroxacin. Upon the addition of fleroxacin to the solution, the intensity of the 5D0 → 7FJ (J = 0–4) transitions of the Eu3+ ions was extremely enhanced. Subsequently, to verify the unique selectivity of the probe, nine types of quinolone drugs were added to the aqueous solution with Eu3+@1, and the luminescence responses were examined. As depicted in Fig. 10(a) and (b), Eu3+@PbMOF exhibited different degrees of luminescence response to the quinolone drugs, and the ratios between the intensity of fleroxacin and that of other drugs at 612 nm are marked in the figure. The luminescence titration experiment revealed an obvious linear relationship (R2 = 0.99541) between the emission intensity of the solution at 613 nm and the concentration of fleroxacin in the experimental range, with the LOD of 43.91 ng m−1 (Fig. 10(c)). The mechanism of luminescence enhancement can be easily attributed to the self-assembly of Eu3+ ions with β-diketonate. The structure of fleroxacin contains a β-diketone moiety, which produces an effective coordination interaction and “antenna effect” with Eu3+. The phosphorescence spectra exhibited the triplet state energy of the these quinolone drugs, and fleroxacin showed a good match with Eu3.
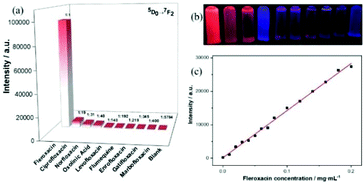 |
| Fig. 10 (a) Emission intensities of the main peak at 612 nm assigned to the 5D0 → 7F2 transition of Eu3+ (λex = 377 nm). (b) Photograph of Eu3+@PbMOF aqueous suspensions containing different quinolone drugs under 365 nm UV light, where the order is the same as that in (a). (c) Fitting curve of the emission intensity (613 nm) of Eu3+@PbMOF vs. fleroxacin concentration. Reproduced with permission from ref. 66b Copyright 2019, The Royal Society of Chemistry. | |
Sun et al. prepared a novel and feasible method for the detection of N-methylformamide (NMF) with a hybrid (Eu3+@Ga-MOF).66c As shown in Fig. 11a (top), upon the addition of NMF to the suspensions of Eu3+@GaMOF, the luminescence intensity of Eu3+ at 613 nm was enhanced significantly, while the LC emission was suppressed. The bright red color could be observed with the naked eyes under a UV lamp. The intensity of the 5D0 → 7F2 transition increased by 5 times in the presence of NMF, as shown in Fig. 11b (top). Only NMF induced distinct color change in Eu3+@GaMOF from dark to bright red under a UV lamp, which can act as a sign indicating the emergence of DMF in an individual (inset of Fig. 11b (top)). The recycling performance of Eu3+@GaMOF as a urinary NMF sensor is shown in Fig. 11a (bottom). After four cycles, every cycle exhibited the corresponding enhancement and recovery of the luminescence intensity. As shown in Fig. 11b (bottom), under UV irradiation, the luminescent color of the test card smeared with the suspension of NMF-Eu3+@GaMOF (NMF: 0.01 M) was bright red and could be observed by the naked eyes, while the test card smeared with the suspension of Eu3+@GaMOF in the absence of NMF did not exhibit obvious luminescence. Thus, this sensor can judge the degree of DMF intoxication in an individual based on the color change.
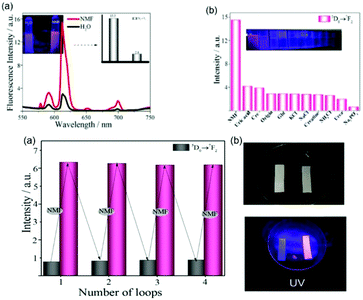 |
| Fig. 11 (Top) (a) Luminescence spectra of Eu3+@GaMOF in the absence and presence of NMF. Inset: changes in the luminescence intensity (613 nm) and luminescence color (under UV-light irradiation of 256 nm) induced by the addition of NMF. (b) Corresponding luminescence intensity (5D0 → 7F2 transition) of Eu3+@GaMOF with various urine components. Inset in (b): corresponding photograph under UV-light irradiation (254 nm). (Bottom) (a) Luminescence intensities of Eu3+@GaMOF at 613 nm during three consecutive enhancement and recovery cycles. (b) Prepared test paper in the absence and presence of NMF upon irradiation with a UV lamp. Reproduced with permission from ref. 66c Copyright 2018, Elsevier. | |
5. “Turn-on–off–on” luminescence response chemical sensing for lanthanide-functionalized MOF hybrids (LFMOFH)
In nature, the “Turn-On–Off–On” luminescent response belongs to a type of “Turn-On” mode if the final response to a special analyte is considered. Generally, here “Turn-On–Off” is the strategy or path to realize the final “Turn-On” effect. Thus, to distinguish it from the direct “Turn-On” mode, it will be discussed separately. Zeng et al. synthesized a dual-emission multivariate Eu3+@AlMOF via a sequential mixed-ligand self-assembly and PSM method. The hybrid possessed two emission bands, i.e. the strong blue emission from the ligands sensitive to hypochlorite and the red emission from Eu3+, which was almost invariable. Thus, it could realize the RL “On–Off” sensing of HCl with an LOD of 0.094 μM. Besides, the suppressed blue emission was recovered after the addition of ascorbic acid (AA), which consumed ClO−via the redox reaction. Therefore, it can be further employed for the RL “On–Off–On” sensing of AA.67a Wang et al. developed a dual-signal probe based on a boric acid (BA)-functionalized LnMOF (BA-Eu-MOF) for the detection of Hg2+ and CH3Hg+ ions. The BA covalently PSM linker not only acted as an “antenna” to sensitize the luminescence of Eu3+, but also provided reaction sites for Hg2+ and CH3Hg+. Owing to the electron-withdrawing effect of the BA group, the “antenna” effect of the ligand was passivated, and BA-Eu-MOF showed weak red emission in water (“Turn-On–Off”). Upon the addition of Hg2+ or CH3Hg+ to the system, a transmetalation reaction occurred, replacing the BA groups with Hg2+ or CH3Hg+, which triggered the “antenna” effect of BA to achieve an enhancement in the emission of Eu3+ (“Turn-On”).67b
More popular works used co-PSM metal ions to quench the luminescence of Ln3+-functionalized MOF hybrids, realizing the “Turn-On–off” effect, and then the response to the analyte resulted in the subsequent “Turn-On” response. Presently, the most studied co-PSM metal ion is Cu2+.68,69 Lian et al. synthesized a phosphonate MOF and its Eu3+ hybrid Eu3+/Cu2+@AlMOF, where its Eu3+ luminescence recovered in the presence of uric acid. This “On–Off–On” pattern was utilized for the detection of uric acid, which is the final metabolite of purine68a (Fig. 12 (top)). As shown in Fig. 12 (middle), only uric acid induced a remarkable increase in the luminescence intensity of Eu3+ at 612 nm. Uric acid did not influence the luminescence of Eu3+@AlMOF, whereas the Eu3+/Cu2+@AlMOF hybrid show almost no luminescence emission, and thus the quenching effect of Cu2+ on the 4f–4f emission of Eu3+ was obvious. However, upon the addition of uric acid to the Eu3+/Cu2+@AlMOF suspension, the luminescent intensity was recovered. They found a good linear relationship (R = 0.991) between the intensity ratio of the 5D0 → 7FJ transition (I0/I) and the logarithm of uric acid concentration, with an LOD of 1.6 μmol L−1. The test paper is shown in Fig. 12b and c (bottom), where under UV irradiation, the luminescent colour of the test paper immersed in urine containing 1000 μmol L−1 uric acid was bright red, whereas the other test papers treated with a low concentration of uric acid exhibited almost no luminescence.
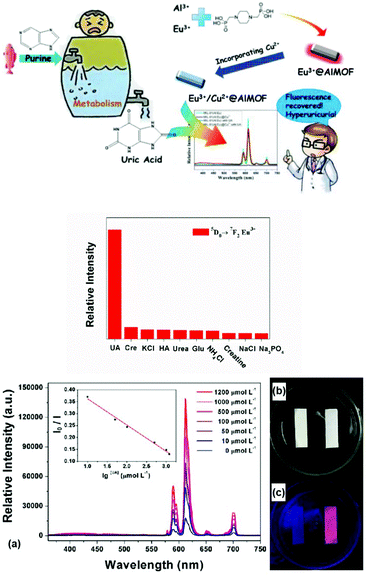 |
| Fig. 12 (Top) Schematic drawing of the whole sensing mechanism and process. (Middle) Histogram based on the intensity of the 5D0 → 7F2 transition of Eu3+ in Eu3+/Cu2+@AlMOF toward various urine chemicals. (Bottom) (a) Luminescence spectra of Cu2+@AlMOF in the presence of different concentrations of uric acid aqueous solution, and plot of I0/I versus the logarithm of the concentration of uric acid. (b) and (c) Test papers under light and UV irradiation. Reproduced with permission from ref. 68a Copyright 2017, the American Chemical Society. | |
Hao et al. designed a luminescent nanoprobe based on a Eu3+/Cu2+@ZrMOF hybrid. The introduction of Cu2+ not only tuned the luminescence of Eu3+via the LMET process, but also served as the reactive sites for urinary thiodiglycolic acid (TDGA) as a biomarker of carcinogenic vinyl chloride monomer (VCM).68b In contrast to TDGA, the addition of other urine components only caused negligible changes in the luminescence spectrum intensity (Fig. 13a). In accordance with the spectral changes, under a UV lamp irradiation, only TDGA induced a visible color change from colorless to red. The differences in the effects on the hybrid probe emission between TDGA and other urine components could be easily distinguished by the naked eye. As shown in Fig. 13b, the emission signals of Eu3+ in Eu3+/Cu2+@ZrMOF were found to gradually increase with an increase in the amount of TDGA added, and tended to become saturated when the concentration exceeded 700 mg L−1. This linear relationship between the emission intensity (IEu) and [TDGA] was fitted with a correlation coefficient (R2) of 0.9878, with an LOD of 89 ng mL−1 for TDGA. As shown in Fig. 13c, the color of the Eu3+/Cu2+@ZrMOF powder changed from brilliant blue to light blue, and finally almost colorless with an increase in the concentration of TDGA from 0 to 700 mg L−1, i.e., the addition of TDGA bleached the blue color of the powders. The strong complexation ability of TDGA for Cu2+ resulted in the leakage of Cu2+ from the MOF hybrid and weakened the quenching effect of Cu2+ on the emission of Eu3+, thus leading to a luminescence enhancement with the sample color faded. Furthermore, a test strip was prepared, the luminescent color of which changed from dark to dark red, faint red, purplish red and finally bright red when soaked in urine samples with an increasing content of TDGA (Fig. 13d).
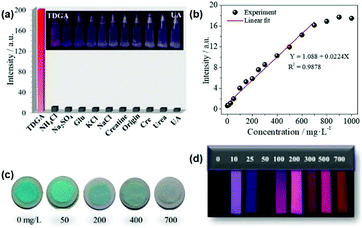 |
| Fig. 13 (a) Luminescence intensities (5D0 → 7F2 transition, 614 nm) of Eu3+/Cu2+@ZrMOF toward various urine components (λex = 310 nm). Inset: Corresponding photograph under a UV-lamp (254 nm). (b) Plot of intensity of Eu3+/Cu2+@ZrMOF at 614 nm vs. TDGA concentration over the linear range of 0–700 mg L−1. (c) Photographs showing the color changes of the Eu3+/Cu2+@ZrMOF sample treated with different concentrations of TDGA. (d) Optical images of the test papers under 254 nm UV light after immersing them in urine spiked with different concentrations of TDGA. Reproduced with permission from ref. 69b Copyright 2018, the American Chemical Society. | |
Ji et al. designed a strategy for the detection of aspartic acid (Asp) based on a PSM MOF Cu2+/Tb3+@ZnMOF. Subsequently, the luminescence-quenched hybrid was developed as a luminescence “Turn-On” probe for Asp with an LOD of 0.54 ppm. In addition, this “On–Off–On” pattern was further employed to construct an advanced analytical model based on an IMPLICATION-type logic gate.68c Zhang et al. utilized a PSM Eu3+/Cu2+@InMOF fluorescent film, realizing the luminescent “Turn-On–Off–On” sensing of gaseous H2S at room temperature with the LOD of 0.535 ppm. The sensor was designed due to the strong affinity of H2S with Cu2+. Upon the formation of CuS, the “antenna effect” between the ligand and Eu3+ recovers, resulting in the “Turn-On” emission of Eu3+.68d Besides Cu2+–Eu3+ co-PSM MOF hybrid systems, a similar sensing mode was also employed for Cu2+–Tb3+ PSM MOF hybrids.69 Liu et al. proposed a sensor based on Cu2+/Tb3+@MOF for the detection of amyloid beta-peptide (Aβ) monomer, a biomarker for Alzheimer's disease (AD). Here, Tb3+ acted as the luminescence centre and building block and Cu2+ as the signal modulator and recognition unit. Interestingly, Cu2+/Tb3+@MOF was almost non-emissive due to the quenching effect of Cu2+ in the MOF. The presence of Aβ triggered a significant emission enhancement in the Cu2+/Tb3+@MOF assay due to the high binding affinity of A for Cu2+ and the subsequent suppression of the quenching effect. Thus, it is a “Turn-On–Off–On” responsive sensor.69a Zheng et al. also performed “Turn-On–Off–On” responsive sensing research based on Tb3+ PSM CuMOF hybrids.69b,c They firstly synthesize a CuMOF and its hybrid Tb3+@CuMOF via PSM for sensing H2S. The obtained hybrid emitted a weak typical Tb3+ ion emission and strong LC emission. Interestingly, H2S, as a strong electron donor, could strongly enhance the luminescence of Tb3+ through its superior affinity for Cu2+ ions. Thus, the hybrid belongs to a “Turn-On–Off–On” RF probe for H2S detection with an LOD 1.20 μM.69b Then they designed and developed two novel hybrids based on a Tb3+/Yb3+-co-functionalized CuMOF, which displayed broad ligand-centered emission and weak typical Tb3+ ion emission. Similarly, it was used as an RF sensor (“Turn-On–Off–On” response for Tb3+ luminescence) for the metabolic product NMF of DMF in the human body (LOD = 0.02 mu M).69c Sun et al. designed a luminescent probe based on a Tb3+/Cu2+ MOF for the beamed monitoring of urinary sarcosine, a differential metabolite indicating the progression of prostate cancer (PCa). The incorporation of Cu2+ not only tuned the luminescence of Tb3+via the LMET process, but it also provided available sites for sarcosine. Thus, Cu2+/Tb3+@GaMOFs presented significantly enhanced luminescence (“On–Off–On”) towards sarcosine.69d
Lian et al. reported the synthesis of a composite hydrogel Fe3+/Eu3+-MOF@SA for the diagnosis of penicillin anaphylaxis. Penicillamine is the metabolite of penicillin, which could be detected by Fe3+/Eu3+-MOF@SA both in aqueous solution and serum. More significantly, the determination of β-lactamase by Fe3+/Eu3+-MOF@SA was achieved through a “Turn-On–Off–On” luminescence trigger pattern.70 Fe3+ was found to quench the emission of Fe3+/Eu3+-MOF, and penicillamine had a strong affinity for Fe3+ and quenched the luminescence of Fe3+/Eu3+-MOF. Thereby, a luminescence enhancement for Fe3+/Eu3+-MOF@SA in the presence of penicillamine was demonstrated, as shown in Fig. 14a (top), the response of which to penicillamine increased by about 32-fold. The luminescence data was recorded and compared, as shown in Fig. 14b (top), and it was found that only penicillamine could induce a remarkable rebound in the luminescence of Eu3+. The luminescence color of 1@SA changed from colorless to red after the addition of penicillamine (Fig. 14c (top)). As shown in Fig. 10a (middle), the luminescence signal of Fe3+/Eu3+-MOF@SA was found to gradually increase with an increase in the concentration of β-lactamase. The fitting curve of β-lactamase concentration vs. luminescent intensity (I3) is plotted in Fig. 14b (middle), with an LOD of 1.25 U mL−1. The changes in these hydrogels under UV irradiation are shown in Fig. 14c (middle). The luminescent color of the cordiform hydrogels changed from dark to bright red with an increase in the concentration of β-lactamase.
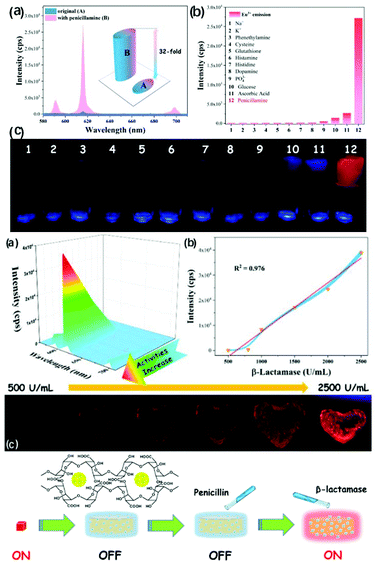 |
| Fig. 14 (Top) (a) Emission enhancement effect of penicillamine to Fe3+/Eu3+-MOF@SA hydrogel, and the inset shows a comparison of the intensities. (b) Emission intensities of Fe3+/Eu3+-MOF@SA with various chemicals under excitation at 325 nm. (c) Corresponding photographs of (b) under UV radiation. (Middle) (a) Luminescence responses of Fe3+/Eu3+-MOF@SA hydrogels with a change in the concentration of β-lactamase. (b) Linear plots of the intensities of Fe3+/Eu3+-MOF@SA and β-lactamase concentration with error bars. (c) Corresponding photographs of the Fe3+/Eu3+-MOF@SA hydrogels with various β-lactamase serum solutions. (Bottom) Structure of MOF-based hybrid hydrogel Fe3+/Eu3+-MOF@SA and the determination of β-lactamase by this hydrogel through a “Turn-On–Off–On” luminescence trigger pattern. Reproduced with permission from ref. 70 Copyright 2019, The Royal Society of Chemistry. | |
6. Dual-responsive ratiometric luminescence (RL) chemical sensing for lanthanide-functionalized MOF hybrids (LnFMOFH)
In contrast to single-mode sensing, dual-mode ratiometric luminescent (RL) sensors can overcome the abovementioned limitations and provide accurate detection through self-calibration with two emissive bands. The RL detection mechanism can be summarized as follows: (1) two emissions with “Turn-On” and “Turn-Off”, where one emission increases while another decreases; (2) two emissions with “Turn-On” or “Turn-Off”, where one emission changes (increases or decreases), while the other maintains its intensity; and (3) the same change in the two emissions, where both emissions increase or decrease to varying degrees. Thus, with the above diverse combinations of luminescence intensity change, many RL sensors have been developed for all types of applications. Nowadays, the tactics of ratio sensing caused by dual-emission MOFs can be summarized as follows: (1) synthesis of self-illuminating MOFs based on MC and LC or introducing a specific target to bridge two emissions, which is common for LnMOF- or LnFMOFH-based materials. (2) Combining single-emissive MOFs with a chromophore such as QDs and dyes. (3) Encapsulating two chromophores into the frameworks of non-emissive MOFs. MOFs with intrinsic dual-emission are mainly synthesized via three routes, where one is using mixed lanthanide metal ions to form double-emitting materials, namely, bimetallic MOFs. The other is the combination of two luminescent ligands, and the last is the target analyte can also be the key to emitting dual luminescence, where it can work as a bridge, causing energy transfer between the metal center and ligands.71a–c In some cases, RL sensors can also be composed of a single lanthanide and luminescent ligand.71d Thus, considering the extensive research on ratiometric sensing for both MOFs and Ln3+-functionalized MOF hybrids,72–74 it can be noted that LnFMOFH materials are ideal candidates for RL sensing. Accordingly, here, it will not be discussed in detail, and only some recent examples on this topic are presented.
Qin et al. developed luminescent, bimetallic MOFs as luminescent sensors for NMF with a focus on Eu3+ and Tb3+ MOFs as visible emitters with red and green emission, respectively. The concentration dependence of the emission spectra (0–1) of the mixed MOFs [EuxTb1−x(FDA)3] (x = 0.01, 0.02, 0.2, 0, and 2; H2FDA = 2,5-furandicarboxylic acid) is illustrated in Fig. 15a. Noticeably, with an increase in the concentration of NMF, the intensity of the Tb3+ luminescence in the hybrid substantially increased, in contrast to the Eu3+ emission. The concentration-dependent curve showed a good linear relationship, which is represented as the concentration versus intensity ratio (5D0 → 7F2/5D4 → 7F5 defines the ratiometric parameter I544 nm/I614 nm), and further confirmed that the hybrid is a self-calibrating luminescent sensor (Fig. 15b). As illustrated in Fig. 15c, the concentration-dependent luminescence emission of the hybrid was systematically tuned from red to green at different concentrations of NMF. Furthermore, the luminescence colour changes make it particularly easy to use the sensitive luminescent colorimetric ratiometric for visualizing the NMF concentration over a wide range.
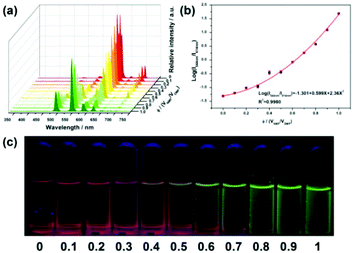 |
| Fig. 15 (a) Suspension-state luminescent spectra of [Eu0.1Tb1.9(FDA)3(DMF)2]·2DMF in volume ratio (ϕ) of NMF/DMF in the range of 0 to 1 excited at 300 nm. (b) Correlation curve for the response of the sensor toward the NMF in the volume ratio range of 0–1 and (c) corresponding luminescence images of 1 under UV light. Reproduced with permission from ref. 74b Copyright 2018, The Royal Society of Chemistry. | |
Zhang et al. constructed a luminescent Ln3+ exchange PSM MOF hybrid (Eu3+@ZnMOF) to detect formaldehyde (FA) in water and food. The two luminescent centers of Eu3+@ZnMOF have completely different responses to FA, which can offer a reference for each other, and thus it can be designed as a precise self-calibrating luminescent detector for FA. Based on this, a portable self-calibrating luminescent logic detector capable for the gradient detection of FA was designed.74c The three different outputs of this logic detector are L (low), H (high), VH (very high), corresponding to three different inputs (<6 ppm, 6–10 ppm, and >10 ppm), respectively. As shown in Fig. 16a (top), the luminescence of Eu3+ (λ614 nm) was quenched completely after it was immersed in FA, while that of Bio-MOF-1 (λ405 nm) exhibited a remarkable enhancement compared with other solvents. The remarkable change could also be clearly distinguished by naked eye under UV light irradiation, as shown in the inset of Fig. 16b (top). As shown in Fig. 16b (bottom), using the concentrations of FA and λex as double inputs and the intensities of IL/IEu as the output, the first logic gate named Gate 1 was designed. In the truth table of Gate 1 in Fig. 16e (bottom), only if both FA and λex are present, output1 is ‘1’. It was clearly concluded that the main function of the first Gate 1 is to detect the presence of FA. If there is no FA, the tandem logic gates are broken. If FA exists, output 1 is ‘1’, and consequently the logic detector outputs “L (low)” and a red light, which is visible with the naked eye. Next, the output of Gate 1, the concentrations of FA and λex were used as the three inputs of Gate 2 to detect whether the concentration of FA exceeded 6 ppm. In the histogram for IL/IEu in Fig. 16a (bottom), the threshold of Gate 2 is labelled as ‘1’. If the concentration of FA is greater than 6 ppm and less than 10 ppm and Gate 1 outputs ‘1’, this tandem logic gate system continues to operate. Accordingly, output 2 is definitely observed to be ‘1’ in the truth table of Gate 2 in Fig. 16e (bottom), and consequently it shows a purple light and “H (high)”. If the concentration of FA is over 10 ppm, it goes to Gate 3. When Gate 2 outputs ‘1’, the output of Gate 3 is ‘1’ in the presence of excitation. Hence the logic detector outputs “VH (very high)” and a blue light visible with the naked eye, revealing the concentration of FA at a relatively high value. This process of gradient sensing FA described above is also vividly and intuitively represented in Fig. 16c and d (bottom).
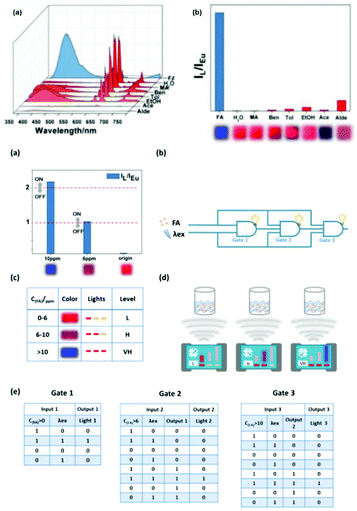 |
| Fig. 16 (Top) (a) Luminescent spectra of Eu3+@ZnMOF dispersed in different solutions when excited at 313 nm. (b) Histogram for IL/IEu of Eu3+@ZnMOF in various solutions (inset: photo of different solutions under UV light). (Bottom) (a) Column diagram of the luminescence intensity, where the dashed line shows the threshold. (b) Electronic equivalent circuitry of the combinational logic gate system in the logic detector. (c) Corresponding color, lights and FA level for different concentrations of FA on the screen of the logic detector. (d) Simple scheme for the sensing process of the logic detector. (e) Truth table of Gate 1, Gate 2 and Gate 3. Reproduced with permission from ref. 74c Copyright 2020, The Royal Society of Chemistry. | |
7. The LMET for luminescence response upon chemical sensing in lanthanide-functionalized MOF hybrids (LnFMOFH)
The combination of different functional or non-functional organic ligands with different inorganic moieties can result in versatile types of PL signals when using MOFs as probes for analyte detection. As is known, for MOFs host themselves, all types of interaction between metal ions and ligands are possibly involved, which determine the final photophysical properties of the whole hybrid. Therefore, LnFMOFH materials mainly have an influence on these interactions.75 Therefore, when LnFMOFH materials respond to analytes, the most common interaction occurs between the analytes and metal ion or linkage or both of them. Subsequently, analytes may change the interaction and energy transfer from the ligand to Ln3+ (LMET). Generally, almost all the luminescence responses of LnFMOFH materials for sensing analytes are ascribed to their influence on the LMET process in the hybrid system in the final analysis. Therefore, LMET is too extensive and common to be excessively emphasize. Herein, only two recent examples are present to highlight this point.
Zhou et al. described a new strategy for the generation of ratiometric MOF thermometers, which was illustrated by imparting an additional lanthanide luminescence to a robust MOF with intrinsic ligand-based emission to form a dual-emissive hybrid for highly sensitive temperature sensing over the physiologic temperature range.76a The imparted emission was sensitized by the parent framework via ET from the organic linkers embedded in the framework. The dual emissions of the hybrid exhibited different thermal dependences, thus enabling their intensity ratio to be highly sensitive to temperature. To examine the feasibility of Eu3+@ZrMOF for ratiometric thermometry, its temperature-dependent photoluminescence properties were studied (Fig. 17 (top)). Eu3+@ZrMOF exhibited the totally opposite thermal dependence with respect to the emissions of its linkers and Eu3+. With an increase in the temperature, the Eu3+ emission intensity declined, while the LC emission significantly increased. The significant luminescence enhancement of the linker and the decrease in Eu3+ emission in the Eu3+@ZrMOF hybrid may be attributed to the back energy transfer (BEnT) from the Eu3+ cations to the linker. The decay time of Eu3+ is reduced with an increase in temperature, while the decay time of the ligand emission slightly increases as the temperature elevates. This phenomenon further verifies the BEnT from Eu3+ to MOF. These results indicate the occurrence of back energy transfer from Eu3+ to the bpydc linkers embedded in framework. To study the BEnT mechanism in detail, the energy back transfer rates from Eu3+ to the linkers (KBEnT) were estimated. The temperature-dependent emission lifetimes (Eu3+) of the MOF hybrid were recorded for the determination of Kb and Ea (Fig. 17a (bottom)). The calculated KBEnT was enhanced with an increase in temperature (Fig. 17b (bottom)). The slope of the Arrhenius plots for KBEnT is 1.98, resulting in a value of 44 kJ mol−1 for the activation energy, Ea (Fig. 17c (bottom)). It is interesting that this LMET process does not commonly occur from the metal ion (Eu3+) to ligand.
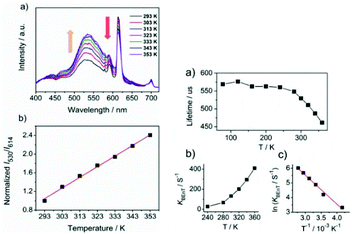 |
| Fig. 17 (Left) (a) Temperature-dependent emission spectra of Eu3+@ZrMOF and (b) intensity ratio of ligand (530 nm) to Eu3+ (614 nm) as a function of temperature. Ratios are normalized to the value at 293 K. (Right) (a) Temperature-dependence of luminescence lifetime of Eu3+ in Eu3+@ZrMOF. (b) Thermometric response curve plotting back energy transfer rate (KBEnT) vs. temperature. (c) Arrhenius plots for back KBEnT constants of Eu3+@ZrMOF. Reproduced with permission from ref. 76a Copyright 2015, The Royal Society of Chemistry. | |
Zhang et al. chose an LnFMOFH material as the signal transducer to construct a dual-functional intelligent logic detector, which could visually and simultaneously two-dimensional monitor pyrethroid (PYR) exposure time and exposure extent.76d 3-Phenoxybenzoic acid (3-PBA) and 3-phenoxybenzaldehyde (3-PBD) have different quenching effect towards Eu3+ emission under the different excitation of 313 nm and 322 nm. As shown in Fig. 18a (top), the Eu3+@Hf-MOF treated by 3-PBA and 3-PBD exhibited the same PXRD pattern with the pristine sample, indicating that the quenching effect cannot be ascribed to the framework collapse of Eu3+@Hf-MOF. As shown in Fig. 18b (top), the unchanged lifetime of Eu3+ in 3-PBA/3-PBD is strong evidence to prove the absence of interaction between them. Fig. 18c (top) shows the UV-vis spectra of 3-PBA and 3-PBD, where the absorption of 3-PBD completely overlaps with the excitation of 313 nm and 322 nm, while the absorption of 3-PBA can only overlap with the excitation of 313 nm. Hence, the excitation light absorbed by the ligand is reduced, which further decreases the antenna effect between the ligand and Eu3+. As shown in Fig. 18d (top), with the addition of 3-PBA, a red-shift in the excitation band can be clearly observed, and thus it can be inferred that there is an exciplex formed by the framework of Eu3+@Hf-MOF and 3-PBA, which causes the obvious red shift of the maximum excitation. Further, a dual-functional logic detector was constructed (Fig. 18a (bottom), which was composed of two combinational logic gates, named Part 1 and Part 2. Part 1 contains two gates (Gate 1 and Gate 2) and the function of the Part 1 is to infer the exposure time of pyrethroid by switching the excitation light to detect the existence of 3-PBD and 3-PBA. With λ313 nm (the excitation is 313 nm) and EA/D (existence of 3-PBA or 3-PBD) as the two inputs and λ614 nm as the output 1, the main function of Gate 1 is to distinguish 3-PBD and 3-PBA from other interfering substances under the threshold of ‘1’ (Fig. 18b (bottom)). Considering that the excitation in the logic detector was fixed, it will not be described subsequently, and it is acquiesced as ‘1’. If input 1 is (1, 1), output 1 is ‘0’, which means if one of 3-PBA or 3-PBD exists, the luminescence is quenched. With λ322 nm (excitation is 313 nm) and EA (EA is ‘1’ means 3-PBA exists and ‘0’ means 3-PBD exists) as the two inputs, and λ614 nm as the output 2, the role of Gate 2 is to further distinguish 3-PBD and 3-PBA with the threshold of ‘1’ (Fig. 18c (bottom))). When output 1 is ‘0’, the excitation is 322 nm and EA is ‘1’, the output 2 is ‘1’, which means the biomarker of pyrethroid is only 3-PBA since the exposure time is relatively long.
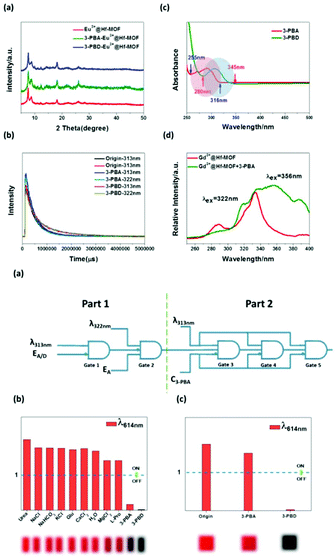 |
| Fig. 18 (Top) (a) PXRD patterns of Eu3+@Hf-MOF and Eu3+@Hf-MOF after treatment with 3-PBA and 3-PBD. (b) Luminescence decay curves of Eu3+@Hf-MOF and Eu3+@Hf-MOF immersed in 3-PBA and 3-PBD under the excitation of 313 nm and 322 nm respectively. (c) UV-vis spectra of Eu3+@Hf-MOF immersed in 3-PBA (red line) and 3-PBD (green line). (d) Excitation spectra of Gd3+@Hf-MOF (red line) and Gd3+@Hf-MOF in 3-PBA (green one). (Bottom) (a) Electronic equivalent circuitry of the dual-functional intelligent visual logic detector. (b) Column diagram of the luminescence intensity of Eu3+@Hf-MOF under the excitation of 313 nm, where the dashed line shows the threshold. (c) Column diagram of the luminescence intensity of Eu3+@Hf-MOF and Eu3+@Hf-MOF after treatment with 3-PBA and 3-PBD under the excitation of 322 nm, where the dashed line shows the threshold. The inset shows the corresponding optical photograph. Reproduced with permission from ref. 76d Copyright 2020, The Royal Society of Chemistry. | |
8 The photo-induced energy transfer (PET) and fluorescence (Förster) resonance energy transfer (FRET) for luminescence response upon chemical sensing in lanthanide-functionalized MOF hybrids (LnFMOFH)
The common mechanistic pathways for MOF materials in luminescence sensing mainly involve two pathways, namely photo-induced electron transfer (PET) and fluorescent (Förster) resonance energy transfer (FRET). Especially, they occur for the majority of MOFs with LC luminescence or all types of CT. The energy transfer process mainly focuses on the probe (often molecule system and linkers of MOFs) and the analytes (especially molecular species). In this regard, the character of MOF sensing is identical to small molecule systems. FRET is the non-radiative transfer of energy from a donor to an acceptor molecule, which is also distance dependent, and hence can be used to investigate the coulombic interaction between molecules. On the other hand, PET is similar to DEE, which involves the transmission of an excited electron from a donor to acceptor via a non-radiative pathway; however, this is a redox process and results in luminescence quenching. Tuning of the HOMO and LUMO energy levels by complexation of the analyte with probe may be reflected in the luminescence energy and intensity of the probe. This is relevant to MC luminescence, especially for lanthanide-based sensors having spin-forbidden f–f transitions, and hence difficult to excite directly.38c However, neither PET nor FRET is common in MOFs materials, and even rarer in LnFMOFH materials.
PET for luminescence response upon chemical sensing.
Herein several recent works on PET for MOF-based materials are introduced. Yang et al. synthesized two LnMOFs, which could absorb the carboxyfluorescein (FAM)-tagged probe DNA (P-DNA) and quench the luminescence of FAM via the PET process. Thus, the non-emissive P-DNA@MOF hybrids formed functioned as sensing platforms to distinguish conservative linear, single-stranded RNA sequences of Sudan virus with high selectivity and LOD of 112 and 67 pM, respectively.77a Wang et al. designed and synthesized two ZrMOFs, which showed intense luminescence in water and could be solely quenched by trace amounts of Fe3+ ions with an LOD toward Fe3+ ions of 212 and 16 ppb, respectively. The efficient luminescent quenching effect is attributed to the PET between the Fe3+ ions and the ligands in these MOFs.77b Bej et al. synthesize Cd2+- and Zn2+-based MOFs for the selective identification of m-xylene in a pool of other isomers via fluorometric methods. Inhibition of the PET process is the prime reason for the luminescence enhancement owing to the comparable molecular orbital energies of m-xylene in comparison with that of o- and p-xylene.77c Fan et al. designed an unprecedented 3D self-penetrated Zn-MOF as a multi-response chemo-sensor for the detection of dichloronitroaniline pesticide and nitrofuran antibiotics in water with the LOD of 116 ppb for DCN pesticide, 16 ppb for NFT antibiotic, and 12 ppb for NTZ antibiotic. Besides, the mechanisms of the luminescence quenching were revealed from the viewpoint of IFE and PET.77d Tang et al. developed a hybrid via PSM of a ZrMOF through an efficient click reaction with 1-ethynylpyrene for the detection of 2,4,6-trinitrophenol (PA). The hybrid showed an 85% luminescence quenching efficiency with 0.203 mMPA and the LOD of 4.5 × 10−7 M. The hydrogen bond interaction promotes the PET between the MOF-based hybrid and PA, leading to better selectivity for PA detection over TNT.77e
Lian et al. used a hetero bimetallic-organic framework (Ag+/Tb3+@MOFs) and mercaptan linker to build a versatile platform for sensing and decontaminating CS lachrymator.78 MOF-SH was used as the detector for CS based on the thiolene reaction. The photos of these suspensions under UV light also displayed obvious luminescence extinction from bright green to dark green (Fig. 19a (top)(left)), which exhibited conspicuous significant luminescence quenching effects. However, the luminescence of Ag+/Tb3+@MOFs was maintained even upon contact with the CS molecule. This quenching effect of the emission of CS was clearly observed, indicating that MOF-SH can be considered as a promising specific luminescent probe for the detection of the CS toxicant. Concentration-dependent studies on the photoluminescence were carried out, which revealed a good linear relationship (R2 = 0.9987) between the logarithm of the intensity ratio and molar concentration of CS in aqueous solutions (Fig. 19b (top)(right)) over the range of 1.0 × 10−7 to 5.0 × 10−5 mol L−1 with an LOD of 32.1 ppb. This can be attributed to the chemical bond between the CS molecule and MOF-SH framework. For Ag+/Tb3+@MOFs and MOFs-SH (Fig. 19a (bottom)), this aspect can play an important role, where the organic ligand bpydc has excited states in a region relevant for LMET to Tb3+. Hence, the interdiction of the emission was confirmed, and the PET pathway was proposed (Fig. 19b and c (bottom)). Specifically, for the original MOF, the electrons in the HOMO are excited to the LUMO, and subsequently go back to the HOMO via a transition and accompanied by luminescence. When structures with high HOMO levels appear around Ag+/Tb3+@MOF, such as CS-SH, the electrons in the HOMO of CS-SH will be transferred to the empty HOMO of Ag+/Tb3+@MOF, resulting in forbidden electronic transitions, and thus no luminescence can be observed. The PET process will occur in the resulting structure of MOF-SH with CS.
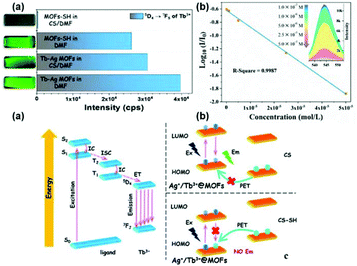 |
| Fig. 19 (Top) (a) Histogram for the emission of the 5D4 → 7F5 transition of Ag+/Tb3+@MOFs or MOF-SH in pure DMF or CS/DMF solution. (b) Linear relationship curve between log10(Ii/I0) with concentration of CS in water and the partial spectra between 535–555 nm (insert). (Bottom) (a) Jablonski diagram for the ligand and Tb3+. (b and c) Schematic diagram of the HOMO and LUMO of different materials or structures, and scheme of the PET pathway, Ex = excitation, and Em = emission. Reproduced with permission from ref. 78 Copyright 2019 Elsevier. | |
FRET for luminescence response upon chemical sensing.
For the FRET mode of sensing, there are some reports on MOF probes. Here, only some comments are presented on the typical works in the last two years.79,80 Ding et al. prepared two types of luminescent ZrMOFs as energy donor and stimuli-responsive polymers conjugated to fluorophores as energy acceptors, forming a FRET nanosystem. The ratiometric sensing of pH (3.0 and 8.0 under 420 nm excitation and by tuning the ratio of the emission peaks at 645 and 530 nm) and temperature (25 °C to 50 °C under 550 nm excitation and by tuning the ratio of the emission peaks at 810 and 695 nm) was accomplished by monitoring the luminescence.79a Afzalinia et al. reported a luminescent biosensor based on the “sandwich-type” hybridization of oligonucleotides and the FRET strategy to determine the expression levels of microRNA-155 (miRNA-155) as a cancer biomarker. A modified La3+-MOF and Ag NPs were used as the energy donor–acceptor pairs for luminescence quenching through the FRET process, and the surface of both was conjugated with different 5′-amino-labeled ssDNA strands (aptamers).79b Qu et al. designed a luminescence sensor platform by the interaction of aggregated/dispersed Au NPs with Tb-MOFs. AuNPs can effectively quench the luminescence of Tb-MOFs, involving FRET and IFE and the dynamic quenching effect (DQE). The linear range of PSA was achieved in the range 1 to 100 ng mL−1 with a detection limit of 0.36 ng mL−1.79c Sun et al. reported a thiol-labeled adenosine triphosphate (ATP) binding aptamer covalently linked on the surface of Au NPs. The dispersed AuNPs could quench the luminescence of the Tb-MOFs at 547 nm with the excitation wavelength of 290 nm, which is similarly ascribed to the combined action of FRET and IFE.79d Cao et al. reported the FRET properties of a 2D Cd-MOF, which was encapsulated with two guest dyes, acridine orange (AO) and rhodamine B (RhB), in its honeycomb-type nanochannel. Cd-MOF serves as an energy funnel, which harvests high energy excitation and channels it onto AO and then onto RhB.80a Khatun et al. constructed a luminescent pillared paddle wheel ZnMOF, and its highly ordered arrangement and good overlap between the emission and absorption spectra of these two complementary energy donor and acceptor units enabled ligand-to-ligand FRET, allowing the MOF to display exclusively DPTTZ-centric blue emission (410 nm) regardless of the excitation of the chromophores at different wavelengths. ZnMOF displayed an Hg2+-specific luminescence signal, demonstrating the selective detection of Hg2+.80b Cai et al. studied a series of dye@ZnMOF, featuring a unique mechanism of tandem FRET among the MOF host and multiple dye guests.80c Xiao et al. synthesized a Cd-MOF and fabricated an unparalleled luminescence-silent system CrO42−@Cd-MOFs by PSM. Firstly, this probe quenched the luminescence intensity of CrO42− ions, originating from the FRET mechanism. Further, the fluorescent intensity of the CrO42−@Cd-MOF system could be recovered for sensing ascorbic acid due to the elimination of the FRET process.80d
For LnFMOFH, Hao et al. firstly report the design of a luminescent Eu3+@GaMOF sensor, the luminescence of which could be effectively quenched by 1-hydroxypyrene (1-HP) via a FRET process, thus achieving the recognition of 1-HP.81a As shown in Fig. 20a (top), upon the addition of 1-HP (1 mg mL−1) to the suspension of Eu3+@GaMOF, a significant decrease (77%) in the luminescence intensity of Eu3+ at 614 nm was observed, leading to the emission color of the hybrid changing from red to colorless. Notably, the fluorescent response rate of the hybrid probe toward 1-HP was very fast, as illustrated by the time-dependent PL spectra and intensity curve (Fig. 18b (top)). The interaction between 1-HP and the MOF linker caused the luminescence quenching. The LUMO energy level of the ligands (donor) is obviously lower than that of 1-HP (acceptor). This indicates the absence of the PET process in the luminescence quenching by 1-HP. The other mechanism for the luminescence quenching is the FRET process between the donor and acceptor. The efficiency of FRET depends the extent of spectral overlap between the donor emission spectrum and the acceptor absorption spectrum. The donor–acceptor (ligand-1-HP) distance (r) and Förster distance (R0) in this study were also calculated. This also facilitates the occurrence of the FRET process between the ligands of 1a and 1-HP. The bright red emission of 1a is based on the ligand-to-metal energy transfer (LMET) process, where the ligands strongly absorb UV light and transfer the absorbed energy to Eu3+, and thus facilitates the luminescence of Eu3+ and endows 1a with the characteristic emission of Eu3+ (Fig. 20 (bottom)). However, in the presence of 1-HP, the FRET process between the ligands and 1-HP competes with the LMET process by suppressing or blocking the energy transfer from the ligands to Eu3+. Consequently, the emission of 1a is restricted or quenched, and thus 1-HP is detected (20 (bottom)).
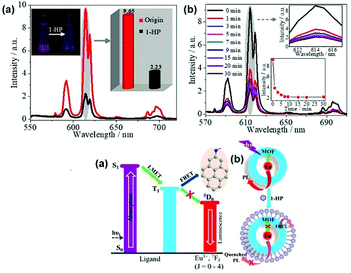 |
| Fig. 20 (Top) (a) Suspension-state luminescence spectra of Eu3+@GaMOF before and after treatment with 1-HP (1 mg mL−1). Inset: changes in the emission intensity (614 nm) and luminescence colors of Eu3+@GaMOF induced by the addition of 1-HP. (b) Emission spectra of Eu3+@GaMOF suspensions upon exposure to 1-HP at various intervals (λex = 302 nm) and the emission intensity at 614 nm as a function of exposure time in the inset. (Bottom) (a) Simplified scheme of the LMET and FRET processes in Eu3+@GaMOF with 1-HP. (b) Schematic illustration of the mechanism of 1-HP sensing by Eu3+@GaMOF. Reproduced with permission from ref. 81a Copyright 2017, WILEY-VCH. | |
Lian et al. fabricated a sandwiched mixed matrix membrane (MMM) based on an MOF-hydrogel hybrid and achieved ppb-level sensitivity and good selectivity for the detection of mitoxantrone in serum among other analogous antineoplastics.81b The MMM in mitoxantrone solution showed a very faint emission compared to that of the original MMM (Fig. 21a (top)). This quencher-induced reduction of the luminescence of MMMs was used for the detection of mitoxantrone. As shown in Fig. 21b (top), various analytes induced different degrees of effect on the luminescence of the suspension; however, mitoxantrone exhibited the most remarkable quenching effect. There is no evidence that the interfering substances can affect the selectivity of the detection platform (Fig. 21c (top)). The ribbon hydrogel MMMs also exhibited distinct luminescence under UV radiation, which could be observed by the naked eye (Fig. 21a (bottom)). Overall, the presence of the FRET process between MMM and mitoxantrone was confirmed (Fig. 21b (bottom)). In this process, the donor is the Eu3+ luminescence center and the acceptor is the quencher mitoxantrone. The MMM strongly absorbed photons and transferred the energy to Eu3+, thus allowing MMM to display the characteristic emission of Eu3+. However, in the presence of mitoxantrone, the FRET process restrained the energy transfer from MMM to Eu3+ (Fig. 21c (bottom)).
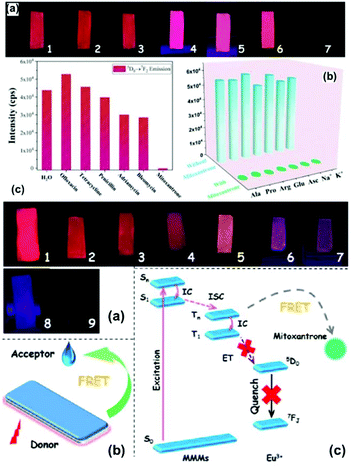 |
| Fig. 21 (Top) (a) Photographs of MMM hydrogels under UV irradiation after the addition of H2O (1), ofloxacin (2), tetracycline (3), penicillin (4), adriamycin (5), bleomycin (6) and mitoxantrone (7). (b) Luminescence intensity of MMMs at 614 nm toward various drugs. (c) Luminescence responses of MMMs toward mitoxantrone in the presence of other various serum components. (Bottom) (a) Photographs of MMMs after the addition of serum with different concentrations of mitoxantrone (1–9: 10−3–100 μM). (b) and (c) FRET process between MMMs and mitoxantrone. Reproduced with permission from ref. 81b Copyright 2020, the American Chemical Society. | |
9. Special interactions for luminescence response upon chemical sensing in lanthanide-functionalized MOF hybrids (LnFMOFH)
Besides the general metal ions and linkers or ligands in MOF materials, there are still some special interactions affecting the luminescence response to be applied in sensing. They include the hydrogen bonding or π → π interactions in the MOF host or even some reactions (coordination, redox, precipitation, etc.) between the MOF building blocks and analytes.82–87 Although these interactions do not seem to be very common, they still provide useful and attractive significance in this field.
Hydrogen bonding for luminescence response upon chemical sensing.
Comparatively, some research on hydrogen bonding in luminescent responsive sensing have been reported. Here, this is extended to some works on MOF materials.82,83 Kahn et al. synthesized a ZnMOF, and its luminescence and electron-rich nature were utilized for the selective sensing of Fe3+ and 2,4,6-trinitrophenol (TNP) in water with detection limits of 3.7 and 1.8 ppm, respectively. The mechanistic evidence revealed that a combination of strong hydrogen bonding with FRET and PET processes is synchronously responsible for the quenching of the luminescence intensity of Zn-MOF.82a Zhu et al. used an amino-group functionalized MOF NH2-UiO-66 to obtain a drastic luminescence enhancement at 455 nm induced by F− ions with an LOD of 0.229 mg L−1. Its luminescent sensing mechanism can be ascribed to the formation of hydrogen bonds between the guest F− anions and free amino groups in NH2-UiO-66, resulting in incremental electron transfer from the ligands to Zr-oxo clusters.82b Sharma et al. reported the synthesis of a hydroxyl-functionalized MOF for the rapid and efficient sensing of NACs, and examined in detail its luminescence quenching mechanisms. In chloroform, quenching occurs primarily by exciton migration to the ground-state complex formed between the MOF and the analytes. A combination of hydrogen-bonding interactions and π–π stacking interactions are responsible for luminescence quenching, and this observation was supported by single crystal structures.83c Liu et al. revealed the underlying principles for the sensing mechanism by comprehensively studying the analyte–sensor interactions. They discovered that the hydrogen bond shows multi-functions during the detection process, which, on the one hand, serves as the electron transfer bridge, and on the other hand, reinforces the π–π stacking.82d Tehrani et al. synthesized two urea-containing MOFs (namely TMU-35 and TMU-36), whose urea functional groups can interact favorably through hydrogen bonding with carbonyl compounds, and thus they are potential candidates for sensing carbonyl compounds. When the pillaring linker containing a benzene core (TMU-35) was replaced by a pillaring linker containing a naphthalene core (TMU-36), the luminescence sensing ability towards carbonyl compounds was dramatically quenched.83a Pentyala et al. synthesized a ZnMOF with specific binding sites for guest molecules, and its sensing interaction with alcohol gas was studied. It was argued that the sensing mechanism is based on the exchange of Zn-coordinated water against alcohol molecules. The unfavourable loss of hydrogen bonds when replacing water with alcohol in the structure can be partially balanced by van der Waals interactions between the alcohol alkyl chains and the aromatic rings of the linker molecules.83b Mallick et al. reported a luminescent Zr-based MOF, which exhibited an unprecedented low LOD of 66 nM for amines in aqueous solution. Markedly, this ultralow detection was driven by hydrogen bonding interactions between the linker and the host amines.83c
For LNFMOFH materials sensing via hydrogen bonding interaction, Hao et al. designed an Eu3+ PSM GaMOF hybrid (Eu3+@GaMOF). It could selectively and sensitively detect ammonia gas and simultaneously realize the determination of the biological metabolite of ammonia in human body (urinary urea), which represents a rare example of a reported luminescent senor that can realize both the environmental monitoring of pollutants and detection biological of indicators (scheme in Fig. 22 (top)).84 The solid-state luminescent measurements are presented and compared in Fig. 22 (middle). Interestingly, most organic vapors had a negligible effect on the luminescence of the hybrid film, except in the case of NH3, which induced a remarkable reduction (76%) in the luminescence intensity of Eu3+ at 614 nm. In accordance with the change in the luminescence spectra, under the irradiation of a UV lamp, only the hybrid film with absorbed NH3 showed significantly darker luminescence than that of the original film (inset of Fig. 22b (middle)). More importantly, the hybrid also realized the determination of the biological metabolite (urinary urea) of inhaled ammonia gas in the human body. For the suspension-state fluorescence in Fig. 22a (bottom), notably, only urea induced a 2-fold enhancement in the emission intensity at 614 nm. They tentatively explored the mechanism of the luminescence response to ammonia and urea. As a sensor for both ammonia and urea, it can be simply and quickly regenerated, indicating that the interactions between ammonia or urea and the ligands are the hydrogen bonds between ammonia or urea and the ligands affect the energy transfer efficiency from the ligand to Eu3+ ions since the luminescence intensity of Ln3+ crucially depends on the efficiency of the ligand to Ln energy transfer. In the presence of ammonia, the ligand emission exhibited a bathochromic shift of 24 nm, indicating a decrease in the π* energy level of the ligands by the hydrogen bonds. On the contrary, urea induced a hypochromic shift (27 nm) in the emission of the ligands, demonstrating an increase in the energy level of the π* orbitals of the ligands by the hydrogen bonds. Thus, the sensing mechanism for ammonia or urea is based on the hydrogen bonds interaction with the ligands, which changes the excited-state energy level of the ligands, thus affecting the ligand-to-Ln energy transfer efficiency, and consequently leads to different luminescent emissions.
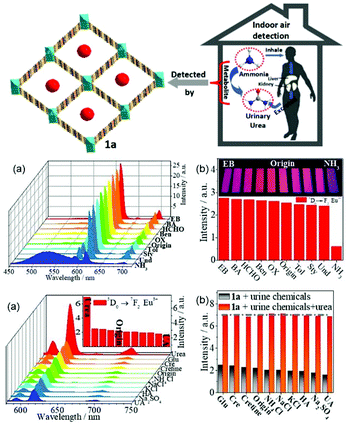 |
| Fig. 22 (Top) Schematic of the synthesis of Eu3+@GaMOF nanocrystal as a luminescent sensor for indoor ammonia and its biological metabolite (urinary urea) in the human body. (Middle) (a) PL spectra of Eu3+@GaMOF film after exposure to various indoor gas pollutants for 1 h. (b) Relative emission intensities at 614 nm. (λex = 338 nm), and the inset shows the corresponding photographs under 365 nm UV-light irradiation. (Bottom) (a) Suspension-state PL spectra and the relative intensities of the 5D0 → 7F2 transition at 614 nm (inset of a) for Eu3+@GaMOF dispersed in various urine chemicals (10−2 M, λex = 338 nm). (b) Luminescence responses of Eu3+@GaMOF (1 mg mL−1) upon the addition of urea (0.01 M) in the presence of various urine chemicals (0.02 M) in aqueous solution (λex = 338 nm). Reproduced with permission from ref. 84 Copyright 2016, The RSC. | |
Coordination interaction for luminescence response upon chemical sensing.
Lian et al. prepared an Eu3+-functionalized Sc-MOF as a “Turn-On” fluorescent switch for phenylglyoxylic acid (PGA) for the coordination interaction between Eu3+ and PGA.85 The Eu-functionalized Sc-MOF was constructed based on the following reasons: (a) the carboxyl-rich MOF synthesized by the mix-ligand strategy can effectively sensitize the luminescence of Eu3+ through the LMCT process and (b) in the presence of PGA, the emission of Eu-functionalized MOF (Eu3+@ScMOF) is rapidly and greatly enhanced. Moreover, the on-site monitoring of PGA in urine by a paper-based fluorescent probe integrated with a smartphone was also proposed (Fig. 23 (top)). Compared with the excitation spectrum of the original Eu3+@MOF (Fig. 23a (bottom)), the maximum excitation peak in the spectrum of Eu3+@ScMOF with PGA shifted to 322 nm, and the intensity of the maximum excitation peak was much stronger than the original spectrum under the same experimental conditions. As shown in Fig. 23b (bottom), only PGA could induce a significant enhancement in the luminescence of Eu3+, indicating that Eu3+@MOF can be a potential sensor with excellent selectivity for PGA in an aqueous environment. The luminescence changes of Eu3+@MOF suspensions over time were recorded by successive photographs (Fig. 23c (bottom)). The luminescence enhancement is presented in Fig. 23a (bottom), and the calculated LOD value was 4.16 ppb. The lifetime of the Eu3+@MOF suspension with PGA was much larger than the others due to the interaction between Eu3+ and the PGA molecules. A new complex structure was formed in the suspension of Eu3+@MOF with PGA. The diketone group in the PGA molecule is known excellent structure for Eu3+ sensitization. The appropriate interatomic distance is also favourable for this speculation. The calculated results based on DFT revealed that the possible complex structure is stable in the suspension system.
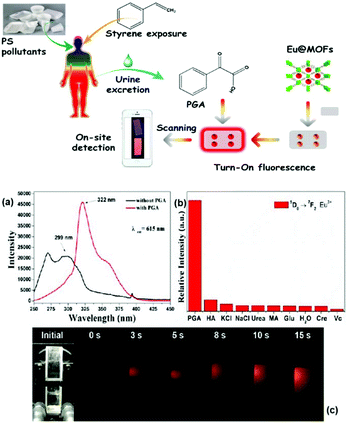 |
| Fig. 23 (Top) Schematic diagram of the “Turn-On” luminescence switch for the on-site detection of urinary PGA. (Bottom) (a) Excitation spectra of Eu3+@ScMOF with or without PGA. (b) Histogram for the luminescence of Eu3+@ScMOF (5D0 → 7F2 transition of Eu3+) in different urine chemicals under excitation at 322 nm. (c) Successive photographs of Eu3+@ScMOF with the addition of PGA solution for different intervals (0 s–15 s). Reproduced with permission from ref. 85 Copyright 2017, Elsevier. | |
Reduction reaction for luminescence response upon chemical sensing.
Hao et al. fabricated an Eu3+/Ag+-functionalized MOF hybrid (Eu3+/Ag+@ZrMOF) as a luminescent sensor for the detection of indoor formaldehyde (FA). The implantation of Ag+ ions allowed the material to simultaneously exhibit the luminescence of both Eu3+ and the organic ligands in the MOF to form a dual-emitting hybrid.86 As shown in Fig. 24 (top), the sensing of FA by the Ag+/Eu3+ co-doped MOF was achieved by its induced emission change. The pure Eu3+@ZrMOF only exhibited the characteristic emission of Eu3+. In the presence of Ag+, the Ag+/Eu3+ co-doped MOF also exhibited the emission of the organic ligands accompanied by a decrease in the emission of Eu3+ since Ag+ changes the electronic structure of the organic ligands and affects the ligand-to-Eu energy transfer process. The energy gap between S1 and T1 of the organic ligands was calculated to be 12
300 cm−1. This high energy gap (>5000 cm−1) is favorable for the intersystem crossing process (Fig. 24a (bottom)) and gives the emission of organic ligands. In contrast, the energy gap between the T1 (21
598) and 5D0 level of Eu3+ (17
500 cm−1) exceeds the optimal ΔE (∼2500 cm−1) due to the presence of Ag+, weakening the ligand-to-Eu energy transfer efficiency and decreasing the emission of Eu3+. FA has the tendency to interact with the Ag+ in hybrids, thus affecting their electronic structure and energy transfer process. Accordingly, the sensing of FA is achieved by its redox reaction with Ag+ and the induced luminescence change in the hybrid (Fig. 24b (bottom)).
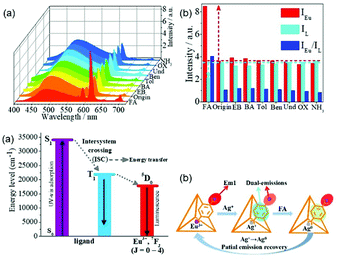 |
| Fig. 24 (Top) Luminescence spectra (a) and intensities (IEu, IL, and IEu/IL) (b) of Eu3+/Ag+@ZrMOF films after treatment with various indoor pollution gases. (Bottom) (a) Simplified schematic diagram of the LMET process in Eu3+/Ag+@ZrMOF. (b) Schematic illustration of the mechanism of FA sensing by Eu3+/Ag+@ZrMOF. Reproduced with permission from ref. 86 Copyright 2016, The RSC. | |
Precipitation reaction for luminescence response upon chemical sensing.
Xu et al. selected two LnMOFs to provide high color purity and accurate measurements, where Ag+/Eu3+@UiO-67 was the working luminescence center and TbMOF was the reference luminescence center.87 The coordinated Ag+ reduced the efficiency of the energy transfer from UiO-67 to Eu3+, which was proven by the quantum yields (4%) and luminescence lifetime study (undetectable) (Fig. 25 (top)). The reduced lifetime indicates a dynamic quenching mechanism and that the quenching is due to the interaction of Ag+ and the excited ligand molecule. Considering the strong affinity between Ag+ and Cl− to cause a precipitation reaction, they examined the potential of Ag+/Eu3+@UiO-67 for the detection of Cl−. As shown in Fig. 25a–c (bottom), the presence of Cl− triggered an apparent enhancement in the Eu3+-based emission (24.6-fold for 614 nm). Under a UV lamp, a bright red color could be observed. By integrating DUT-101 with Ag+/Eu3+@UiO-67, a luminescence sensor to monitor Cl− could be developed using Ag+/Eu3+@UiO-67 for signal processing and DUT-101 for signal calibration. In contrast to Cl−, a color conversion could not be observed for any of the added components under a 365 nm UV lamp (inset of Fig. 25d (bottom)). The emission spectra are shown in Fig. 25e (bottom), which reflect the Cl− sensor response to artificial sweat. Upon a gradual increase in the concentration of Cl−, the Eu3+ emission intensity progressively increased, and the Tb3+-based emission intensity remained unchanged. The luminescence colors of the sensing cotton piece changed from green to yellow, and then, orange and red as the Cl− concentration increased under 365 nm UV light irradiation. As shown in Fig. 25f (bottom), a good linear correlation was observed over the concentration range, and the relationship could be fitted with a correlation coefficient (R2) of 0.99752 with an LOD of 0.1 mM.
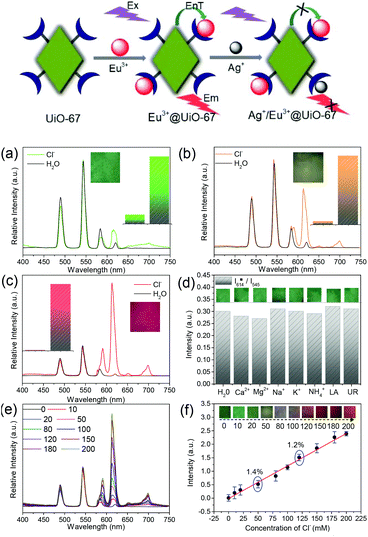 |
| Fig. 25 (Top) Simplified illustration showing the Ag+-induced luminescence quenching of Eu3+ in Ag+/Eu3+@UiO-67. (Bottom) Luminescence response of MCP-1 (a), MCP-2 (b) and MCP-3 (c) towards Cl− (1 mL, 100 mM), and the insets show the corresponding luminescence photographs and luminescence intensity of I614/I545. (d) Luminescence response of MCP-2 towards various sweat components at their physiological level, and the insets show the corresponding photographs. (e) Concentration-dependent emission spectra of MCP-2 upon the addition Cl− (0–200 mM). (f) Linear fitted curve showing the luminescence intensity of I614/I545vs. Cl− concentration for MCP-2, and the insets show the photographs for each concentration. λex = 365 nm and UV-light irradiation of 365 nm. Reproduced with permission from ref. 87 Copyright 2017, The RSC. | |
10. Conclusions
In this review, the recent research progress mainly focusing on lanthanide-functionalized metal–organic frameworks (MOF) hybrid materials (abbreviated as LnFMOFH) and their luminescence response mode and chemical sensing mechanism was summarized. These studies revealed that all types of typical luminescence response modes and sensing mechanisms can be realized in LnFMOFH materials. Firstly, the fundamental knowledge related to LnFMOFH materials and their relevant applications were introduced. Secondly, the luminescence response and luminescence sensing of LNFMOFH materials were outlined. Thirdly, the luminescence response modes in LnFMOFH materials were presented, which involve four aspects: Turn-On mode, Turn-On mode, Turn-On–Off–On mode and ratiometric mode. Fourthly, the chemical sensing mechanisms for LnFMOFH materials were concluded, focusing on the three aspects: L(Ligand)–Metal (Ln)-ET mechanism; PET and FRET mechanism; and other mechanisms based on special interactions. After inspecting all the research progress, several chief distinguishing features can be emphasized. (1) The “Turn-Off” response based on the quenching effect is the most popular for LnFMOFH materials, which covers all types of sensing mechanisms and analytes. (2) “Ratiometric luminescence (RL)” is universal for LnFMOFH for both lanthanide emission and linker or other guests. (3) The “Turn-On” response is relatively not common and mainly focuses some special analytes or a single mechanism. The “Turn-Off–On” response is an important strategy to realize the “Turn-On” mode even though they are individually strengthened. (4) Considering the nature LnFMOFH with metal ions (including functionalized Ln3+) and linkers (ligands), LMET is the most widespread mechanism for the luminescence response mode. Some special interactions including reactions also result in a luminescence response mode with an influence on the LMET process in LnFMOFH materials. (5) Both the FRET and PET mechanism are rare in LnFMOFH materials because they are mainly ascribed to the interaction between the linker of MOF unit and analytes. In fact, there are multiple luminescent responses in many LnFMOFH materials, and thus their exact mechanism and effective tuning will be of significance for practical sensing in complicated systems. Besides, it is strongly expected to realize the regular correlation between luminescence response and chemical sensing mechanism, which is favourable to design candidate sensing LnFMOFH materials for function-oriented studies in the field of luminescence responsive chemical sensing.
Conflicts of interest
There are no conflicts to declare.
Acknowledgements
This work is supported by the National Natural Science Foundation of China (21971194).
Notes and references
-
(a) S. L. James, Metal-organic frameworks, Chem. Soc. Rev., 2003, 32, 276–288 RSC
;
(b) D. J. Tranchemontagne, J. L. Mendoza-Cortés, M. O'Keeffe and O. M. Yaghi, Secondary building units, nets and bonding in the chemistry of metal-organic frameworks, Chem. Soc. Rev., 2009, 38, 1257–1283 RSC
;
(c) W. G. Lu, Z. W. Wei, Z. Y. Gu, T. F. Liu, J. Park, J. Park, J. Tian, M. W. Zhang, Q. Zhang, T. Gentle III, M. Bosch and H. C. Zhou, Tuning the structure and function of metal-organic frameworks via linker design, Chem. Soc. Rev., 2014, 43, 5561–5593 RSC
;
(d) S. Abednatanzi, P. G. Derakhshandeh, H. Depauw, F. X. Coudert, H. Vrielinck, P. Van Der Voort and K. Leus, Mixed-metal metal-organic frameworks, Chem. Soc. Rev., 2019, 48, 2535–2565 RSC
.
-
(a) H. C. Zhou and S. Kitagawa, Metal-organic frameworks (MOFs), Chem. Soc. Rev., 2014, 43, 5415–5418 RSC
;
(b) Z. L. Lin, J. Lv, M. C. Hong and R. Cao, Metal-organic frameworks based on flexible ligands (FL-MOFs): structures and applications, Chem. Soc. Rev., 2014, 43, 5867–5895 RSC
;
(c) Y. Bai, Y. B. Dou, L. H. Xie, W. Rutledge, J. R. Li and H. C. Zhou, Zr-based metal-organic frameworks: design, synthesis, structure, and applications, Chem. Soc. Rev., 2016, 45, 2327–2367 RSC
;
(d) U. Kokcam-Demir, A. Goldman, L. Esrafili, M. Gharib, A. Morsali, O. Weingart and C. Janiak, Coordinatively unsaturated metal sites (open metal sites) in metal-organic frameworks: design and applications, Chem. Soc. Rev., 2020, 49, 2751–2798 RSC
.
-
(a) A. Schoedel, M. Li, D. Li, M. O'Keeffe and O. M. Yaghi, Structures of metal-organic frameworks with rod secondary building units, Chem. Rev., 2016, 116, 12466–12535 CrossRef CAS
;
(b) S. Pullen and G. H. Clever, Mixed-ligand metal-organic frameworks and heteroleptic coordination cages as multifunctional scaffolds-a comparison, Acc. Chem. Res., 2018, 51, 3052–3064 CrossRef CAS
;
(c) C. Healy, K. M. Patil, B. H. Wilson, L. Hermanspahn, N. C. Harvey-Reid, B. I. Howard, C. Kleinjan, J. Kolien, F. Payet, S. G. Telfer, P. E. Kruger and T. D. Bennett, The thermal stability of metal-organic frameworks, Coord. Chem. Rev., 2020, 419, 213388 CrossRef CAS
.
-
(a) M. O'Keeffe and O. M. Yaghi, Deconstructing the crystal structures of metal-organic frameworks and related materials into their underlying nets, Chem. Rev., 2012, 112, 675–702 CrossRef
;
(b) X. C. Cai, Z. X. Xie, D. D. Li, M. Kassymova, S. Q. Zang and H. L. Jiang, Nano-sized metal-organic frameworks: Synthesis and applications, Coord. Chem. Rev., 2020, 417, 213366 CrossRef CAS
;
(c) S. A. A. Razavi and A. Morsali, Linker functionalized metal-organic frameworks, Coord. Chem. Rev., 2019, 399, 213023 CrossRef
;
(d) J. H. Lee, S. Jeoung, Y. G. Chung and H. R. Moon, Elucidation of flexible metal-organic frameworks: Research progresses and recent developments, Coord. Chem. Rev., 2019, 389, 161–188 CrossRef CAS
.
-
(a) D. Zhao, D. J. Timmons, D. Yuan and H. C. Zhou, Tuning the topology and functionality of metal-organic frameworks by ligand design, Acc. Chem. Res., 2011, 44, 123–133 CrossRef CAS
;
(b) Q. Q. Pang, B. B. Tu and Q. W. Li, Metal–organic frameworks with multicomponents in order, Coord. Chem. Rev., 2019, 388, 107–125 CrossRef CAS
;
(c) H. Y. Guan, R. J. LeBlanc, S. Y. Xie and Y. F. Yue, Recent progress in the syntheses of mesoporous metal-organic framework materials, Coord. Chem. Rev., 2018, 369, 76–90 CrossRef CAS
.
-
(a) S. Norbert and B. Shyam, Synthesis of metal-organic frameworks (MOFs): routes to various MOF topologies, morphologies, and composites, Chem. Rev., 2012, 112, 933–969 CrossRef
;
(b) A. Kirchon, L. Feng, H. F. Drake, E. A. Joseph and H. C. Zhou, Chem. Soc. Rev., 2018, 47, 8611–8638 RSC
;
(c) R. G. Victor, T. Sergio and M. G. Carlos, Chem. Soc. Rev., 2020, 49, 5601–5638 RSC
;
(d) L. F. Yang, S. H. Qian, X. B. Wang, X. L. Cui, B. L. Chen and H. B. Xing, Chem. Soc. Rev., 2020, 49, 5359–5406 RSC
;
(e) W. H. Guo, K. X. Zhang, Z. B. Liang, R. Q. Zou and Q. Xu, Chem. Soc. Rev., 2019, 48, 5658–5716 CAS
.
-
(a) Y. J. Cui, J. Zhang, H. J. He and G. D. Qian, Photonic functional metal-organic frameworks, Chem. Soc. Rev., 2018, 47, 5740–5785 RSC
;
(b) E. A. Dolgopolova, A. M. Rice, C. R. Martin and N. B. Shustova, Photochemistry and photophysics of MOFs: steps towards MOF-based sensing enhancements, Chem. Soc. Rev., 2018, 47, 4710–4728 RSC
;
(c) A. E. Thorarinsdottir and T. D. Harris, Metal-organic framework magnets, Chem. Rev., 2020, 120, 8716–8789 CrossRef CAS
;
(d) D. D. Wang, D. Jana and Y. L. Zhao, Metal-organic framework derived nanozymes in biomedicine, Acc. Chem. Res., 2020, 53, 1389–1400 CrossRef CAS
.
-
(a) A. F. Kateshali, S. G. Dogaheh, J. Soleimannejad and A. J. Blake, Structural diversity and applications of Ce(III)-based coordination polymers, Coord. Chem. Rev., 2020, 419, 213392 CrossRef
;
(b) A. D. Firmino, F. Figueira, J. P. C. Tome, F. A. Almeida Paz and J. Rocha, Metal-organic frameworks assembled from tetraphosphonic ligands and lanthanides, Coord. Chem. Rev., 2018, 355, 133–149 CrossRef CAS
;
(c) F. Saraci, V. Quezada-Novoa, P. R. Donnarumma and A. J. Howarth, Rare-earth metal-organic frameworks: from structure to applications, Chem. Soc. Rev., 2020, 49 10.1039/d0cs00292e
;
(d) L. Wang and Y. Chen, Luminescence-sensing Tb-MOF nanozyme for the detection and degradation of estrogen endocrine disruptors, ACS Appl. Mater. Interfaces, 2020, 12, 8351–8358 CrossRef CAS
.
-
(a) Z. H. Yan, M. H. Du, J. X. Liu, S. Y. Jin, C. Wang, G. L. Zhuang, X. J. Kong, L. S. Long and L. S. Zheng, Photo-generated dinuclear {Eu(II)}2 active sites for selective CO2 reduction in a photosensitizing metal-organic framework, Nat. Commun., 2018, 9, 3353 CrossRef
;
(b) P. Y. Wu, L. L. Xia, M. J. Huangfu, F. B. Fu, M. Q. Wang, B. X. Wen, Z. Y. Yang and J. Wang, Lanthanide-based metal-organic framework containing “V-shaped” tetracarboxylate ligands: synthesis, crystal structures, “naked-eye” luminescent detection, and catalytic properties, Inorg. Chem., 2020, 59, 264–273 CrossRef CAS
;
(c) D. F. S. Gallis, D. J. Vogel, G. A. Vincent, J. M. Rimsza and T. M. Nenoff, NOx adsorption and optical detection in rare earth metal-organic frameworks, ACS Appl. Mater. Interfaces, 2019, 11, 43270–43277 CrossRef
;
(d) M. Y. Xu, Y. L. Wang, Q. Y. Liu, Z. T. Lin and Q. Y. Liu, Lanthanide 5,7-disulfonate-1,4-naphthalenedicarboxylate frameworks constructed from trinuclear and tetranuclear lanthanide carboxylate clusters: proton conduction and selective fluorescent sensing of Fe3+, Inorg. Chem., 2020, 59, 7265–7273 CrossRef CAS
.
-
(a) M. X. Li, S. Gul, D. Tian, E. L. Zhou, Y. B. Wang, Y. D. Han, L. S. Yin and L. Huang, Erbium(III)-based metal-organic frameworks with tunable upconversion emissions, Dalton Trans., 2018, 47, 12868–12872 RSC
;
(b) M. N. Song, Y. Y. Dang, J. Dong, X. T. Zhang, S. B. Lei and W. P. Hu, Eu-based coordination polymer microrods for low-loss optical waveguiding application, Nanoscale, 2019, 11, 21061–21067 CAS
;
(c) X. G. Yang, X. Q. Lin, Y. B. Zhao, Y. S. Zhao and D. P. Yan, Lanthanide metal-organic framework microrods: colored optical waveguides and chiral polarized emission, Angew. Chem., Int. Ed., 2017, 56, 7853–7857 CrossRef CAS
;
(d) K. Krekic, D. Klintuch and R. Pietschnig, Facile access to efficiently luminescent Ln3+ phosphonic ester coordination polymers (Ln = Eu, Tb, Dy), Chem. Commun., 2017, 53, 11076–11079 RSC
.
-
(a) J. D. Einkauf, J. M. Clark, A. Paulive, G. P. Tanner and D. T. de Lill, Coordination change, lability and hemilability in metal-organic frameworks, Inorg. Chem., 2017, 56, 5544–5552 CrossRef CAS
;
(b) L. Li, Y. L. Zhu, X. H. Zhou, C. D. S. Brites, D. Ananias, Z. Lin, F. A. A. Paz, J. Rocha, W. Huang and L. D. Carlos, Visible-light excited luminescent thermometer based on single lanthanide organic frameworks, Adv. Funct. Mater., 2016, 26, 8677–8684 CrossRef CAS
.
-
(a) P. Mahata, S. K. Mondal, D. K. Singha and P. Majee, Luminescent rare-earth-based MOFs as optical sensors, Dalton Trans., 2017, 46, 301–328 RSC
;
(b) X. L. Zeng, Z. Long, X. F. Jiang, Y. J. Zhang, Q. Liu, J. Hu, C. H. Li, L. Wu and X. D. Hou, Single Bimetallic lanthanide-based metal-organic frameworks for visual decoding of a broad spectrum of molecules, Anal. Chem., 2020, 92, 5500–5508 CrossRef CAS
;
(c) X. J. Li, S. Y. Zhou, S. Lu, D. T. Tu, W. Zheng, Y. Liu, R. F. Li and X. Y. Chen, Lanthanide metal-organic framework nanoprobes for the in vitro detection of cardiac disease markers, ACS Appl. Mater. Interfaces, 2019, 11, 43989–43995 CrossRef CAS
;
(d) D. Zhao, X. Han, S. Wang, J. W. Liu, Y. T. Lu and C. X. Li, 808 nm-Light-excited near-infrared luminescent lanthanide metal-organic frameworks for highly sensitive physiological temperature sensing, Chem. – Eur. J., 2020, 26, 3145–3151 CrossRef CAS
.
-
(a) H. Xu, C. S. Cao, J. Z. Xue, Y. Xu, B. Zhai and B. Zhao, A cuprous/lanthanide-organic framework as the luminescent sensor of hypochlorite, Chem. – Eur. J., 2018, 24, 10296–10299 CrossRef CAS
;
(b) S. Y. Wu, Y. N. Lin, J. W. Liu, W. Shi, G. M. Yang and P. Cheng, Rapid detection of the biomarkers for carcinoid tumors by a water stable luminescent lanthanide metal-organic framework Sensor, Adv. Funct. Mater., 2018, 28, 1707169 CrossRef
;
(c) W. Liu, X. Huang, C. Y. Chen, C. Xu, J. X. Ma, L. Z. Yang, W. J. Wang, W. Dou and W. S. Liu, Function-Oriented: The construction of lanthanide MOF luminescent sensors containing dual-function urea hydrogen-bond sites for efficient detection of picric acid, Chem. – Eur. J., 2018, 25, 1090–1097 CrossRef
;
(d) C. X. Zhao, X. P. Zhang, Y. Shu and J. H. Wang, Europium-pyridinedicarboxylate-adenine light-up fluorescence nanoprobes for selective detection of phosphate in biological fluids, ACS Appl. Mater. Interfaces, 2020, 12, 22593–22600 CrossRef CAS
.
-
(a) J. D. Evans, C. J. Sumby and C. J. Doonan, Post-synthetic metalation of metal-organic frameworks, Chem. Soc. Rev., 2014, 43, 5933–5951 RSC
;
(b) T. Islamoglu, S. Goswami, Z. Y. Li, A. J. Howarth, O. K. Farha and J. T. Hupp, Postsynthetic tuning of metal-organic frameworks for targeted applications, Acc. Chem. Res., 2017, 50, 805–813 CrossRef CAS
.
-
(a) K. K. Tanabe and S. M. Cohen, Postsynthetic modification of metal-organic frameworks—a progress report, Chem. Soc. Rev., 2011, 40, 498–519 RSC
;
(b) S. M. Cohen, Modifying MOFs: new chemistry, new materials, Chem. Sci., 2010, 1, 32–36 RSC
;
(c) S. M. Cohen, Postsynthetic methods for the functionalization of metal-organic frameworks, Chem. Rev., 2012, 112, 970–1000 CrossRef CAS
;
(d) S. M. Cohen, The postsynthetic renaissance in porous solids, J. Am. Chem. Soc., 2017, 139, 2855–2863 CrossRef CAS
.
-
(a) Y. Zhou and B. Yan, Imparting tunable and white-light luminescence to a nanosized metal-organic framework by controlled encapsulation of lanthanide cations, Inorg. Chem., 2014, 53, 3456–3463 CrossRef CAS
;
(b) Y. Lu and B. Yan, A novel visible and near infrared luminescent monolayer thin film based on postsynthetic method and functional linker, J. Mater. Chem. C, 2014, 2, 5526–5532 RSC
;
(c) Y. Zhou and B. Yan, Lanthanides post-functionalized nanocrystalline metal-organic frameworks for tunable white-light emission and orthogonal multi-readout thermometry, Nanoscale, 2015, 7, 4063–4069 RSC
.
- S. Y. Zhu and B. Yan, A novel sensitive fluorescent probe of S2O82− and Fe3+ based on covalent post-functionalization of zirconium(IV) metal-organic framework, Dalton Trans., 2018, 47, 11586–11592 RSC
.
-
(a) R. M. Abdelhameed, L. D. Carlos, A. M. S. Silva and J. Rocha, Chem. Commun., 2013, 49, 5019–5021 RSC
;
(b) X. Lian and B. Yan, Multi-component luminescent lanthanide hybrids of both functionalized IR-MOF-3 and SBA-15, New J. Chem., 2015, 39, 5898–5901 RSC
;
(c) S. Y. Zhu and B. Yan, Ind. Eng. Chem. Res., 2018, 57, 16564–16571 CrossRef CAS
;
(d) R. M. Abdelhameed, D. Ananias, A. M. S. Silva and J. Rocha, Luminescent nanothermometers obtained by post-synthetic modification of metal-organic framework MIL-68, Eur. J. Inorg. Chem., 2019, 1354–1359 CrossRef CAS
.
-
(a) X. Shen and B. Yan, Anionic metal-organic framework hybrids: double functionalization with lanthanides/cationic dyes and fluorescent sensing small molecules, RSC Adv., 2016, 6, 28165–28170 RSC
;
(b) X. Shen and B. Yan, A novel fluorescence probe for sensing organic amine vapors from Eu3+β-diketonate functionalized bio-MOF-1 hybrid system, J. Mater. Chem. C, 2015, 3, 7038–7046 RSC
;
(c) Y. H. Zhang, B. Li, H. P. Ma, L. M. Zhang and Y. X. Zheng, Rapid and facile ratiometric detection of an anthrax biomarker by regulating energy transfer process in bio-metal-organic framework, Biosens. Bioelectron., 2017, 85, 287–293 CrossRef
;
(d) J. Pan, D. Zhang, M. M. Shang, Y. Mu, S. D. Han and G. M. Li, An anionic Cd-based coordination polymer exhibiting ion-exchange behavior for photoluminescence and selective dye adsorption, J. Lumin., 2019, 210, 70–74 CrossRef CAS
.
-
(a) T. W. Duan and B. Yan, Hybrids based on lanthanide ions activated yttrium metal organic frameworks: functional assembly, polymer film preparation and luminescence tuning, J. Mater. Chem. C, 2014, 2, 5098–5104 RSC
;
(b) H. S. Jena, A. M. Kaczmarek, C. Krishnaraj, X. Feng, K. Vijayvergia, H. Yildirim, S. N. Zhao, R. Van Deun and P. Van Der Voort, White light emission properties of defect engineered metal-organic frameworks by encapsulation of Eu3+ and Tb3+, Cryst. Growth Des., 2019, 19, 6339–6350 CrossRef CAS
;
(c) G. Liu, Y. K. Lu, Y. Y. Ma, X. Q. Wang, L. Hou and Y. Y. Wang, Syntheses of three new isostructural lanthanide coordination polymers with tunable emission colours through bimetallic doping, and their luminescence sensing properties, Dalton Trans., 2019, 48, 13607–13613 CAS
;
(d) J. Q. Liu, L. Pei, Z. G. Xia and Y. Xu, Hierarchical accordion-like lanthanide-based metal-organic frameworks: solvent-free syntheses and ratiometric luminescence temperature-sensing properties, Cryst. Growth Des., 2019, 19, 6586–6591 CrossRef CAS
.
-
(a) C. Liu and B. Yan, Photofunctional nanocomposites based on the functionalization of metal-organic framework by up/downconverion luminescent nanophosphors, New J. Chem., 2015, 39, 1125–1131 RSC
;
(b) Y. Kitagawa, M. Kumagai, T. Nakanishi, K. Fushimi and Y. Hasegawa, The role of pi-f orbital interactions in Eu(III) complexes for an effective molecular luminescentt thermometer, Inorg. Chem., 2020, 59, 5865–5871 CrossRef CAS
;
(c) X. F. Qiao, Z. M. Ma, L. H. Si, W. Ding and G. M. Xu, Eu complex encapsulating composition Doping metal-organic framework with a series of europium-antenna cations: Obviously improved spectral response for O2 gas via long-range energy roll-back procedure, Sens. Actuators, B, 2019, 299, 126978 CrossRef CAS
.
-
(a) M. D. Allendorf, C. A. Bauer, R. K. Bhakta and R. J. T. Houk, Luminescent metal-organic frameworks, Chem. Soc. Rev., 2009, 38, 1330–1352 RSC
;
(b) Y. J. Cui, Y. F. Yue, G. D. Qian and B. L. Chen, Luminescent functional metal-organic frameworks, Chem. Rev., 2012, 112, 1126–1162 CrossRef CAS
;
(c) W. P. Lustig and J. Li, Luminescent metal-organic frameworks and coordination polymers as alternative phosphors for energy efficient lighting devices, Coord. Chem. Rev., 2018, 373, 116–147 CrossRef CAS
.
-
(a)
B. Yan, Photofunctional Rare Earth Hybrid Materials, Springer publishers, 2017 CrossRef
;
(b) J. N. Hao, X. Y. Xu, H. H. Fei, L. C. Li and B. Yan, , Functionalization of metal-organic frameworks for photoactive materials, Adv. Mater., 2018, 30, 1705634 CrossRef
.
-
(a) Y. Lu, B. Yan and J. L. Liu, Nanoscale metal-organic framework as highly sensitive luminescent sensor for Fe2+in aqueous solution and living cell, Chem. Commun., 2014, 50, 9969–9972 CAS
;
(b) J. X. Wu and B. Yan, A postsynthetically modified MOFs hybrid as a ratiometric fluorescent sensor for anions recognition and detection, Dalton Trans., 2016, 45, 18585–18590 RSC
;
(c) N. N. Sun and B. Yan, Near-infrared emission sensitization of lanthanide cation based on Ag+ functionalized metal-organic frameworks, J. Alloys Compd., 2018, 765, 63–68 CrossRef CAS
.
-
(a) J. Z. Wang, Y. Suffren, C. Daiguebonne, S. Freslon, K. Bernot, G. Calvez, L. Le Polles, C. Roiland and O. Guillou, Multi-emissive lanthanide-based coordination polymers for potential application as luminescent barcodes, Inorg. Chem., 2019, 58, 2659–2668 CrossRef
;
(b) H. N. Abdelhamid, M. Wilk-Kozubek, A. M. El-Zohry, A. B. Gomez, A. Valiente, B. Martin-Matute, A. V. Mudring and X. D. Zou, Luminescence properties of a family of lanthanide metal-organic frameworks, Microporous Mesoporous Mater., 2019, 279, 400–406 CrossRef CAS
;
(c) S. S. Wang, J. Xu, J. Wang, K. Y. Wang, S. Dang, S. Y. Song, D. Liu and C. Wang, Luminescence of samarium(III) bis-dithiocarbamate frameworks: codoped lanthanie emitters that cover visible and near-infrared domains, J. Mater. Chem. C, 2017, 5, 6620–6628 RSC
.
-
(a) X. Q. Song, M. Zhang, C. Y. Wang, A. A. A. Shamshooma, H. H. Meng and W. Xi, Mixed lanthanide coordination polymers for temperature sensing and enhanced Nd-III NIR luminescence, J. Lumin., 2018, 201, 410–418 CrossRef CAS
;
(b) X. N. Mi, D. F. Sheng, Y. Yu, Y. H. Wang, L. M. Zhao, J. Lu, Y. W. Li, D. C. Li, J. M. Dou, J. G. Duan and S. N. Wang, Tunable light emission and multi-responsive luminescent sensitivities in aqueous solutions of two series of lanthanide metal-organic frameworks based on structurally related ligands, ACS Appl. Mater. Interfaces, 2019, 11, 7914–7926 CrossRef CAS
;
(c) T. C. Sun, P. Wang, R. Q. Fan, W. Chen, S. Hao and Y. L. Yang, Functional microscale single- phase white emission lanthanide MOF for tunable fluorescent sensing and water quality monitoring, J. Mater. Chem. C, 2019, 7, 3598–3606 RSC
.
-
(a) S. L. Anderson, D. Tiana, C. P. Ireland, G. Capano, M. Fumanal, A. Gladysiak, S. Kampouri, A. Rahmanudin, N. Guijarro, K. Sivula, K. C. Stylianou and B. Smit, Taking lanthanides out of isolation: tuning the optical properties of metal-organic frameworks, Chem. Sci., 2020, 11, 4164–4170 RSC
.
-
(a) F. Artizzu, M. Atzori, J. Liu, D. Mara, K. Van Hecke and R. Van Deun, Solution-processable Yb/Er 2D-layered metal-organic frameworks with high NIR-emission quantum yields, J. Mater. Chem. C, 2019, 7, 11207–11214 RSC
;
(b) J. D. Einkauf, J. M. Clark, A. Paulive, G. P. Tanner and D. T. de Lill, A general model of sensitized luminescence in lanthanide-based coordination polymers and metal organic framework materials, Inorg. Chem., 2017, 56, 5544–5552 CrossRef CAS
.
-
(a) Y. S. Yang, K. Z. Wang and D. P. Yan, Lanthanide doped coordination polymers with tunable afterglow based on phosphorescence energy transfer, Chem. Commun., 2017, 53, 7752–7755 RSC
;
(b) L. F. Xu, Y. Xu, X. L. Li, Z. P. Wang, T. Sun and X. Zhang, Eu3+/Tb3+ functionalized Bi- based metal-organic frameworks toward tunable white-light emission and fluorescence sensing application, Dalton Trans., 2019, 47, 16696–16703 RSC
.
-
(a) J. D. Xiao and H. L. Jiang, Metal-organic frameworks for photocatalysis and photothermal catalysis, Acc. Chem. Res., 2019, 52, 356–366 CrossRef CAS
;
(b) H. Q. Yin and X. B. Yin, Metal-organic frameworks with multiple luminescence emissions: designs and applications, Acc. Chem. Res., 2020, 53, 485–495 CrossRef CAS
.
- H. Kaur, S. Sundriyal, V. Pachauri, S. Ingebrandt, K. H. Kim, A. L. Sharma and A. Deep, Luminescent metal-organic frameworks and their composites: potential future materials for organic light emitting displays, Coord. Chem. Rev., 2019, 401, 213077 CrossRef CAS
.
-
(a) T. Rasheed and F. Nabeel, Luminescent metal-organic frameworks as potential sensory materials for various environmental toxic agents, Coord. Chem. Rev., 2019, 401, 213065 CrossRef CAS
;
(b) Y. M. Zhang, S. Yuan, G. Day, X. Wang, X. Y. Yang and H. C. Zhou, Luminescent sensors based on metal-organic frameworks, Coord. Chem. Rev., 2018, 354, 28–45 CrossRef CAS
.
-
(a) Y. Lu and B. Yan, Lanthanide organic-inorganic hybrids based on functionalized metal-organic frameworks (MOFs) for near-UV white LED, Chem. Commun., 2014, 50, 15443–15446 RSC
;
(b) T. W. Duan and B. Yan, Lanthanide ions (Eu3+, Tb3+, Sm3+, Dy3+) activated ZnO embedded zinc 2,5-pridinedicarboxylic metal organic frameworks for luminescent application, J. Mater. Chem. C, 2015, 3, 2823–2830 RSC
.
-
(a) Y. Lu and B. Yan, Luminescent lanthanide barcodes based on postsynthetic functionalized nanoscale metal-organic frameworks, J. Mater. Chem. C, 2014, 2, 7411–7417 RSC
;
(b) Y. Zhou and B. Yan, Ratiometric multiplexed barcodes based on luminescent metal-organic framework films, J. Mater. Chem. C, 2015, 3, 8413–8318 RSC
;
(c) X. Shen and B. Yan, Barcoded materials based on photoluminescent hybrid system of lanthanide ions-doped metal organic framework and silica via ion exchange, J. Colloid Interface Sci., 2016, 468, 220–226 CrossRef CAS
.
-
(a) L. E. Kreno, K. Leong, O. K. Farha, M. Allendorf, R. P. Van Duyne and J. T. Hupp, Metal-organic framework materials as chemical sensors, Chem. Rev., 2012, 112, 1105–1125 CrossRef CAS
;
(b) S. A. A. Razavi and A. Morsali, Metal ion detection using luminescent-MOFs: Principles, strategies and Roadmap, Coord. Chem. Rev., 2020, 415, 213299 CrossRef CAS
.
-
(a) W. P. Lustig, S. Mukherjee, N. D. Rudd, A. V. Desai, J. Li and S. K. Ghosh, Metal–organic frameworks: functional luminescent and photonic materials for sensing applications, Chem. Soc. Rev., 2017, 46, 3242–3285 RSC
;
(b) I. Stassen, N. Burtch, A. Talin, P. Falcaro, M. Allendorf and R. Ameloot, An updated roadmap for the integration of metal–organic frameworks with electronic devices and chemical sensors, Chem. Soc. Rev., 2017, 46, 3185–3241 RSC
;
(c) E. A. Dolgopolova, A. M. Rice, C. R. Martin and N. B. Shustova, Photochemistry and photophysics of MOFs: steps towards MOF-based sensing enhancements, Chem. Soc. Rev., 2018, 47, 4710–4728 RSC
.
-
(a) B. L. Chen, S. C. Xiang and G. D. Qian, Metal-organic frameworks with functional pores for recognition of small molecules, Acc. Chem. Res., 2010, 43, 1115–1124 CrossRef CAS
;
(b) Z. Hu, B. J. Deibert and J. Li, Luminescent metal-organic frameworks for chemical sensing and explosive detection, Chem. Soc. Rev., 2014, 43, 5815–5840 RSC
.
-
(a) A. Karmakar, P. Samanta, A. V. Desai and S. K. Ghosh, Guest-responsive metal−organic frameworks as scaffolds for separation and sensing applications, Acc. Chem. Res., 2017, 50, 2457–2569 CrossRef CAS
;
(b) H. Wang, W. P. Lustig and J. Li, Sensing and capture of toxic and hazardous gases and vapors by metal-organic frameworks, Chem. Soc. Rev., 2018, 47, 4729–4756 RSC
;
(c) J. Q. Liu, Z. D. Luo, Y. Pan, A. K. Singh, M. Trivedi and A. Kumar, Recent developments in luminescent coordination polymers: designing strategies, sensing application and theoretical evidences, Coord. Chem. Rev., 2020, 406, 213145 CrossRef CAS
.
-
(a) Y. Zhou, H. Chen and B. Yan, Eu3+post-functionalized nanosized metal-organic framework for cation exchange-based Fe3+−-sensing in aqueous environment, J. Mater. Chem. A, 2014, 2, 13691–11369 RSC
;
(b) X. Shen and B. Yan, Photofunctional hybrids of lanthanide functionalized bio-MOF-1 for fluorescence tuning and sensing, J. Colloid Interface Sci., 2015, 451, 63–68 CrossRef CAS
;
(c) T. Rasheed and F. Nabeel, Coord. Chem. Rev., 2019, 401, 213065 CrossRef CAS
.
-
(a) X. Y. Xu and B. Yan, Eu(III)-functionalized MIL-124 as fluorescent probe for highly selectively sensing ions and organic small molecules especially for Fe(III) and Fe(II), ACS Appl. Mater. Interfaces, 2015, 7, 721–728 CrossRef CAS
;
(b) L. N. Li, S. S. Shen, W. P. Ai, S. Y. Song, Y. Bai and H. W. Liu, Facilely synthesized Eu3+ post-functionalized UiO-66-type metal-organic framework for rapid and highly selective detection of Fe3+ in aqueous solution, Sens. Actuators, B, 2018, 267, 542–548 CrossRef CAS
;
(c) X. Y. Dao and Y. H. Ni, Dalton Trans., 2017, 16, 5373–5383 RSC
;
(d) Y. Zhang and B. Yan, A ratiometric fluorescent sensor with dual response of Fe3+/Cu2+ based on europium post-modified sulfone-metal-organic frameworks and its logical application, Talanta, 2019, 197, 291–298 CrossRef CAS
.
-
(a) C. Liu and B. Yan, A novel photofunctional hybrid material of pyrene functionalized metal-organic framework with conformation change for luminescence sensing of Cu2+, Sens. Actuators, B, 2016, 235, 541–546 CrossRef CAS
;
(b) H. Weng and B. Yan, A Eu(III) doped metal-organic framework conjugated with fluorescein-labeled single-stranded DNA for decection of Cu(II) and sulphide, Anal. Chim. Acta, 2017, 988, 89–95 CrossRef CAS
;
(c) C. Liu and B. Yan, Highly effective chemosensor of luminescent silica@lanthanide complex@MOF heterostructure composite for metal ion sensing, RSC Adv., 2015, 5, 101982–101988 RSC
.
-
(a) J. N. Hao and B. Yan, A water-stable lanthanide-functionalized MOF as a highly selective and sensitive fluorescent probe for Cd2+, Chem. Commun., 2015, 51, 7737–7741 RSC
;
(b) X. Y. Xu and B. Yan, Eu(III) functionalized Zr-based metal-organic framework as excellent fluorescent probe for Cd2+detection in aqueous environment, Sens. Actuators, B, 2016, 222, 347–353 CrossRef CAS
;
(c) C. Liu and B. Yan, Zeolite-type metal organic frameworks immobilized Eu3+ for cation sensing in aqueous environment, J. Colloid Interface Sci., 2015, 459, 206–211 CrossRef CAS
.
-
(a) X. Y. Xu and B. Yan, Fabrication and application of ratiometric and colorimetric fluorescent probe for Hg2+based on dual-emissive metal-organic framework hybrids with carbon dots and Eu3+, J. Mater. Chem. C, 2016, 4, 1543–1549 RSC
;
(b) X. X. Zhang, W. J. Zhang, C. L. Li, X. H. Qin and C. Y. Zhu, Inorg. Chem., 2019, 58, 3910–3915 CrossRef CAS
.
-
(a) J. N. Hao and B. Yan, Ag+-sensitized lanthanide luminescence in Ln3+post-functionalized metal-organic framework and Ag+sensing, J. Mater. Chem. A, 2015, 3, 4788–4794 RSC
;
(b) X. Lian and B. Yan, Novel core-shell structure microspheres based on lanthanide complexes for white light emission and fluorescence sensing, Dalton Trans., 2016, 45, 2666–2673 RSC
;
(c) N. N. Sun and B. Yan, A reliable amplified fluorescence-enhanced chemosensor (Eu-MIL-61) for directional detection of Ag+ in aqueous solution, Dalton Trans., 2017, 46, 875–881 RSC
.
-
(a) J. N. Hao and B. Yan, Amino-decorated lanthanide (III) – organic extended frameworks for multi-color luminescence and fluorescence sensing, J. Mater. Chem. C, 2014, 2, 6758–6764 RSC
;
(b) S. Y. Zhu and B. Yan, Novel covalently post-synthetic modified MOF hybrids as sensitive and selective fluorescent probe for Al3+ dectection in aqueous media, Dalton Trans., 2018, 47, 1674–1681 RSC
;
(c) Y. Wang, H. Yang, G. Cheng, Y. Y. Wu and S. M. Lin, CrystEngComm, 2018, 19, 7270–7276 RSC
.
-
(a) T. W. Duan and B. Yan, Europium activated yttrium hybrid system for sensing toxic anion of Cr(VI) species, Microporous Mesoporous Mater., 2015, 217, 196–202 CrossRef CAS
;
(b) X. Shen and B. Yan, Photoactive rare earth complexes for fluorescent tuning and sensing cations (Fe3+) and anions (Cr2O72−)), RSC Adv., 2015, 5, 6752–6757 RSC
;
(c) J. X. Wu and B. Yan, Eu(III)-functionalized In-MOF (In(OH)bpydc) as fluorescent probe for highly selectively sensing organic small moleucles and anions especially for CHCl3 and MnO4−, J. Colloid Interface Sci., 2017, 504, 197–205 CrossRef CAS
.
-
(a) J. X. Wu and B. Yan, “A novel food preservative sensor and water scavenger for NO2− base with luminescent Tb3+ functionalized metal-organic frameworks, Ind. Eng. Chem. Res., 2018, 57, 7105–7111 CrossRef CAS
;
(b) S. Y. Zhu, L. M. Zhao and B. Yan, A novel spectroscopic probe for detecting food preservative NO2−: citric acid functionalized metal-organic framework and luminescence sensing, Microchem. J., 2020, 155, 104768 CrossRef CAS
.
-
(a) G. F. Ji, X. C. Gao, T. X. Zheng, W. H. Guan, H. T. Liu and Z. L. Liu, Inorg. Chem., 2018, 57, 10525–10532 CrossRef CAS
;
(b) Y. Q. Zhang, S. S. Sheng, S. Mao, X. H. Wu, Z. Li, W. Q. Tao and I. R. Jenkinson, Water Res., 2019, 163, 114883 CrossRef CAS
;
(c) N. N. Sun and B. Yan, Rapid and facile ratiometric detection of CO32− based on heterobimetallic metal-organic frameworks (Eu/Pt-MOFs), Dyes Pigm., 2017, 142, 1–7 CrossRef CAS
;
(d) H. Y. Zheng, X. Lian, S. J. Qin and B. Yan, A novel “turn-on” fluorescent probe for highly selectively sensing fluoride in aqueous solution based on Tb3+ functionalized metal-organic frameworks, ACS Omega, 2018, 3, 12513–12519 CrossRef CAS
.
-
(a) H. Weng and B. Yan, A flexible Tb(III) functionalized cadmium metal-organic framework as fluorescent probe for highly selectively sensing ions and organic small molecules, Sens. Actuators, B, 2016, 228, 702–708 CrossRef CAS
;
(b) J. N. Hao and B. Yan, Ln3+ post-functionalized metal-organic frameworks for color tunable emission and highly-sensitivity sensing of toxic anions and small molecules, New J. Chem., 2016, 40, 4646–4661 RSC
;
(c) H. Y. Zheng, X. Lian, S. J. Qin and B. Yan, Lanthanide hybrids of covalently-coordination cooperative post-functionalized metal-organic frameworks for luminescent tuning and highly-selectively sensing of tetrahydrofuran, Dalton Trans., 2018, 47, 6210–6217 RSC
.
-
(a) Y. Zhou and B. Yan, A responsive MOF nanocomposite for decoding volatile organic compounds, Chem. Commun., 2016, 52, 2265–2268 RSC
;
(b) X. Lian and B. Yan, A lanthanide metal-organic framework (MOF-76) for adsorbing dyes and fluorescence detecting aromatic pollutants, RSC Adv., 2016, 6, 11570–11576 RSC
;
(c) H. Weng and B. Yan, , N-GQDs and Eu3+ co-encapsulated anionic MOF: two-dimensional luminescent platform for decoding benzene homologues, Dalton Trans., 2016, 45, 8795–8801 RSC
;
(d) J. X. Wu and B. Yan, Europium ion post-functionalized indium metal-organic frameworks hybrid system for fluorescence detecting aromatics, Analyst, 2017, 142, 4633–4637 RSC
.
-
(a) X. Y. Xu and B. Yan, Eu(III) functionaized ZnO@MOFs heterostructures: integration of pre-concentration and efficient charge tranfer as ppb-level sensing platform for volatile aldehyde gases in vehicles, J. Mater. Chem. A, 2017, 5, 2215–2223 RSC
;
(b) Y. Zhang and B. Yan, A portable self-calibrating logic detector for gradient detecting formadehyde based on luminescent metal organic frameworks hybrids, J. Mater. Chem. C, 2019, 7, 5652–5657 RSC
;
(c) J. Min, X. L. Qu and B. Yan, Tb(III) post-functionalized La(III) metal organic framework hybrid probe for simple and highly sensitive detection of acetaldehyde, Sens. Actuators, B, 2019, 300, 126985 CrossRef CAS
.
-
(a) Z. C. Hu, B. J. Deibert and J. Li, Chem. Soc. Rev., 2014, 43, 5815–5840 RSC
;
(b) X. C. Sun, Y. Wang and Y. Lei, Fluorescence based explosive detection: from mechanisms to sensory materials, Chem. Soc. Rev., 2015, 44, 8019–8061 RSC
;
(c) T. T. Cheng, J. S. Hu, C. H. Zhou, Y. M. Wang and M. D. Zhang, Luminescent metal-organic frameworks for nitro explosives detection, Sci. China: Chem., 2016, 59, 929–947 CrossRef CAS
.
-
(a) N. S. Bobbitt, M. L. Mendonca, A. J. Howarth, T. Islamoglu, J. T. Hupp, O. K. Farha and R. Q. Snurr, Metal–organic frameworks for the removal of toxic industrial chemicals and chemical warfare agents, Chem. Soc. Rev., 2017, 46, 3357–3385 RSC
;
(b) A. M. Ploskonka and J. B. DeCoste, Insight into organophosphate chemical warfare agent simulant hydrolysis in metal-organic frameworks, J. Hazard. Mater., 2019, 375, 191–197 CrossRef CAS
.
-
(a) M. K. Yu, Y. Xie, X. Y. Wang, Y. X. Li and G. M. Li, Highly water-stable dye@Ln-MOFs for sensitive and selective detection toward antibiotics in water, ACS Appl. Mater. Interfaces, 2019, 11, 21201–21210 CrossRef CAS
;
(b) W. B. Zhong, R. X. Li, J. Lv, T. He, M. M. Xu, B. Wang, L. H. Xie and J. R. Li, Two isomeric In(III)-MOFs: unexpected stability difference and selective fluorescence detection of fluoroquinolone antibiotics in water, Inorg. Chem. Front., 2020, 7, 1161–1171 RSC
;
(c) Q. Liu, D. Ning, W. J. Li, X. M. Du, Q. Wang, Y. Li and W. J. Ruan, Metal–organic framework-based fluorescent sensing of tetracycline-type antibiotics applicable to environmental and food analysis, Analyst, 2019, 144, 1916–1922 RSC
;
(d) M. K. Yu, X. Yao, X. Y. Wang, Y. X. Li and G. M. Li, White-Llight-emitting decoding sensing for eight frequently-used antibiotics based on a lanthanide metal-organic framework, Polymers, 2019, 11, 99–104 CrossRef
;
(e) Y. Gao, J. F. Wu, J. Q. Wang, Y. X. Fan, S. Y. Zhang and W. Dai, A novel multifunctional p-type semiconductor@MOFs nanoporous platform for simultaneous sensing and photodegradation of tetracycline, ACS Appl. Mater. Interfaces, 2020, 12, 11036–11044 CrossRef
.
-
(a) K. Vikrant, D. C. W. Tsang, N. Raza, B. S. Giri, D. Kukkar and K. H. Kim, Potential utility of metal−organic framework-based platform for sensing pesticides, ACS Appl. Mater. Interfaces, 2018, 10, 8797–8817 CAS
;
(b) X. H. Xu, Y. N. Guo, X. Y. Wang, W. Li, P. P. Qi, Z. W. Wang, X. Q. Wang, S. Gunasekaran and Q. Wang, Sensitive detection of pesticides by a highly luminescentmetal-organic framework, Sens. Actuators, B, 2018, 260, 339–345 CAS
;
(c) K. Y. He, Z. S. Li, L. Wang, Y. C. Fu, H. R. Quan, Y. B. Li, X. Q. Wang, S. Gunasekaran and X. H. Xu, A water-stable luminescent metal−organic framework for rapid and visible sensing of organophosphorus pesticides, ACS Appl. Mater. Interfaces, 2019, 11, 26250–26260 CrossRef CAS
;
(d) L. Yang, Y. L. Liu, C. G. Liu, F. Ye and Y. Fu, Two luminescent dye@MOFs systems as dual-emitting platforms for efficient pesticides detection, J. Hazard. Mater., 2020, 381, 120966 CrossRef CAS
.
-
(a) J. N. Hao and B. Yan, Recyclable lanthanide-functionalized MOF hybrids to determine hippuric acid in urine as biological indices of toluene exposure, Chem. Commun., 2015, 51, 14509–144512 RSC
;
(b) X. L. Qu and B. Yan, A highly stable Tb(III)-based metal-organic framework: structure, photoluminescence and chemical sensing of 2-thiazolidinethione-4-carboxylic acid as a biomarker of CS2, Inorg. Chem., 2019, 58, 524–534 CrossRef CAS
;
(c) B. H. Wang and B. Yan, A turn-on fluorescent probe Eu3+ functionalized Ga-MOF integrated with logic gate operation for detecting ppm-level Ciprofloxacin (CIP) in urine, Talanta, 2020, 208, 120438 CrossRef CAS
;
(d) B. H. Wang, X. Lian and B. Yan, Recyclable Eu3+ functionalized Hf-MOF fluorescent probe for urinary metabolites of some organophosphorus pesticides, Talanta, 2020, 214, 120856 CrossRef CAS
.
-
(a) B. Yan, Photofunctional MOFs based hybrid materials for chemical sening on biomarkers, J. Mater. Chem. C, 2019, 7, 8155–8175 RSC
;
(b) S. J. Qin and B. Yan, The point-of care colorimetric detection of the biomarker of phenylamine in the human urine with smartphone based on Tb3+functionalized metal-organic frameworks, Anal. Chim. Acta, 2018, 1012, 82–89 CrossRef CAS
;
(c) J. X. Wu and B. Yan, Visible detection of copper ions via fluorescent probe based on red carbon dots and zirconium metal-organic frameworks, Dalton Trans., 2017, 46, 15080–15086 RSC
;
(d) D. N. Zhang, Y. Zhou, J. Cuan and N. Gan, A lanthanide functionalized MOF hybrid for ratiometric luminescence detection of an anthrax biomarker, CrystEngComm, 2018, 20, 1264–1270 RSC
.
-
(a) C. D. S. Brites, S. Balabhadra and L. D. Carlos, Lanthanide-based thermometers: at the cutting-edge of luminescence thermometry, Adv. Opt. Mater., 2019, 7, 1801239 CrossRef
;
(b) D. Zhao, D. Yue, K. Jiang, L. Zhang, C. X. Li and G. D. Qian, Isostructural Tb3+/Eu3+ co-doped metal organic framework based on pyridine-containing dicarboxylate ligands for ratiometric luminescence temperature sensing, Inorg. Chem., 2019, 58, 2637–2644 CrossRef CAS
;
(c) X. Feng, Y. P. Shang, H. Zhang, X. F. Liu, X. Y. Wang, N. Chen, L. Y. Wang and Z. J. Li, Multi-functional lanthanide-CPs based on tricarboxylphenyl terpyridyl ligand as ratiometric luminescent thermometer and highly sensitive ion sensor with turn on/off
effect, Dalton Trans., 2020, 49, 4741–4750 RSC
.
-
(a) J. F. Feng, T. F. Liu, J. L. Shi, S. Y. Gao and R. Cao, Dual-emitting UiO-66(Zr&Eu) metal-organic framework films for ratiometric temperature sensing, ACS Appl. Mater. Interfaces, 2018, 10, 20854–20861 CrossRef CAS
;
(b) Y. Zhou, D. A. Zhang, J. Zeng, N. Gan and J. Cuan, A luminescent lanthanide-free MOF nanohybrid for highly sensitive ratiometric temperature sensing in physiological range, Talanta, 2018, 181, 410–415 CrossRef CAS
;
(c) J. De Bellis, L. Bellucci, G. Bottaro, L. Labella, F. Marchetti, S. Samaritani, D. B. Dell'Amico and L. Armelao, Single-crystal-to-single-crystal post-synthetic modifications of three-dimensional LOFs (Ln = Gd, Eu): a way to modulate their luminescence and thermometric properties, Dalton Trans., 2020, 49, 6030–6042 CAS
.
-
(a) Q. G. Meng, X. L. Xin, L. L. Zhang, F. N. Dai, R. M. Wang and D. F. Sun, A multifunctional Eu MOF as a fluorescent pH sensor and exhibiting highly solvent-dependent adsorption and degradation of rhodamine B, J. Mater. Chem. A, 2015, 3, 24016–24021 RSC
;
(b) Y. J. Li, Y. L. Wang and Q. Y. Liu, The highly connected MOFs constructed from nonanuclear and trinuclear lanthanide-carboxylate clusters: selective gas adsorption and luminescent pH sensing, Inorg. Chem., 2017, 56, 2159–2164 CrossRef CAS
;
(c) Z. W. Qi and Y. Chen, Charge-transfer-basedterbiumMOFnanoparticlesas fluorescentpH sensor forextremeacidity, Biosens. Bioelectron., 2017, 87, 236–241 CrossRef CAS
;
(d) S. Z. Huang, S. S. Liu, H. J. Zhang, Z. Han, G. Zhao, X. Y. Dong and S. Q. Zang, Dual-functional proton-conducting and pH-sensing polymer membrane benefiting from a Eu-MOF, ACS Appl. Mater. Interfaces, 2020, 12, 28720–28726 CrossRef CAS
.
-
(a) Y. Lu and B. Yan, A ratiometric fluorescent pH sensor based on nanoscale metal-organic frameworks (MOFs) modified by europium(III) complex, Chem. Commun., 2014, 50, 13323–13326 RSC
;
(b) X. Zhang, K. Jiang, H. J. He, D. Yue, D. Zhao, Y. J. Cui, Y. Yang and G. D. Qian, A stable lanthanide-functionalized nanoscale metal-organic framework as a fluorescent probe for pH, Sens. Actuators, B, 2018, 254, 1069–1077 CrossRef CAS
;
(c) T. Y. Liu and B. Yan, A stable broad-range fluorescent pH sensor based on Eu3+ post-synthetic modification of metal-organic framework, Ind. Eng. Chem. Res., 2020, 59, 1764–1771 CrossRef CAS
.
-
(a) J. Ma and B. Yan, Multi-component hybrid soft ionogels for photoluminescence tuning and sensing organic solvent vapors, J. Colloid Interface Sci., 2018, 513, 133–140 CrossRef CAS
;
(b) J. Zhao, X. L. Qu, J. M. Wang and B. Yan, Photophysical tuning of viologen based metal-organic framework hybrids via anion exchange and chemical sensing on persulfate (S2O82−), Ind. Eng. Chem. Res., 2019, 58, 18533–18539 CrossRef CAS
;
(c) J. Ma, L. M. Zhao, C. Y. Jin and B. Yan, Luminescence responsive composites of rare earth metal-organic frameworks covalently linking microsphere resin, Dyes Pigm., 2020, 173, 107883 CrossRef CAS
;
(d) J. Zhao, X. L. Qu, L. M. Zhao and B. Yan, New lanthanide coordination polymers of viologen carboxylic acid for luminescence spectroscopic response tuning, J. Photochem. Photobiol., A, 2020, 390, 112296 CrossRef CAS
.
-
(a) T. F. S. Mahapatra, A. Dey, H. Singh, S. S. Hossain, A. K. Mandal and A. Das, Two-dimensional lanthanide coordination polymer nanosheets for detection of FOX-7, Chem. Sci., 2020, 11, 1032–1042 RSC
;
(b) Y. Liu, Y. K. Lu, B. Zhang, L. Hou and Y. Y. Wang, Post-synthetic functionalization of Ni-MOF by Eu3+ ions: luminescent probe for aspartic acid and magnetic property, Inorg. Chem., 2020, 59, 7531–7538 CrossRef CAS
;
(c) C. N. Fan, X. X. Lv, M. Tian, Q. C. Yu, Y. Y. Mao, W. W. Qiu, H. Wan and G. D. Liu, A terbium(III)-functionalized zinc(II)-organic framework for fluorometric determination of phosphate, Microchim. Acta, 2020, 187, 84 CrossRef CAS
;
(d) H. Yang, C. J. Zhou, Y. F. Yang, Z. H. Chu, W. Yan, S. Q. Nie, J. Luo, S. M. Lin and Y. Wang, A new three sensing channels platform of Eu@Zn-MOF for quantitative detection of Cr(III), Inorg. Chem. Commun., 2020, 116, 107898 CrossRef CAS
.
-
(a) X. L. Qu and B. Yan, Ln(III)-functionalized metal-organic frameworks hybrid systems: luminescence properties and sensor for trans, trans-muconic acid as a biomarker of benzene, Inorg. Chem., 2018, 57, 7815–7824 CrossRef CAS
;
(b) X. L. Qu and B. Yan, Zn(II)/Cd(II)-based metal-organic frameworks: crystal structures, Ln(III)-functionalized luminescence and chemical sensing of dichloroaniline as pesticide biomarker, J. Mater. Chem. C, 2020, 8, 9427–9439 RSC
;
(c) Y. Zhang and B. Yan, A point-of-care diagnostics logic detector based on glucose oxidase immobilized lanthanide functionalized metal-organic frameworks, Nanoscale, 2019, 11, 22946–22953 RSC
;
(d) X. L. Qu and B. Yan, A Cd-based MOF containing uncoordinated carbonyl groups as lanthanide post-synthetic modification sites and chemical sensing of diphenyl phosphate as fame retardant biomarker, Inorg. Chem., 2020, 59, 15088–15100 CrossRef CAS
.
-
(a) N. N. Sun and B. Yan, Ag+-induced photoluminescence enhancement in lanthanide post-functionalized MOFs and Ag+ sensing, Phys. Chem. Chem. Phys., 2017, 19, 9174–9180 RSC
;
(b) A. Gamonal, C. Sun, A. L. Mariano, E. Fernandez-Bartolome, E. Guerrero-SanVicente, B. Vlaisavljevich, J. Castells-Gil, C. Marti-Gastaldo, R. Poloni, R. Wannemacher, J. Cabanillas-Gonzalez and J. S. Costa, Divergent adsorption-dependent luminescence of amino-functionalized lanthanide metal−organic frameworks for highly sensitive NO2 sensors, J. Phys. Chem. Lett., 2020, 11, 3362–3368 CrossRef CAS
.
-
(a) X. Y. Xu, X. Lian, J. N. Hao, C. Zhang and B. Yan, Double stimuli-responsive fluorescent center for food spoilage monitoring based on dye covalent modified EuMOFs: from sensory hydrogel to logic device, Adv. Mater., 2017, 29, 1702298 CrossRef
;
(b) T. Y. Liu, X. L. Qu and B. Yan, A highly sensitive and selective “turn-on” fluorescent probe of fleroxacin in human serum and urine based on Eu3+ functionalized metal-organic framework, Dalton Trans., 2019, 48, 17945–17952 RSC
;
(c) N. N. Sun and B. Yan, Fluorescence dection of urinary N-methylformamide for methylformamide for biomonitoring of hyman occupational exposure to N,N-dimethylformamide by Eu(III) functionalizedf MOFs, Sens. Actuators, B, 2018, 261, 153–160 CrossRef CAS
.
-
(a) Y. N. Zeng, H. Q. Zheng, J. F. Gu, G. J. Cao, W. E. Zhuang, J. D. Lin, R. Cao and Z. J. Lin, Dual-emissive metal−organic framework as a fluorescent “switch” for ratiometric sensing of hypochlorite and ascorbic acid, Inorg. Chem., 2019, 58, 13360–13369 CrossRef CAS
;
(b) H. Wang, X. L. Wang, M. S. Liang, G. Chen, R. M. Kong, L. A. Xia and F. L. Qu, Anal. Chem., 2020, 92, 3366–3372 CrossRef CAS
.
-
(a) X. Lian and B. Yan, Phosphonate MOFs composite as Off-On fluorescent sensor for detecting purine metabolite uric acid and diagnose hyperuricuria, Inorg. Chem., 2017, 56, 6802–6808 CrossRef CAS
;
(b) J. N. Hao, X. Y. Xu, X. Lian, C. Zhang and B. Yan, A luminescent 3d-4f-4d MOF nanoprobe as a diagnosis platform for human occupational exposure to vinyl chloride carcinogen, Inorg. Chem., 2017, 56, 11176–11183 CrossRef CAS
;
(c) G. F. Ji, T. X. Zheng, X. C. Gao and Z. L. Liu, A highly selective turn-on luminescent logic gates probe based on postsynthetic MOF for aspartic acid detection, Sens. Actuators, B, 2019, 284, 3–95 CrossRef
;
(d) J. Zhang, F. Liu, J. L. Gan, Y. J. Cui, B. Li, Y. Yang and G. D. Qian, Sci. China Mater, 2019, 62, 1445–1453 CrossRef CAS
.
-
(a) B. X. Liu, H. S. Shen, Y. Q. Hao, X. Zhu, S. Z. Li, Y. K. Huang, P. Qu and M. T. Xu, Lanthanide functionalized metal-organic coordination polymer: toward novel turn-on fluorescent sensing of amyloid beta-peptide, Anal. Chem., 2018, 90, 12449–12455 CrossRef CAS
;
(b) X. B. Zheng, R. Q. Fan, Y. Song, A. N. Wang, K. Xing, X. Du, P. Wang and Y. L. Yang, A highly sensitive turn-on ratiometric luminescent probe based on postsynthetic modification of Tb3+@Cu-MOF for H2S detection, J. Mater. Chem. C, 2017, 5, 9943–9951 RSC
;
(c) X. B. Zheng, R. Q. Fan, H. Y. Lu, B. W. Wang, J. K. Wu, P. Wang and Y. L. Yang, A dual-emitting Tb(iii)&Yb(iii)-functionalized coordination polymer: a “turn-on” sensor for N-methylformamide in urine and a “turn-off” sensor for methylglyoxal in serum, Dalton Trans., 2019, 48, 14408–14417 RSC
;
(d) N. N. Sun and B. Yan, A fluorescence probe based on Tb3+/Cu2+ co-functionalized MOFs to urinary sarcosine detection, Analyst, 2018, 143, 2349–2355 RSC
.
- X. Lian and B. Yan, Diagnosis of penicillin allergy: a MOFs-based composite hydrogel for detecting β-lactamase in serum, Chem. Commun., 2019, 55, 241–244 RSC
.
-
(a) L. Chen, D. H. Liu, J. Pen, Q. Z. Du and H. He, Ratiometric fluorescence sensing of metal-organic frameworks: tactics and perspectives, Coord. Chem. Rev., 2020, 404, 213113 CrossRef CAS
;
(b) S. Y. Wu, H. Min, W. Shi and P. Cheng, Multicenter metal-organic framework-based ratiometric fluorescent sensors, Adv. Mater., 2020, 32, 1805871 CrossRef CAS
;
(c) Y. F. Zhao and D. Li, Lanthanide-functionalized metal-organic frameworks as ratiometric luminescent sensors, J. Mater. Chem. C, 2020, 8, 12739–12754 RSC
;
(d) B. Yan, Lanthanide functionalized metal-organic frameworks” hybrid systems to create multiple luminescent centers for chemical sensing, Acc. Chem. Res., 2017, 50, 2789–2798 CrossRef CAS
;
(e) E. J. Gao, S. Y. Wu, J. Wang, M. C. Zhu, Y. Zhang and V. P. Fedin, Water-stable lanthanide coordination polymers with triple luminescent centers for tunable emission and efficient self-calibration sensing wastewater pollutants, Adv. Opt. Mater., 2020, 8, 1901659 CrossRef CAS
;
(f) S. J. Qin and B. Yan, An AND logic gate-based fluorescence probe for the detection of homovanillic acid, an indicator of the tumor, J. Lumin., 2019, 211, 431–436 CrossRef CAS
.
-
(a) D. M. Chen, C. X. Sun, Y. Peng, N. N. Zhang, H. H. Si, C. S. Liu and M. Du, Ratiometric fluorescence sensing and colorimetric decoding methanol by a bimetallic lanthanide-organic framework, Sens. Actuators, B, 2018, 265, 104–109 CrossRef CAS
;
(b) B. B. Li, W. J. Wang, Z. X. Hong, E. M. El-Sayed and D. Q. Yuan, Ratiometric fluorescence detection of trace water in an organic solvent based on bimetallic lanthanide metal-organic frameworks, Chem. Commun., 2019, 55, 6926–6929 RSC
;
(c) Y. Cheng, H. M. Zhang, B. Yang, J. Wu, Y. X. Wang, B. Ding, J. Z. Huo and Y. Li, Highly efficient fluorescence sensing of phosphate by dual-emissive lanthanide MOFs, Dalton Trans., 2018, 47, 12273–12283 RSC
;
(d) S. Y. Wu, M. C. Zhu, Y. Zhang, M. Kosinova, V. P. Fedin and E. J. Gao, A water-stable lanthanide coordination polymer as multicenter platform for ratiometric luminescent sensing antibiotics, Chem. – Eur. J., 2020, 26, 3137–3144 CrossRef CAS
;
(e) L. Yu, Q. T. Zheng, H. Wang, C. X. Liu, X. Q. Huang and Y. X. Xiao, Double-color lanthanide metal-organic framework based logic device and visual ratiometric fluorescence water microsensor for solid pharmaceuticals, Anal. Chem., 2020, 92, 1402–1408 CrossRef CAS
.
-
(a) W. Liu, C. Y. Chen, X. Huang, E. Q. Xie and W. S. Liu, Functional construction of dual-emitting 4-aminonaphthalimide encapsulated lanthanide MOFs composite for ratiometric temperature sensing, Chem. – Eur. J., 2019, 25, 10054–10058 CrossRef CAS
;
(b) A. M. Kaczmarek, Y. Y. Liu, C. H. Wang, B. Laforce, L. Vincze, P. Van Der Voort and R. Van Deun, Grafting of a Eu3+-tfac complex on to a Tb3+-metal organic framework for use as a ratiometric thermometer, Dalton Trans., 2017, 46, 12717–12723 RSC
;
(c) R. He, Y. L. Wang, H. F. Ma, S. G. Yin and Q. Y. Liu, Ratiometrioc Eu3+-functionalized metal-organic framework composite as ratiometric fluorescent sensor for highly selective detecting urinary 1-hydroxypyrene, Dyes Pigm., 2018, 151, 342–347 CrossRef CAS
;
(d) C. M. Li, J. P. Huang, H. L. Zhu, L. L. Liu, Y. M. Feng, G. Hu and X. B. Yu, Dual-emitting fluorescence of Eu/Zr-MOF for ratiometric sensing formaldehyde, Sens. Actuators, B, 2018, 253, 275–282 CrossRef
.
-
(a) X. Y. Xu, B. Yan and X. Lian, Wearable glove sensor for non-invasive organophosphorus pesticides detection based on double-signal fluorescence strategy, Nanoscale, 2018, 10, 13722 RSC
;
(b) S. J. Qin, X. L. Qu and B. Yan, A self-calibrating bimetallic lanthanide metal-organic luminescent sensor integrated with logic gate operation for detecting N-methylformamide, Inorg. Chem. Front., 2018, 5, 2971–2977 RSC
;
(c) Y. Zhang and B. Yan, A novel cucurbit [7] uril anchored bis-functionalized metal-organic framework hybrid and its potential use in fluorescent analysis of illegal stimulants in saliva, Sens. Actuators, B, 2020, 324, 128956 Search PubMed
;
(d) S. J. Qin and B. Yan, Dual-emissive ratiometric fluorescent probe based on Eu3+/C-dots@MOF hybrids for the biomarker diaminotoluene sensing, Sens. Actuators, B, 2018, 272, 510–517 CrossRef CAS
.
-
(a) K. Müller-Buschbaum, F. Beuerle and C. Feldmann, MOF based luminescence tuning and chemical/physical sensing, Microporous Mesoporous Mater., 2015, 216, 171–199 CrossRef
;
(b)
X. Z. Song, S. Y. Song and H. J. Zhang, Lanthanide Metal–Organic Frameworks, ed. P. Cheng, Springer publishers, 2015, pp. 109–144 Search PubMed
;
(c) J. H. S. K. Monteiro, Recent advances in luminescence imaging of biological systems using lanthanide(III) luminescent complexes, Molecules, 2020, 25, 2089 CrossRef CAS
;
(d) J. N. Xiao, L. J. Song, M. Y. Liu, X. L. Wang and Z. L. Liu, Intriguing pH-modulated luminescence chameleon system based on postsynthetic modified dual-emitting Eu3+@Mn-MOF and its application for histidine chemosensor, Inorg. Chem., 2020, 59, 6390–6397 CrossRef CAS
.
-
(a) Y. Zhou and B. Yan, Ratiometric detection of temperature using responsive dual-emissive MOF hybrids, J. Mater. Chem. C, 2015, 3, 9353–9358 RSC
;
(b) S. J. Qin, J. N. Hao, X. Y. Xu, X. Lian and B. Yan, Highly sensing probe for biological metabolite of benzene series pollutants based on recyclable Eu3+ functionalized metal-organic frameworks hybrids, Sens. Actuators, B, 2017, 253, 852–859 CrossRef CAS
;
(c) S. J. Qin and B. Yan, A facile indicator box based on Eu3+ functionalized MOF hybrid for the determination of 1-naphthol, a biomarker for carbaryl in urine, Sens. Actuators, B, 2018, 259, 125–132 CrossRef CAS
;
(d) Y. Zhang, X. Lian and B. Yan, A point-of-care diagnostics logic detector based on glucose oxidase immobilized lanthanide functionalized metal-organic frameworks, J. Mater. Chem. C, 2020, 8, 3023–3028 RSC
.
-
(a) S. P. Yang, W. Zhao, P. P. Hu, K. Y. Wu, Z. H. Jiang, L. P. Bai, M. M. Li and J. X. Chen, Lanthanum-based metal-organic frameworks for specific detection of Sudan Virus RNA conservative sequences down to single-base mismatch, Inorg.
Chem., 2017, 56, 14880–14887 CrossRef CAS
;
(b) B. Wang, Q. Yang, C. Guo, Y. X. Sun, L. H. Xie and J. R. Li, Stable Zr(IV)-based metal-organic Frameworks with predesigned functionalized ligands for highly selective detection of Fe(III) ions in water, ACS Appl. Mater. Interfaces, 2017, 9, 10286–10295 CrossRef CAS
;
(c) S. Bej, R. Das, N. C. Murmu and P. Banerjee, Selective identification and encapsulation of biohazardous m-xylene among a pool of its other constitutional C8 alkyl isomers by luminescent d10 MOFs: a combined theoretical and experimental study, Inorg. Chem., 2020, 59, 4366–4376 CrossRef CAS
;
(d) L. M. Fan, F. Wang, D. S. Zhao, Y. X. Peng, Y. X. Deng, Y. W. Luo and X. T. Zhang, A self-penetrating and chemically stable zinc(ii)-organic framework as multi-responsive chemo-sensor to detect pesticide and antibiotics in water, Appl. Organomet. Chem., 2020, e5960 CrossRef
;
(e) Y. L. Tang, H. F. Wu, J. M. Chen, J. L. Jia, J. P. Yu, W. Xua, Y. Y. Fu, Q. G. He, H. M. Cao and J. G. Cheng, Highly fluorescent metal organic framework probe for 2,4,6-trinitrophenol detection via post-synthetic modification of UIO-66-NH2, Dyes Pigm., 2019, 167, 10–15 CrossRef CAS
.
- X. Lian and B. Yan, Antineoplasticmitoxantrone monitor: a sandwimixed matrix membrane (MMMs) based on luminescent MOFs-hydrogel hybrid, Sens. Actuators, B, 2019, 281, 168–174 CrossRef CAS
.
-
(a) Z. Y. Ding, C. F. Wang, S. C. Wang, L. Wu and X. J. Zhang, Light-harvesting metal-organic framework nanoprobes for ratiometric fluorescence energy transfer-based determination of pH values and temperature, Microchim. Acta, 2019, 186, 476 CrossRef
;
(b) A. Afzalinia and M. Mirzaee, Ultrasensitive fluorescent miRNA biosensor based on a “sandwich” oligonucleotide hybridization and fluorescence resonance energy transfer process using an Ln(III)-MOF and Ag nanoparticles for early cancer diagnosis: application of central composite design, ACS Appl. Mater. Interfaces, 2020, 12, 16076–16087 CrossRef CAS
;
(c) F. Qu, Y. R. Ding, X. X. Lv, L. Xia, J. M. You and W. L. Han, Emissions of terbium metal-organic frameworks modulated by dispersive/agglomerated gold nanoparticles for the construction of prostate-specific antigen biosensor, Anal. Bioanal. Chem., 2019, 411, 3979–3988 CrossRef CAS
;
(d) C. Sun, S. Y. Zhao, F. Qu, W. L. Han and J. M. You, Determination of adenosine triphosphate based on the use of fluorescent terbium(III) organic frameworks and aptamer modified gold nanoparticles, Microchim. Acta, 2020, 187, 34 CrossRef CAS
.
-
(a) L. H. Cao, H. Y. Li, H. Xu, Y. L. Wei and S. Q. Zang, Diverse dissolution-recrystallization structural transformations and sequential Förster resonance energy transfer behavior of a luminescent porous Cd-MOF, Dalton Trans., 2017, 46, 11656–11663 RSC
;
(b) A. Khatun, D. K. Panda, N. Sayresmith, M. G. Walter and S. Saha, Thiazolothiazole-based luminescent metal-organic frameworks with ligand-to-ligand energy transfer and Hg2+-sensing capabilities, Inorg. Chem., 2019, 58, 12707–12715 CrossRef CAS
;
(c) H. Cai, W. G. Lu, C. Yang, M. Zhang, M. Li, C. M. Che and D. Li, Tandem Föster resonance energy transfer induced luminescent ratiometric thermometry in dye-encapsulated biological metal-organic frameworks, Adv. Opt. Mater., 2019, 7, 1801149 CrossRef
;
(d) J. N. Xiao, J. J. Liu, M. Y. Liu, G. F. Ji and Z. L. Liu, Fabrication of a luminescence-silent system based on a post-synthetic modification Cd-MOFs: a highly selective and sensitive turn-on luminescent probe for ascorbic acid detection, Inorg. Chem., 2019, 58, 6167–6174 CrossRef CAS
.
-
(a) J. Hao and B. Yan, Determination of urinary 1-hydroxypyrene for biomonitoring of human exposure to polycyclic aromatic hydrocarbons carcinogens by a lanthanide-functionalized metal-organic framework sensor, Adv. Funct. Mater., 2017, 27, 1603856 CrossRef
;
(b) X. Lian, Y. Zhang, J. M. Wang and B. Yan, Antineoplastic mitoxantrone monitor: a sandwith mixed matrix membrane (MMMs) based on luminescent MOFs-hydrogel hybrid, Inorg. Chem., 2020, 59, 10304–10310 CrossRef CAS
.
-
(a) S. Khan, P. Das and S. K. Mandal, Design and construction of a luminescent and highly stable 3D metal-organic framework with a [Zn4(μ3-OH)2]6+ core, Inorg. Chem., 2020, 59, 4588–4600 CrossRef CAS
;
(b) H. L. Zhu, J. P. Huang, Q. Y. Zhou, Z. W. Lv, C. Y. Li and G. Hu, Enhanced luminescence of NH2-UiO-66 for selectively sensing fluoride anion in water medium, J. Lumin., 2019, 208, 67–74 CrossRef CAS
;
(c) A. Sharma, D. W. Kim, J. H. Park, S. Rakshit, J. Seong, G. H. Jeong, O. H. Kwon and M. S. Lah, Mechanistic insight into the sensing of nitroaromatic compounds by metal-organic frameworks, Commun. Chem., 2019, 2, 39, DOI:10.1038/s42004-019-0135-2
;
(d) L. Liu, X. F. Chen, J. S. Qiu and C. Hao, New insights into the nitroaromatics detection mechanism of the luminescent metal-organic framework sensor, Dalton Trans., 2015, 44, 2897–2906 RSC
.
-
(a) A. A. Tehrani, H. Abbasi, L. Esrafili and A. Morsali, Urea-containing metal-organic frameworks for carbonyl compounds sensing, Sens. Actuators, B, 2018, 256, 706–710 CrossRef
;
(b) V. Pentyala, P. Davydovskaya, M. Ade, R. Pohle and G. Urban, Metal-organic frameworks for alcohol gas sensor, Sens. Actuators, B, 2016, 222, 904–909 CrossRef CAS
;
(c) A. Mallick, A. M. El-Zohry, O. Shekhah, J. Yin, J. T. Jia, H. Aggarwal, A. H. Emwas, O. F. Mohammed and M. Eddaoudi, Unprecedented ultralow detection limit of amines using a thiadiazole-functionalized Zr(IV)-based metal-organic framework, J. Am. Chem. Soc., 2019, 141, 7245–7249 CrossRef CAS
.
- J. N. Hao and B. Yan, Simultaneous determination of indoor ammonia pollution and its biological metabolite in human body by use of a recyclable nanocrystalline lanthanide functionalized MOF, Nanoscale, 2016, 8, 2881–2886 RSC
.
- X. Lian, T. F. Miao, X. Y. Xu, C. Zhang and B. Yan, Eu3+functionalized Sc-MOFs: turn-on fluorescent switch for ppb-level biomarker of plastic pollutant polystyrene, Biosens. Bioelectron., 2017, 97, 299–304 CrossRef CAS
.
- J. N. Hao and B. Yan, A dual-emitting 4d-4f metal-organic framework as a self-calibrating luminescent sensor for indoor formaldehyde pollution, Nanoscale, 2016, 8, 12047–12053 RSC
.
- X. Y. Xu and B. Yan, Fluorescent wearable platform for sweat Cl− analysis and logic smart-device fabrication based on color adjustable lanthanide MOFs, J. Mater. Chem. C, 2018, 7, 1863–1869 RSC
.
|
This journal is © the Partner Organisations 2021 |
Click here to see how this site uses Cookies. View our privacy policy here.