DOI:
10.1039/D0QI01060J
(Review Article)
Inorg. Chem. Front., 2021,
8, 234-272
Investigation on nanostructured Cu-based electrocatalysts for improvising water splitting: a review
Received
1st September 2020
, Accepted 27th October 2020
First published on 28th October 2020
Abstract
The effective use of earth-abundant electrocatalyst copper in the splitting of water as nanostructures with different combinations is central in replacing noble metals for the industrialization of hydrogen generation. Carbonaceous fuels, being front-line suppliers of energy, adversely affect the environment with greenhouse gas emission. Considering the electrocatalytic way of splitting water, it is one of the finest ways for producing pure hydrogen with a fast rate with no other undesired by-products; hence, researchers across the world have focused maximum attention to make them commercially applicable. To replace the noble metals, transition metal-based catalysts are promising. In this review, we have chosen to highlight solely the importance of Cu-based nanostructures as effective electrocatalysts for both oxygen evolution reaction (OER) and hydrogen evolution reaction (HER). Moreover, various synthetic approaches with Cu nanostructures such as mono-, bi-, and tri-metallic catalysts as oxides, hydroxides, sulfides, selenides, tellurides, and phosphides were studied for OER and HER in different pH conditions. Hence, this review gives a brief understanding of Cu-based nanostructures in electrocatalytic water splitting and based on this, it can be applied with other advancements in catalysts development for viable hydrogen generation with electrocatalytic water splitting.
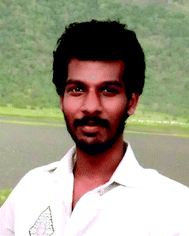 Kannimuthu Karthick | K. Karthick received his B.Sc. degree from Government Arts and Science College, Udumalapet, India, and M.Sc. degree from The American College, Madurai, India, in General Chemistry. He qualified UGC-JRF in December-2014 and joined Dr Subrata Kundu's research group in August 2015. He is currently working towards his Ph.D. thesis, which is mainly focused on electrocatalytic water splitting applications. |
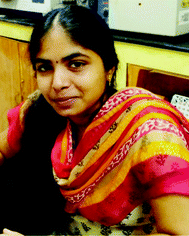 Kumaravel Sangeetha | K. Sangeetha received her B.Sc. degree in Chemistry (2011–2014) and M.Sc. degree in Organic Chemistry (2014–2016) from Periyar University, Salem. As she was the university topper in M.Sc, she received the gold medal and was awarded the INSPIRE scholarhsip (JRF) in March 2017 by DST, New Delhi. Currently, she is pursuing her Ph.D. under Dr Subrata Kundu since August 2017 in the field of size- and shape-selective synthesis of various transition metal nanostructures and their applications in energy, environment, and catalysis. |
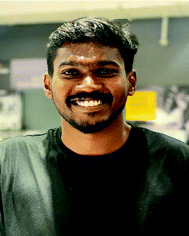 Selvasundarasekar Sam Sankar | S. Sam Sankar received his Masters degree in Chemistry (2013–2015) from Loyola College affiliated to Madras University, Chennai. He has cleared the Joint CSIR-UGC NET exam and has been awarded the UGC-JRF fellowship in December 2016. Currently, he is pursuing his Ph.D. under Dr Subrata Kundu in the field of synthesis and the fine-tuning of novel metal nanoparticles for their application in catalysis and electrocatalysis. |
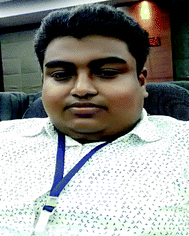 Arun Karmakar | Arun Karmakar received his B.Sc. degree from Derozio Memorial College affiliated to West Bengal State University (2017) and received his M.Sc. degree in Inorganic Chemistry from the same university (2019). While pursuing his M.Sc., he qualified the NET exam (National Eligibility Test) and obtained a JRF (Junior Research fellowship) from CSIR, New Delhi. Now, he is pursuing his Ph.D. under Dr Subrata Kundu at CSIR-CECRI since August 2019. His Ph.D. thesis work is mainly focused on the synthesis of nanostructured materials for catalysis, electrocatalysis, and SERS application. |
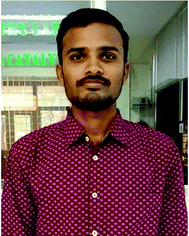 Ragunath Madhu | M. Ragunath received his B.Sc. degree (2014–2017) from ‘The Gandhigram Rural Institute’ Dindigul, India, and M.Sc. degree from Madras University, Chennai, India, in Analytical Chemistry (2017–2019). He obtained the DST-Inspire fellowship and joined under Dr Subrata Kundu's research Group in August 2020. His Ph.D. thesis work is mainly focused on catalysis, SERS, and electrocatalytic water splitting applications. |
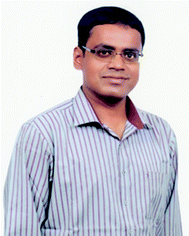 Subrata Kundu | Dr Subrata Kundu received his Ph.D. from the Indian Institute of Technology (IIT), Kharagpur, India, in early 2005. Then, he moved to the University of Nebraska, Lincoln, USA and later to Texas A&M University, College Station, Texas, USA, as a post-doc fellow (from 2005 to 2010). He is currently working as a Principal Scientist at CSIR-CECRI, Karaikudi, India. Dr Kundu is serving as an editorial board member of several international journals including prestigious ‘Scientific Reports’ from Nature publishers since 2015. Very recently, from a survey conducted by Stanford University, USA, Dr Kundu has been honoured as ‘Top 2% Scientist List’ (https://docs.google.com/spreadsheets/d/1mMonq947bS3KcMhsDpf_5z3H46My2t1k_7B3McozA68/htmlview) worlwide from India in Chemical Physics category having worldwide rank of 992. Dr Kundu and his co-workers are working in the forefront area of Materials Science with emphasis on energy, environment, catalysis, and electrocatalysis. |
1. Introduction
Hydrogen, the so-called ‘future fuel’, has been produced with different methods of approaches such as steam reforming, coal gasification, partial oxidation of methane, and by water electrolysis.1 The necessity of hydrogen is unparalleled for meeting the energy requirements, which is mainly demanded for ammonia production (Haber process), hydrogenation processes of oils and fats, production of hydrochloric acid, methanol production, etc.2 As of now, hydrogen is majorly produced by the steam reforming process and possesses its own drawbacks such as the utilization of high temperature and pressure conditions, and also the discharge of CO2 into the environment. Since fossil fuels are finite, findings other sources of energy in order to obtain hydrogen in a viable way and a large scale with no harm to the environment is highly required currently for a greener world.3,4 In the above-mentioned approaches, water electrolysis is one of the primary ways of producing highly pure hydrogen with no carbonaceous emission. When the produced hydrogen is utilized for the fuel cell, the by-product is just water, which makes this process as a highly advantageous one compared to other methods. Since renewable energy sources are not rational and intermittent, it retards their continuous utilization.3,5
Electrocatalytic splitting of water consists of oxygen evolution reaction (OER), which occurs at the anode and hydrogen evolution reaction (HER) that occurs at the cathode.6 As water is stable and poorly conductive, electrolytes that are conductive such as 0.5 M H2SO4 and 1 M KOH are used. The state-of-the-art catalysts so far are IrO2 or RuO2 as anodes and Pt as the cathode in the acidic environment.7–10 As they are scarce, developing stable earth-abundant catalysts for the extensive generation of hydrogen at a low cost is highly demanded considering the electrocatalytic splitting of water.7,11 Recent findings have enabled the successful utilization of iron group metals such as Fe, Co, and Ni, and they have been mostly explored as oxides,12,13,14–21,22–25 hydroxides,26–38 phosphides,39–46 and chalcogenides6,47–58 for OER and HER at different pH conditions. Recently, Ostrikov et al. reported several pioneering works with transition metal-based catalysts towards water splitting applications.59–62 However, OER in acidic electrolyte is still a bottleneck for the researchers.63 Hence, total water splitting in alkaline conditions with different kinds of 3d-transition metal-based catalysts have shown predominant results with extendable activity through pioneering surface modifications of the nanostructures.64
The activities of these transition metal-based catalysts are fine-tuned when they are studied as bi- and tri-metallic catalysts with other less explored metals of interest.64 Recent literature analysis has showed the appreciable use of earth-abundant Cu-based electrocatalysts for both OER and HER applications.65,66 Cu-Based electrocatalysts are prepared in different ways such as oxides, hydroxides, chalcogenides, phosphides, and also as layered double hydroxide (LDH)-based catalysts with other transition metal-based catalysts for both OER and HER in unique pH conditions.67–71 The results of Cu-based catalysts showed their potential in water splitting applications, which can be further enhanced by making them as effective nanostructures via different synthetic approaches. There is no review that has previously highlighted the nanostructures of Cu-based catalysts for both OER and HER. Hence, this review is devoted to the advancements in Cu-based nanostructures in terms of the synthesis and their application in both OER and HER applications. Also, in the future, with the aid of this review, one can easily employ Cu-based nanostructures as effective supports in electrocatalytic water splitting.
2. A short overview on the central parameters in electrocatalytic water splitting
2.1. Overpotential
The electrocatalytic splitting of water is a feasible method compared to others. In general, water, with a stable octet configuration, is not prone to release its components as oxygen and hydrogen, and hence, is thermodynamically non-spontaneous with the free energy change ΔG of +237 kJ mol−1.72 The standard reduction potential for the evolution of hydrogen is 0 V vs. RHE, whereas it is 1.23 V for the evolution of oxygen. Hence, the theoretical potential needed to split water is 1.23 V. | H2O → H2 + 1/2O2 E° = 1.23 V vs. RHE | (1) |
However, with the assisted resistances such as circuit, electrode, electrolyte, distance, and conductivity, and also the kinetic steps involved in both OER and HER drag the excess potentials, which are all generally called as overpotentials. Between OER and HER, the mechanistic steps involved in OER are too complex with four-proton coupled electron transfer reaction and each step drags additional overpotentials. It follows many pathways such as metal–oxide, hydroxide, or oxy-hydroxide and it is still not clear which is followed for oxygen evolution. The activity is dependent upon the electron density of the t2g and eg orbitals of 3d-transition metals. This is attributed to the effect of –OH ions’ intercalation and O2 cleavage at the surface, which affects the overall OER activity.72 The binding of –OH group should be optimum and after the formation of O2, it should be cleaved effectively, for which the electron density should be rich on the electrode. Considering these aspects, iron group metals (Ni, Co, and Fe) show favorable electronic configuration for OER. In case of Cu, even though repulsion is favored, the electron density is large, and hence retards –OH interaction effectively. Hence, engineering in the structures of transition metal-based catalysts is triggered with different combinations and the oxide/hydroxide phase formed during OER merely acts as the real catalyst surface.6 In case of HER, the mechanistic steps are based on the hydrogen adsorption and desorption phenomenon; thus, the mechanism is not complex compared to that of OER. Hydrogen adsorption is the initial step, which is called the Volmer step, followed by hydrogen desorption from the electrode surface either by electrochemical desorption, which is called the ‘Volmer–Heyrovsky’ step, or by the chemical desorption, which is called the ‘Volmer–Tafel’ step.6 It depends on the exposed active surface area, which mainly determines whether the working electrode undergoes chemical or electrochemical desorption. For example, with a high exposed surface area and optimum free energy for hydrogen adsorption and desorption, platinum stands on top of the ‘Volcano plot’ and follows the Tafel mechanism. In both OER and HER, the reaction mechanism is entirely different as shown below and varies depending on the electrolyte used. In acid, H+ ions form bonds for hydrogen evolution and form H2O for oxygen evolution. In alkali, OH− ions bond to the electrode surface for OER and H2O bonds on it for HER. Hence, in alkaline conditions, OER is too facile and HER is sluggish; when considering the acid, it is vice versa. The equations are as follows:
In acidic medium,
| 4e− + 4H+ → 2H2 (cathodic reaction) | (2) |
| 2H2O → 4H+ + 4e− + O2 (anodic reaction) | (3) |
In alkaline medium,
| 4e− + 4H2O → 4OH− + 2H2 (cathodic reaction) | (4) |
| 4OH− → 2H2O + 4e− + O2 (anodic reaction) | (5) |
Therefore, it is obvious here that bi-functional catalysts cannot meet viable hydrogen generation with earth-abundant materials, for which 3d-transition metal-based catalysts showed high rate activity for OER and HER in alkaline conditions.6,73,74 However, the corrosive nature of the acid retards the long term stability of these catalysts. Hence, the aim is to decrease the dominance of overpotentials to ensure high scale splitting of water with lesser applied energy inputs both anodically and cathodically along with the high robustness of the electrode.
2.2. Tafel slope
The inherent transfer of electrons at the electrode/electrolyte interface is judged by the Tafel slope values. From the derived Butler–Volmer equation at both higher anodic and cathodic overpotentials, it was found to follow the Tafel equation; the current density j is directly proportional to the exchange current density i0 and inversely proportional to the Tafel slope b. It is given as: | i = i0 log (η/b) | (6) |
where, i is the current density, i0 is the exchange current density, η is the overpotential, and b is the Tafel constant. Tafel slopes can be extracted from different methods and the frequently used one is from the polarization curves, where the logarithm of the current density is taken versus the overpotential and the observed values show the Tafel slope values in mV dec−1.6 But here, as it is potentiodynamic, the current observed cannot be the actual one and there is a chance of discrepancy, which will result in deviation in the Tafel slope values. Hence, potentiostatic analysis is precise to obtain a steady state current and based on this, the static current densities observed at different overpotentials can be taken for deriving the Tafel slope values, which will have more accuracy compared to the potentiodynamic method. From the observed Tafel slope values, the kinetics of different electrodes can be accessed for comparing their activities in addition to the overpotentials. This is valid as some catalysts will give higher activity at lower overpotentials whilst some will have higher activity at higher overpotentials with increased current densities.6 This can be well understood with the Tafel slope values when extracted with different overpotential regions. For OER, if it is four-electron transfer at the interface, the value is observed to be closer to 30 mV dec−1 and depending upon the poor kinetics, the observed values are changed with the increment in the values.73,74 Similarly, in the case of HER, the observed Tafel slope values indicate whether the electrode follows chemical or electrochemical desorption. If the value is nearly 30 mV dec−1, it is Tafel mechanism and follows chemical desorption, as observed in the case of Pt-based catalysts. Hence, from the Tafel slope values, one can easily find the charge transfer kinetics; it is always better to go for potentiostatic analysis to extract the log current densities for observing the appropriate Tafel slope values and relating them to the kinetics of the working electrodes in the chosen OER/HER study.73,74
2.3. Mass activity and turn over frequency
The activities of electrocatalysts certainly depend on the loading of a catalyst; hence, it is essential to relate the observed current densities with it for calculating the mass activity. The normalized loading of a catalyst is 0.205 mg cm−2 and when the materials are grown at 3D conducting substrates such as Ni foam and Cu foam, the loading becomes huge and unavoidable.73,74 Mass activity of a catalyst can be calculated bywhere A is the current density at a particular overpotential and g is the corresponding loading of a catalyst. Therefore, correlating the loading and current densities with mass activity becomes important. It also deals with normalized Cdl current densities, suggesting that only the exposed active surface area participates in the concerned OER and HER studies.75 The relevant studies on the increase in the activities with respect to the increased loadings to a certain limit and the subsequent decrease after certain loadings can give information about the participation of electrocatalytically active sites. The turn over frequency (TOF) is another important parameter to judge the potential of a catalyst in addition to the mass activity. It is defined as the molecules of hydrogen or oxygen produced at a single catalytically active site per unit time.75 TOF is a phenomenal activity descriptor and judges the efficiency of a catalyst at different overpotentials. It is calculated bywhere, J is the current density at the defined overpotential, S is the active surface area calculated from either the geometrical surface area or the electrochemically active surface area, n is the number of electrons transferred and F is the Faraday constant. Even with loading differences, TOF gives meaningful results and we cannot expect high TOF for high catalyst loading as all the loaded catalysts cannot be the active sites for OER/HER. TOF can be calculated from the geometrical area used if we use the surface area from the redox features by compromising only the oxidized or reduced species that have undergone the concerned OER/HER reactions. From these, the activities can be judged critically rather than showing the activity only by normalizing the geometrical area.72 Therefore, it is highly recommended to calculate the mass activity and TOF values of the catalyst while studying OER/HER.75
2.4. Stability
The stability of an electrocatalyst is indeed important as the robustness is required for long term utilization in electrocatalytic water splitting. It can be verified via both potentiostatic analyses such as chronoamperometry (potential is static and current is monitored as a function of time), chronopotentiometry (current is static and potential is monitored as a function of time), and potentiodynamic analysis such as cyclic voltammetry at high scan rates in the range from 1000 to 5000 cycles.72 A negligible difference in the activities before and after the stability studies ensures the stable nature of the catalysts. In potentiodynamic conditions, the change in the overpotential at defined current densities is a measure of the robustness of the catalyst. In general, stability in acidic conditions for OER is the bottleneck as both the corrosive nature of the acid and anodic overpotentials together affect the electrode surface strongly. When the materials are grown in Ni foam and Cu foam-like electrodeposition and hydrothermal approaches, they show great stability. This is because of the strong sticking of the electrode material to the pores of the conducting substrates. Recent findings have shown the stable nature of 3d-transition metals based catalysts in alkaline OER and HER.72 Still, the development of bi-functional catalysts is still underway for replacing scarce metals in both the activity and stability aspects. These parameters such as the overpotential, Tafel slope, mass activity, TOF, and stability have to be evaluated and considered critically for deriving better catalysts for electrocatalytic water splitting in multiple pH conditions.
3. Importance of Cu in electrocatalytic water splitting
Cu with the electronic configuration of 3d10 4s1 is a 3d-transition element and is highly earth-abundant in nature. Cu shows high electrical and thermal conductivity and shows strong reluctance to arc erosion when compared with iron group metals such as Ni, Fe, and Co. With promising activities in OER with the ease of hydroxyl intercalation and oxygen cleavage, it can offer impressive activity and stability. Cu has been extensively studied for water splitting because of its favorable electronic properties and ensures comparable OER and HER activity. Cu with oxidation states of 0, +1, and +2 were studied and analyzed with remarkable activities in OER and HER in different pH conditions. Moreover, Cu is studied in the form of oxides, hydroxides, layered double hydroxides, sulfides, selenides, phosphides, and tellurides as only Cu and with other transition metal-based catalysts for OER and HER applications. This review focuses on the sequential investigations of Cu-based catalysts for both OER and HER as follows:
• Cu-Based oxides and hydroxides for OER
• Cu-Based oxides for HER
• Cu-Based layered double hydroxides for OER and HER
• Cu-Based sulfides for OER and HER
• Cu-Based selenides for OER and HER
• Cu-Based tellurides for HER
• Cu-Based phosphides for OER and HER
The possibilities of Cu-based catalysts replacing noble metals will be discussed further and the relative advantages of Cu-based catalysts in electrocatalytic water splitting will be summarized at the end of this review.
4. Cu oxides and hydroxides for OER
Cu oxides with stoichiometries of CuO and Cu2O, even though with the less active nature for OER in terms of the onset overpotential and overpotentials at certain current densities compared to iron group metals such as Fe, Co, and Ni, can still further be extended for OER via surface modifications, growing them in conductive supports, and with porous structures. Moreover, the nature of the active species during OER that act as a real catalyst also matters and recent finding have enabled the identification of metastable CuIII–O as the catalyst surface during OER.76 The exploration of the CuO catalyst at different pH conditions was carried out by Liu et al., where they have electrodeposited CuO over the FTO plate with the area of 1 cm2 by varying the copper(II) precursor and have finally formulated CuO-1, CuO-2, and CuO-3 for OER.77 Here, CuO-1 had shown irregular arrangement of the nanoparticles (NPs) with the size of 200 nm to 1 μm, CuO-2 showed uniform microspheres and the diameter was about 1 μm, and CuO-3 exhibited flaky slices that showed a thickness of about 1.482 μm, which was confirmed via electrodeposition, and by changing the precursor of copper(II), the resulting morphology can be fine-tuned. After this, their OER activities were studied in different electrolytes such as 0.1 M potassium phosphate (pH 7), 0.1 M potassium borate (pH 9.2), 0.1 M KOH (pH 13), and 1 M KOH (pH 13.6). In the observed OER studies, CuO-3, which is annealed at 500 °C with flaky slices, showed high roughness factor and activity in OER. From the described polarization study of CuO-3, it can be seen that there is gradual increase in the current density when the pH is increased (Fig. 1). This is in accordance with the thermodynamic constraints of the ionic conductivities of the electrolytes used. Moreover, the onset potential is reduced when the pH is raised and becomes the highest for 1 M KOH, showing the highly active nature of CuO-3 for OER in alkaline electrolyte. Still, the neutral OER activity is also appealing with this catalyst. The stability of CuO-3 was further assessed via the chronopotentiometry study. At the current densities of 1 and 3 mA cm−2, the observed overpotential was not deviated much even for 24 h of reaction time and an assured stable nature; however, at 5 and 10 mA cm−2, the potential deviation is more after 12 and 8 h of continuous electrolysis, respectively. Extended X-ray absorption fine structure (EXAFS) was analyzed for CuO-3 with the standard for k space and R space is given in Fig. 2. The peaks observed between 1 and 2 Å were for Cu and the first co-ordinate shell O, while 2–4 Å denoted the distance between the nearest Cu co-ordinate shells for k space and fitted in the R space. From these observations and from the structure observed from XAS, the quadrangular structure showed CuOOCu, which could act as an active center for electrocatalytic OER.
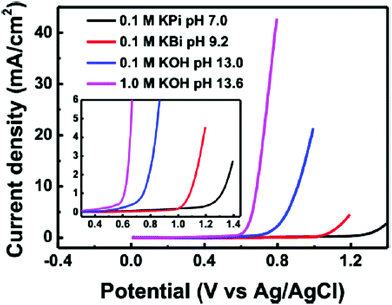 |
| Fig. 1 LSV curves of CuO-3 at different pH conditions for OER showing the variance in activity. Reprinted with permission from ref. 77. Copyright (2016) American Chemical Society. | |
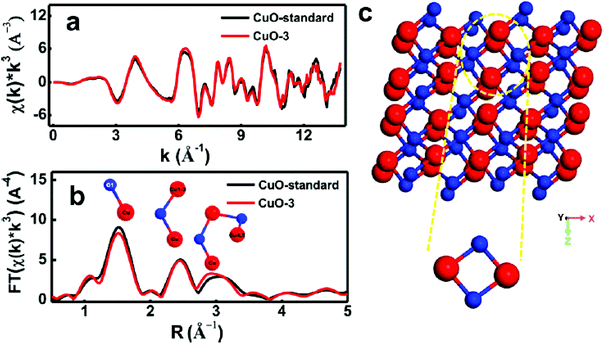 |
| Fig. 2 (a) EXAFS for CuO-3 and the standard in k space, (b) R space, and (c) structure of CuO-3. Reprinted with permission from ref. 77. Copyright (2016) American Chemical Society. | |
Other cost-effective conducting substrates can be utilized as supports such as graphene, carbon, 3D-foams, foils, and stainless steels for having superior activity in Cu-based oxides. In one of the interesting works, Pawar et al. developed 2D CuO over a stainless steel substrate via chemical bath deposition.78 As the stainless steel promises a cost-effective and conducting nature, developing these as strong supports for catalysts such as CuO will be rather advantageous to meet the viable needs of hydrogen production. In this work, nanobundles of CuO were grown over stainless steel and acted as a good catalytic material for OER (CuO was deposited and annealed at 200 °C) in alkali and 0.2 M borate buffer electrolytes. In 1 M KOH, the onset potential varied from 250 to 270 mV, which is superior; at 10 mA cm−2, the overpotentials observed were nearly 380 mV for the as-deposited CuO and 350 mV for annealed CuO. Further, the required overpotential is nearly 540 mV at 1 mA cm−2 in borate electrolyte. This shows the advantageous nature of conducting stainless steel as a valid support for copper-based oxides for assuring extended OER activity.
As only Cu is unstable under the prolonged conditions of electrolysis, passivation as oxides by high temperature annealing gives stability. Recently, Huan et al. developed Cu/CuO/Cu2O on Cu foil by electrodeposition, followed by annealing to have a dendritic growth of Cu for the ease of OER.79 This dendritic growth and porous nature of the copper oxide formed is attributed to the use of electrodeposition at high current densities, where hydrogen bubbles formed intensively and ensured the macropores of Cu/CuO/Cu2O, which is otherwise important for the diffusion of the active species without any mass transport limitations. Fig. 3a shows the micropores of Cu/CuO/Cu2O after electrodeposition, revealing the optimized structure for OER (1) and dendritic growth can be seen in Fig. 3b. The cross-sectional area with the presence of Cu, CuO, and Cu2O confirmed its rationality in delivering superior OER (Fig. 3c). To further enhance the activity, high temperature annealing (2) and also the further electrodeposition of N-containing imidazole was added to the surface (3). These three catalysts were evaluated for polarization studies in 1 M NaOH. The mictrostructural investigations revealed the nature of Cu present in the foil and from Fig. 4a–f, the observed HAADF mapping and SAED pattern from the area chosen in Fig. 4a showed the clear-cut presence of Cu, CuO, and Cu2O in the region chosen in the HAADF image. When catalyst (3) is subjected to OER, it shows tremendous activity by requiring just 290 mV as the overpotential at 10 mA cm−2 with a Tafel slope of 64 mV dec−1 in 1 M NaOH. Also, the stability study at 1 mA cm−2 showed negligible deviation in the activity even for 100 h of reaction. However, at high current densities of 10 mA cm−2, the deviation is considerable.79 These possible results are attributed to the method adapted in directing the sequential growth of copper oxides. This one could be further validated with other porous 3D foams to have highly active Cu-based nanostructures. Following this, in another work by Arshad et al., the electrodeposition of CuO was undertaken with different nanostructures such as nanosheets, nanocubes, nanoflowers, and nanoleaves.67 This has been done with the solution-phase approach, wherein varying the used concentration of NaOH and precursors resulted in the formation of different nanostructures (Fig. 5). These catalysts have been subjected for OER in 1 M KOH. From the observed polarization studies, the activity trend was in the order CuO NFs > CuO NLs > CuO NSs > CuO NCs. The onset potential was found to be the lowest in the case of CuO NFs with 1.48 V vs. RHE. The onset potentials for others were between 1.49 and 1.53 V. The overpotential at 10 mA cm−2 was the lowest for the NFs of CuO with just 270 mV as the overpotential (Fig. 6a). The difference in the overpotentials was considerable for all four types of CuO nanostructures, as can be seen from Fig. 6b. Electron transfer kinetics, as observed from the Tafel slope curves, indicated that CuO NFs showed lesser Tafel slope value of just 84 mV dec−1 (Fig. 6c). Further, the charge transfer resistance, as analyzed from the EIS spectra, showed that with very less Rct, CuO NFs showed improved kinetics of charge transfer at the electrode/electrolyte interface (Fig. 6d). Also, the electrochemically active surface area was found to be higher in the NFs. Here, the activity of CuO NFs is attributed to the highly dense growth of the nanosheets in the flower-like structures. Considering the stability aspect, during controlled potential electrolysis, CuO NFs exhibited higher stability at 20 mA cm−2, which could remain stable for 15 h. This comparative study enabled the further investigation of CuO-based nanostructures by the simple method of synthetic approach for OER studies. In another interesting work by Zhang et al., the inferior activity with bare metal oxides were nullified by choosing MOF frameworks that formed over the CuO dendrites in the 3D-Cu foam, which established outstanding OER activity in 1 M KOH (Fig. 7).80 This study showed the strategic output in engineering the surface of the Cu foam by deploying the intentional incorporation of MOF networks, which served in providing excess copper oxide NPs over the nanodendrites in the Cu/Cu2O@Cu foam. After the annealing process, the MOF network is converted into hollow porous CuO and C over the nanodendrites of the Cu/Cu2O@Cu foam without disturbing its structure. This is highly required in order to have fast diffusion of the electroactive species in directing the OER with higher activity and stability. Moreover, the presence of excess CuO NPs with the annealing of MOF can further provide material stability. For OER in 1 M KOH, the electrocatalyst Cu/Cu2O@Cu foam required a huge overpotential of 400 mV in achieving 10 mA cm−2 current density (Fig. 8a). Here, the low activity might be due to the nanoneedle structure of Cu2O, which is poor for catalyzing water oxidation. When the same was added with hollow porous CuO by means of MOF and after its subsequent annealing, the activity becomes huge and requires an overpotential of 286 mV at 10 mA cm−2 and 482 mV to attain 100 mA cm−2 (Fig. 8b). This is mainly attributed to the incorporation of conducting carbon, which ensured the fast and facile transport of the electroactive species for OER. The Tafel slope decreased from 212 mV dec−1 to nearly 66 mV dec−1 with the hollow porous CuO/C network (Fig. 8c). Further, the charge transfer resistance was in accordance with the earlier results. Also, the stability was monitored with chronopotentiometry study and the hollow porous CuO/Cu nanodendrites ensured no degradation in the activity even up to 50 h (Fig. 8d).80
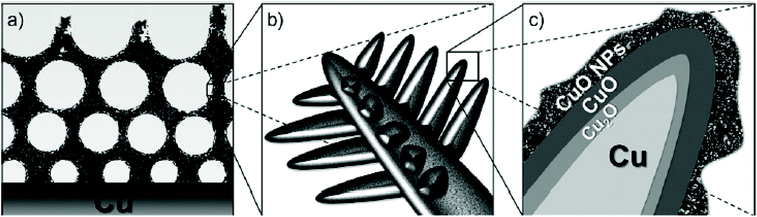 |
| Fig. 3 (a) Macropores of Cu, (b) dendritic growth, and (c) sectional view. Reprinted with permission from ref. 79. Copyright (2017) Wiley-VCH. | |
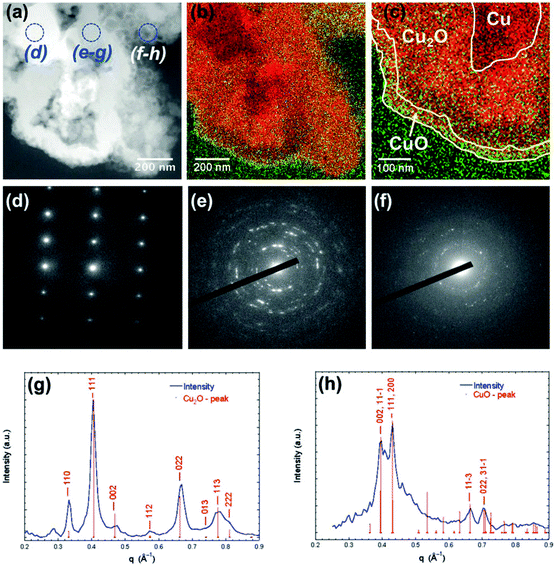 |
| Fig. 4 (a) HAADF area and blue circles indicate the area chosen for SAED, (b) and (c) STEM mapping showing Cu-orange and O-green, (d–f) SAED patterns, and (g and h) intensity profile analysis of the SAED observed. Reprinted with the permission from ref. 79. Copyright (2017) Wiley-VCH. | |
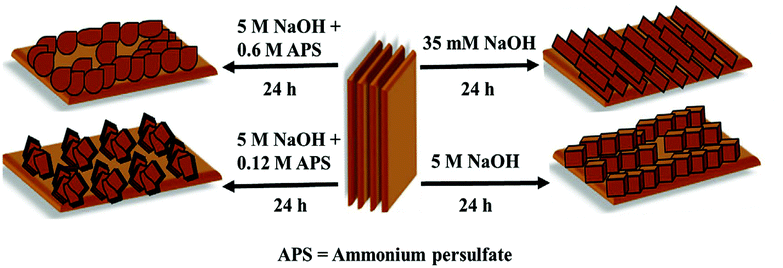 |
| Fig. 5 Schematization for the formulation of different nanostructures of CuO thin films. Reprinted with permission from ref. 67. Copyright (2020) Elsevier. | |
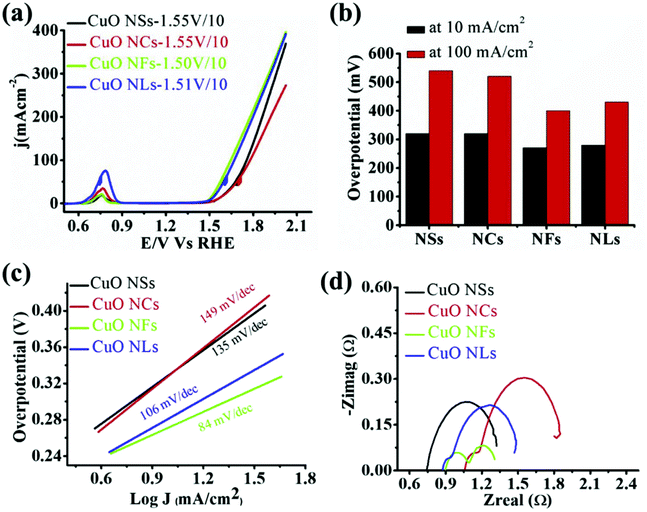 |
| Fig. 6 (a) LSV curves, (b) overpotential at different current densities, (c) Tafel slope, and (d) EIS spectra. Reprinted with permission from ref. 67. Copyright (2020) Elsevier. | |
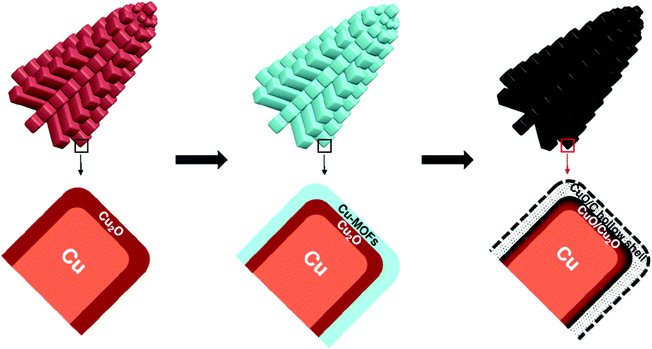 |
| Fig. 7 Schematization showing the hollow shell-coated copper oxide nanodendrites. Reprinted with permission from ref. 80. Copyright (2018) American Chemical Society. | |
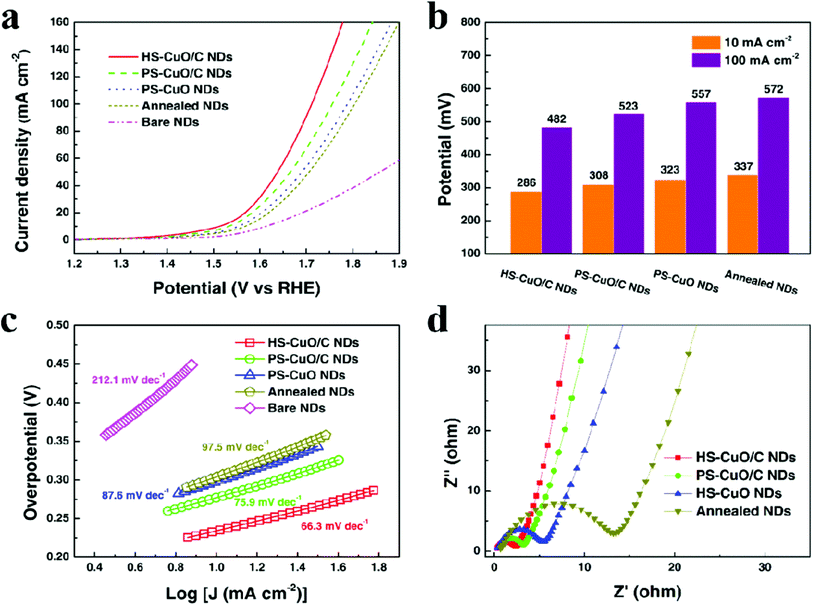 |
| Fig. 8 (a) LSV curves, (b) overpotential at different current densities, (c) Tafel slope, and (d) EIS spectra. Reprinted with permission from ref. 80. Copyright (2018) American Chemical Society. | |
The nature of active species in copper and copper oxides was evaluated further with Deng et al. by in situ Raman spectroscopy and X-ray absorption near-edge structure spectroscopy (XANES) for Cu, Cu2O, Cu(OH)2, and CuO in electrocatalytic OER.76 In general, previously reported Cu-based oxides with high activities have been postulated to be have highly accessible electrochemically active surface area, conducting supports such as C and highly porous structures. In this particular work, the study of Cu as its oxides and hydroxides by in situ Raman spectroscopy gave information that there is the formation of metastable CuIII ions during OER. This intermediate is observed only in the case of Cu(OH)2 and CuO but not in the case of Cu and Cu2O. Also, they observed that Cu(OH)2 and CuO showed 10 times better OER activity compared to Cu and Cu2O. This could be due to the ease of conversion of Cu2+ to Cu3+ compared to Cu+ to Cu3+ oxidative conversion. This is indeed really an important finding as based on this, other earth-abundant materials can be added to Cu(OH)2 and CuO for the viable production of hydrogen with ease. To evaluate the nature of the activity with Cu, Cu2O, Cu(OH)2, and CuO, in situ Raman spectroscopy was carried out during the chronoamperometry study. The potential taken is 1.7 V for chronoamperometry; CuO and Cu(OH)2 showed high current density of 1.7 and 0.8 mA cm−2, respectively. In the case of Cu2O and Cu catalysts, the current densities observed were in the range of 0.1–0.2 mA cm−2 only (Fig. 9a). This shows the poor activity of Cu+ catalysts. Further, the chosen potential of 1.7 V is a transpassive region, where these Cu2O and Cu will not get converted into the oxides or hydroxides during the chronoamperometry study. The Raman spectra before, during, and after chronoamperometry were analyzed for all the four catalysts (Fig. 9b). The observed peak at 603 cm−1 corresponds to CuIII–O, which is observed only in the case of CuO and Cu(OH)2. In case of Cu2O and Cu, no such peak was observed during OER, suggesting that there is no formation of CuIII–O. Also, from this one, it was concluded that the poor activity in these catalysts is corresponding to the unsuccessful conversion of CuIII–O. Also, the their morphological features were further assessed by post-OER SEM images and all of them showed retention of their morphological structures, which suggested the robustness of Cu-based oxides and hydroxides in OER (Fig. 9c).
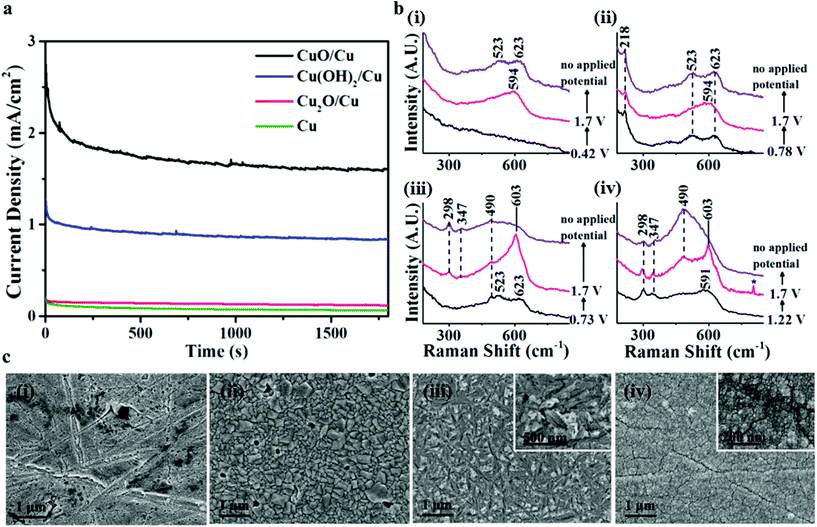 |
| Fig. 9 (a) Chronoamperometry, (b) Raman spectra before, during, and after OER, (c) SEM images after chronoamperometry. Reprinted with permission from ref. 76. Copyright (2018) American Chemical Society. | |
Hence, the development of optimized copper-based oxides is highly demanded as of now. In a work by Wang et al., the electrophoresis method is adapted to synthesize Cu(OH)2 and CuO electrocatalysts in FTO substrate and applied for OER in 0.2 M phosphate buffer (pH = 12).81 Prior to the electrophoresis method, the colloid was prepared by combining both CuCl2 and NaOH solution. After this, electrophoresis was conducted by keeping the FTO plate as the working electrode with electrolytes such as Cu(OH)2, iodine, and acetone. The thickness of the film is crucial for determining the resulting electrocatalytic activity. Hence, in this work, with varying deposition times, Cu(OH)2-FTO was prepared, as depicted in Fig. 10. This showed blue color and the same is annealed to prepare CuO-FTO and after annealing, it exhibited a dark orange color. From microstructural studies, Cu(OH)2-FTO showed a nanobelt-like structure and after annealing, CuO-FTO showed a fluffy nanowire structure. After this, electrochemical OER studies were carried out in phosphate buffer. Among different time optimized conditions, the one with 10 s of electrophoresis showed higher activity. The CuO-FTO electrode showed lesser onset potential of 370 mV and to reach 1 mA cm−2, required 500 mV as the overpotential with a Tafel slope value of 57 mV dec−1. From these observations, it is clear that by fine-tuning the nanostructural aspects, conducting support, enhancement in the active metal center, porosity, accessible surface area, and 3D structure, it becomes easy to engineer the activities with Cu-based oxides and hydroxides for OER. The method of synthesis, which is very simple with extended activity and stability, is always encouraged when we focus on earth-abundant materials such as Cu.
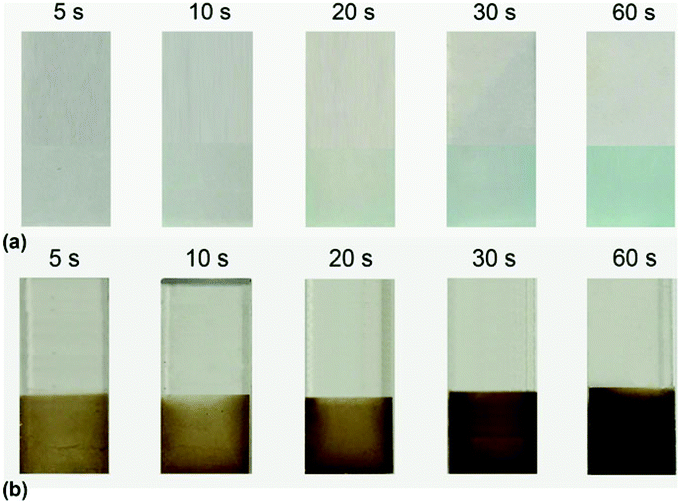 |
| Fig. 10 (a) Snapshots of Cu(OH)2 formed by the electrophoresis method at different time intervals and (b) the same samples annealed at 300 °C for CuO-FTO. Reprinted with permission from ref. 81. Copyright (2017) Materials Research Society. | |
As observed earlier, CuO is found to be highly active and in addition to surface engineering and variation in the method of formulation, the co-doping of other metals to the CuO surface can result in unprecedented increment in OER activities. Recently, Xiong et al., showed the increment in OER activity with CuO by means of Co doping for the alkaline OER.71 By the cation exchange method, some of the Cu in CuO was replaced with Co and the activity is enhanced with the observance of high current densities (Fig. 11). Here, only CuO/CF showed inferior activity and in Co-CuO/CF, the overpotential required at 50 and 10 mA cm−2 was nearly 299 and 330 mV, respectively, thus only empowering the effect of doping in copper oxides for high rate OER. The Tafel slope value was lesser and observed to be about 134 mV dec−1 closer to that of the commercial catalyst RuO2/CF. Moreover, the cycling study and chronopotentiometry study revealed that the doping of Co ensured high robustness of the CuO/CF catalyst for OER. In addition, the electrochemically active surface area and BET area was found to be huge in Co-doped CuO/CF.
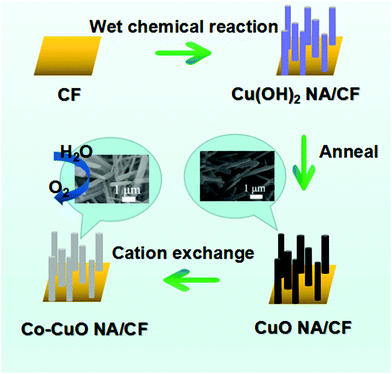 |
| Fig. 11 Schematic representation showing the cation exchange for the doping of Co over CuO/CF. Reprinted with permission from ref. 71. Copyright (2018) American Chemical Society. | |
These findings enabled the utilization of Co-doping over CuO for the enhancement in OER application in alkaline environment. Similarly, the electrodeposition of CuO with highly active Ni is done to prepare NiCuOx for extended OER activity in 1 M Na2CO3.82 In this work, Wang et al. had chosen ITO plate as a conducting support to grow materials such as NiOx, CuOx, and NiCuOx through electrodeposition method. In case of NiOx, surface coverage over ITO is more compared to NiCuOx, which could be due to the low electrodeposition rate here with the addition of Ni. The OER activity of only CuOx is very poor and required an onset overpotential of 420 mV (Fig. 12a). Similarly, NiOx required an onset overpotential of 470 mV and in case of NiCuOx, the onset overpotential required is lower with the value of 320 mV. The observed Tafel slope values were 190, 280, and 120 mV dec−1 for CuOx, NiOx, and NiCuOx, respectively, showing relatively high charge transfer kinetics in the presence of Ni with CuOx (Fig. 12b). The concentration variation of Ni percent with electrodeposition, which has been shown in Fig. 12c, gives evidence that 20% of Ni along with CuOx ensured low onset overpotential (Cu/Ni ratio, 4
:
1). Here also, with the addition of Ni to CuOx, the stability was assured from the chronopotentiometry study, where we can see that the potential deviation was lesser with the presence of Ni compared to only the CuOx catalyst (Fig. 12d). In another work, Chen et al., Ce-modified CuOx catalyst was prepared via the electrodeposition method.66 The addition of Ce to the CuOx surface led to 6.9% improved OER activity in 0.1 M KOH. A further increase in Ce concentration caused a reduction in the activity, which could be ascribed to the formation of the segregated phase of CeO2. Also, the presence of Ce4+ ions contributed to an increase in the OER activity. Up to a certain concentration of Ce, the activity was increased because of tetravalent Ce ions and as a further increase resulted in CeO2 phase segregation, the activity was reduced. For OER in 0.1 M KOH, the Ce-modified CuOx surface with 6.9% increased activity showed a current density of 2.4 mA cm−2 at an overpotential of 400 mV, which was 3.3 times higher than that of CuOx (Fig. 13). At concentrations more than 6.9%, the formation of the CeO2 phase was confirmed by Raman analysis, where a new peak corresponding to CeO2 was observed at 452 cm−1. Moreover, the presence of Ce4+ ions ensured more stability compared to only CuOx, as witnessed from stability study. From the observed results on oxides, the hydroxide catalysts of Cu for OER gave information that Cu can be a promising candidate in delivering high rate OER. Moreover, CuO and Cu(OH)2 catalysts could bring lesser overpotentials compared to the Cu and Cu2O catalysts with metastable Cu3+ ions for OER. Also, conducting supports such as carbon and the MOF framework that increase the surface active sites, 3D foam, and method of syntheses such as electrophoresis method, electrodeposition, cation exchange method, solvothermal approach, and high temperature annealing can result in impressive OER activities and stabilities. Also, the addition of other transition metals such as Ni, Co, and Ce to the CuO material could facilitate facile charge transport and assure high stability under prolonged OER studies.
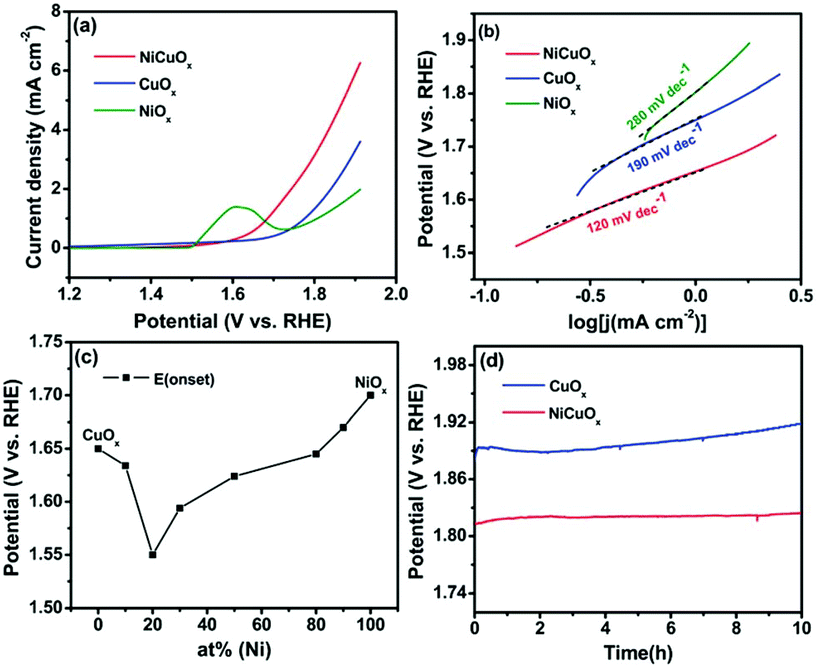 |
| Fig. 12 (a) LSV study, (b) Tafel slope, (c) concentration variation of Ni in % with CuOx, and (d) chronopotentiometry study. Reprinted with permission from ref. 82. Copyright (2017) Royal Society of Chemistry. | |
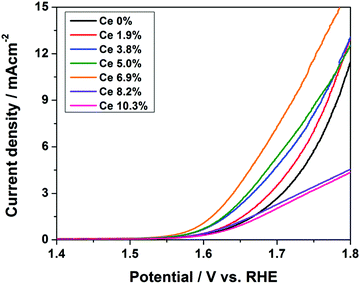 |
| Fig. 13 Polarization study of Ce with CuOx at different concentrations of electrodeposition. Reprinted with permission from ref. 66. Copyright (2017) Royal Society of Chemistry. | |
4.1 Cu-Based oxides for HER
Compared to the OER application of copper-based oxides and hydroxides, the HER studies are actually lesser, which is because of the poor ionic conductivity of the Cu surface in defining the HER kinetics and the subsequent energy descriptor in anchoring the electroactive species. Therefore, HER studies on Cu-based oxides have been carried out with conducting supports such as graphene, modifying methods of synthesis, and by adding other transition metal-based oxides so as to counteract the reluctance in achieving lower overpotentials for HER. In a notable work by Farinazzo et al., the surface of polycrystalline Cu towards HER in alkaline media was studied by carrying out modifications such as electropolishing (E-Cu (poly)), annealing (A-Cu (poly)), and polishing with alumina (P-Cu (poly)).83 The electrocatalytic HER activity in alkaline media was observed and the activity trend was in the following order: E-Cu (poly) < A-Cu (poly) < P-Cu (poly). This was studied by the XPS analysis and the Auger Cu LMM peak at 917 eV along with the peak at 919 eV suggest the Cu(I) and Cu0 oxidation states, respectively. From the observed relative intensities, the surface chemistry of these electrodes was determined for HER studies. The observed intensities are Cu(0) > Cu(I), Cu(0) ∼ Cu(I), and Cu(0) < Cu(I) for E-Cu (poly), A-Cu (poly), and P-Cu (poly) catalysts, respectively. From this, it is understood that the presence of excess Cu(I) in P-Cu (poly) ensured the higher HER activity and the overpotential required at 5 mA cm−2 is nearly 320 mV, whereas for A-Cu (poly) and E-Cu (poly), the observed overpotentials were 500 and 740 mV, respectively, at the same current density. To further validate the activity trends, the hydrophilic nature was studied with O 1s XPS high resolution spectra, where the H2O peak is major for P-Cu (poly) and the poorly active E-Cu (poly) does not show such a peak, thus confirming the increase in the hydrophilic nature of the surface when polishing with P-Cu (poly). From this study, it was suggested to increase the hydrophilic nature with Cu-based catalysts with a rough surface for the strong adsorption of electroactive species for HER (Fig. 14a–d).
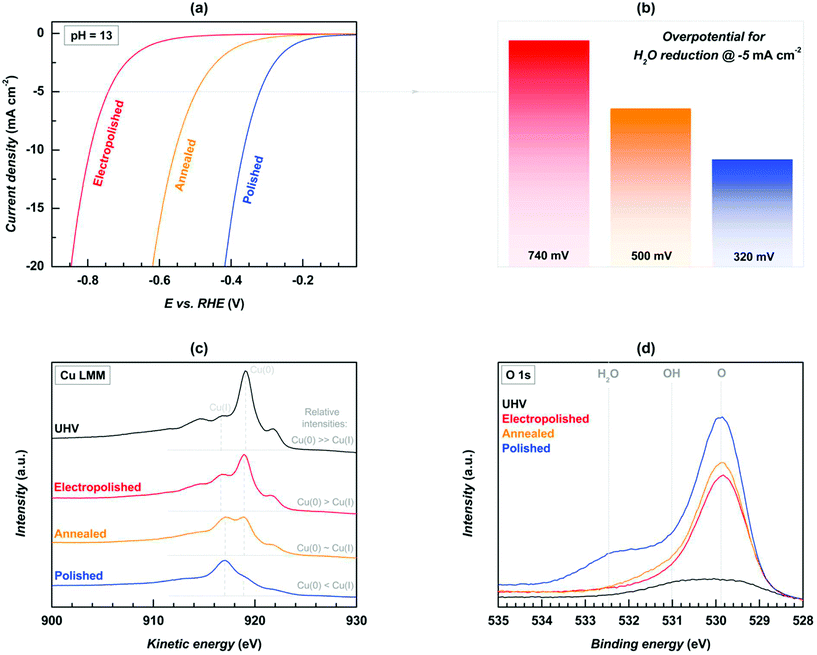 |
| Fig. 14 (a) LSV, (b) overpotential at the defined current density, (c) Cu LMM XPS, and (d) O 1s high resolution spectra. Reprinted with permission from ref. 83. Copyright (2019) Elsevier. | |
To enhance the HER activities of copper oxides, reduced graphene oxide, MOF porous network, and 3D Cu foam were utilized in an interesting work carried out by Ye et al.84 Initially, MOF networks were grown over Cu foam, which then formed Cu/Cu-MOF/GO with the subsequent addition of GO (Fig. 15). This was further annealed to provide Cu/Cu2O–CuO/rGO, which acted as a magnificent HER catalyst in alkaline conditions. The electrodes were studied for HER and as expected with highly conducting supports and a 3D porous structure, Cu/Cu2O–CuO/rGO could give astonishing HER activity by acquiring a very less onset overpotential of just 84 mV and an overpotential of 105 mV at a current density of 10 mA cm−2. From Fig. 16a, it is clear that compared to Cu foam, Cu/MOF/GO, and Cu/Cu2O–CuO catalysts, the Cu/Cu2O–CuO/rGO catalyst delivered superior HER activity by requiring lower overpotentials and by acquiring high current densities. The histogram in Fig. 16b infers the same and shows the potential with conducting supports such as rGO in the enhancing HER kinetics. The relatively less Rct compared to all other catalysts further guarantees the superiority of the Cu/Cu2O–CuO/rGO catalyst in HER (Fig. 16c). The chronoamperometry study for 14 h showed high stability ensuring the robustness of the catalyst (Fig. 16d). This was mainly attributed to the conducting 3D support, rGO network, and along with a high electrical conductivity ensured high rate charge transfer with high activity and stability. This work has paved a way for developing Cu-based oxides with MOFs and other conductive supports for enriching the HER activity and stability in an alkaline environment.
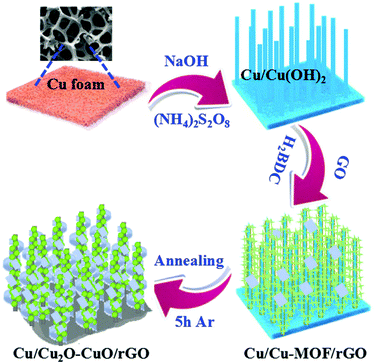 |
| Fig. 15 Schematization of the formation of Cu/Cu2O–CuO/rGO electrodes for HER. Reprinted with permission from ref. 84. Copyright (2018) Royal Society of Chemistry. | |
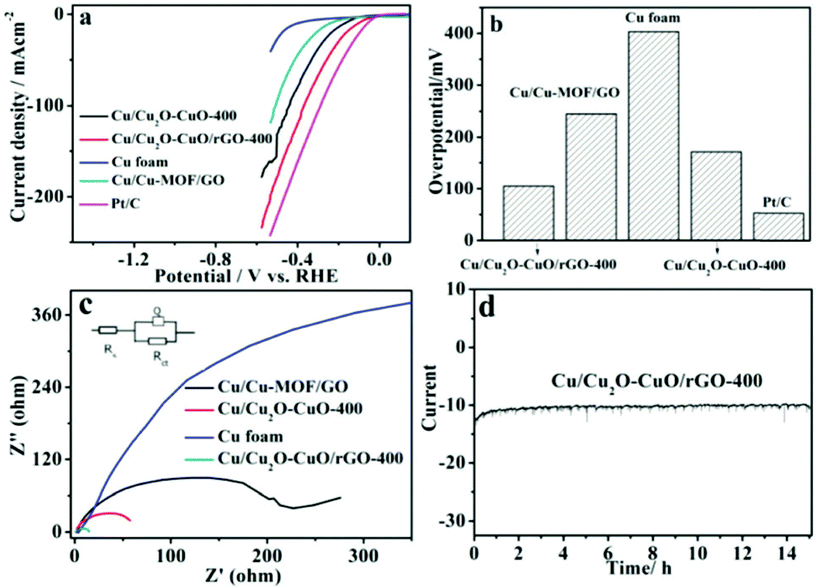 |
| Fig. 16 (a) LSV, (b) overpotential at the defined current density, (c) EIS, and (d) chronoamperometry for 14 h. Reprinted with permission from ref. 84. Copyright (2018) Royal Society of Chemistry. | |
Nano-composites can be used to increase the activities in HER in alkaline media. Following such a strategy, Tahira et al., formulated Co3O4–CuO nano-composites by the dip-coating method, which showed promising activities in HER.85 To reach 10 mA cm−2, Co3O4–CuO nano-composites required just 288 mV, whereas Co3O4 and CuO showed poor HER activity (Fig. 17a). This was attributed to the synergistic enhancement with Co and Cu, which ensured high activity. The charge transfer kinetics, as extracted from the Tafel slope values, showed a similar trend and the Co3O4–CuO nano-composites showed 65 mV dec−1 compared to that of Co3O4 and CuO, which needed 94 and 243 mV dec−1, respectively (Fig. 17b). Also, the stability was enhanced with the composites and in both the potentiodynamic and galvanostatic analyses, negligible deviation was achieved. These studies on Cu oxides for HER showed the possibilities for developing catalysts with distinct modifications to provide high HER activity in future. From these discussions, it is clear that the effect of Cu-based oxides can be made superior with the addition of conducting supports, anchoring in 3D foam, using nano-composites, etc., in HER.
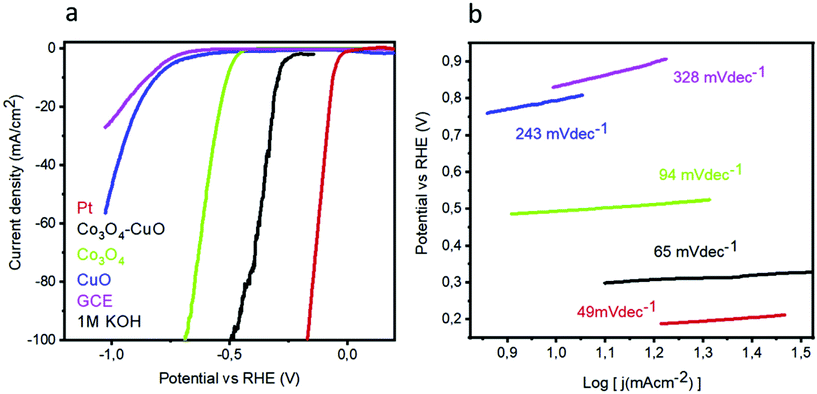 |
| Fig. 17 (a) LSV and (b) Tafel slopes for the Co3O4, CuO, and Co3O4–CuO nano-composites. Reprinted with permission from ref. 85. Copyright (2019) Elsevier. | |
5. Cu-Based layered double hydroxides for OER and HER
Layered double hydroxide catalysts are well known for their sequential layered arrangements of metal sharing octahedron (MII/MIII-(OH)6) that are stacked and present in between the anions and water molecules arranged via both hydrogen bonding and weak van der Waals force to make a sufficient path and space for the electrolyte ions to come and intercalate for OER and HER. This gives sufficient interlayer spacing and depending upon the anions, the spacing can be varied and the hydrophilic nature is enhanced with excess water molecules. The role of Cu in LDH systems has recently got attention with substantial activity and stability, considering both OER and HER and total water splitting (TWS). Yu et al. reported the easy formation of Cu@CoFe LDH for TWS with both electroreduction and electrodeposition approaches (Fig. 18).86 Initially, Cu foam was taken and to this, Cu(OH)2 was grown, which on further annealing gave the CuO@Cu foam. The subsequent electroreduction provided Cu Nanowires@Cu foam. This was again treated with Co and Fe metal precursors for undergoing electrodeposition again, which finally ended up in forming core–shell nanoarchitectures. These catalysts have been studied for OER, HER, and TWS in aqueous 1 M KOH. From the OER polarization studies, compared to the Cu foam, Cu NWs@Cu foam, and CoFe LDH@Cu foam, Cu@CoFe LDH showed greater activity. Cu@CoFe LDH electrodeposited at different times and among which Cu@CoFe LDH-60 s delivered better activity, required just 240 mV of overpotential to meet 10 mA cm−2 current density. The Tafel slope value is very lesser with just 44.4 mV dec−1, compared to all other catalysts, thus showing the high rate charge transfer kinetics of the catalyst. Moreover, the stability was further proved by the chronopotentiometric study for 24 h, where not much potential deviation was observed. Similarly, in HER, to attain the current density of 10 mA cm−2, the requirement of overpotential was nearly 171 mV with a lower Tafel slope value of 36.4 mV dec−1. The chronoamperometry study carried out for 30 h showed almost no degradation in activity, thus empowering the HER activity and stability for making it as a two-electrode system in a strong alkaline environment. When this Cu@CoFe LDH-60 was used as both the anode and cathode for a two-electrode set-up, the voltage required at 10 mA cm−2 was just 1.681 V, which is only 60 mV higher than that of the IrO2 anode and Pt cathode electrolyzer (Fig. 19a). Finding robustness with Cu-based catalysts for the two-electrode set-up is highly demanding and here, the chronopotentiometric study was carried out at 10 mA cm−2 for both the systems. The IrO2/Pt system after 12 h showed a gradual increase in the overpotential, whereas Cu@CoFe LDH-60 as anode and cathode delivered higher stability even up to 48 h and degradation was negligible. Here, the calculated faradaic efficiency was nearly 100%, confirming Cu-based LDHs as promising alternatives. Further, the calculated Cdl was found to be higher and with lesser Rct in EIS, showed admirable properties to be used as alternatives in the future (Fig. 19b–f). This study ensured the role of Cu-efficient TWS application in an alkaline environment. Similarly, in another interesting work, the same group highlighted the formation of Cu@NiFe LDH for TWS in 1 M KOH.87 Here, highly active NiFe as the metal precursor was taken for the LDH structure over Cu foam. Similar to the above discussions, electroreduction followed by electrodeposition resulted in the formation of the Cu@NiFeLDH/Cu foam (Fig. 20). For OER at 10 mA cm−2, it required an overpotential of 199 mV with a Tafel slope value of just 27 mV dec−1, suggesting nearly four-electron transfer for OER. In the same way, for HER at 10 mA cm−2, the overpotential required was just 116 mV with a Tafel slope of 58.9 mV dec−1. For both OER and HER, it showed high stability. After this, TWS was carried out and to attain 10 mA cm−2 current density with Cu@NiFe LDH as the anode and the cathode, just 1.54 V was required compared to IrO2/Pt, which required 1.63 V, which is actually 90 mV higher than that of the Cu-based NiFe LDH system. Post-structural studies such as HRTEM, mapping, and XPS have confirmed the stable nature of Cu@NiFe LDH electrodes (Fig. 21a–e). The mapping study confirmed the presence of all the expected elements such as Cu, Ni, and Cu. The activity for OER and HER from the polarization curves was observed again after the stability studies. From the observed LSV curves of OER and HER, it could be observed that the changes were still negligible (Fig. 21f). These reports of Cu-based LDH structures with other transition metals are promising for directing other simple ways to commercialize them in the future.
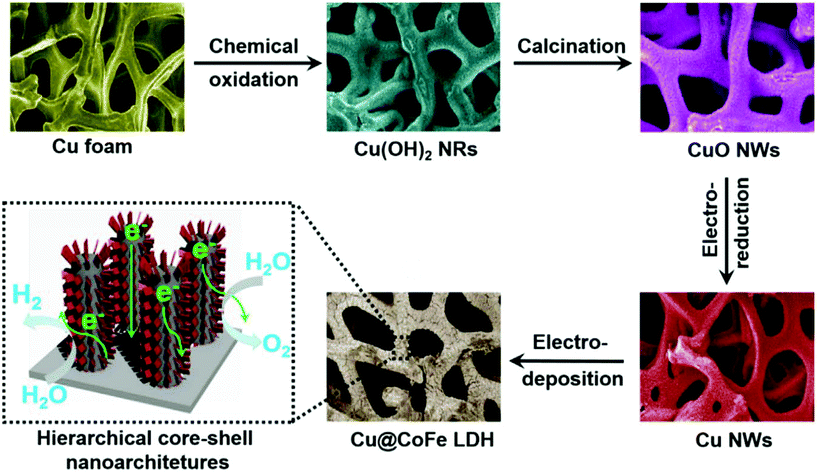 |
| Fig. 18 Schematization of the formation of core–shell Cu@CoFeLDH in Cu foam by the electrodeposition method. Reprinted with permission from ref. 86. Copyright (2017) Elsevier. | |
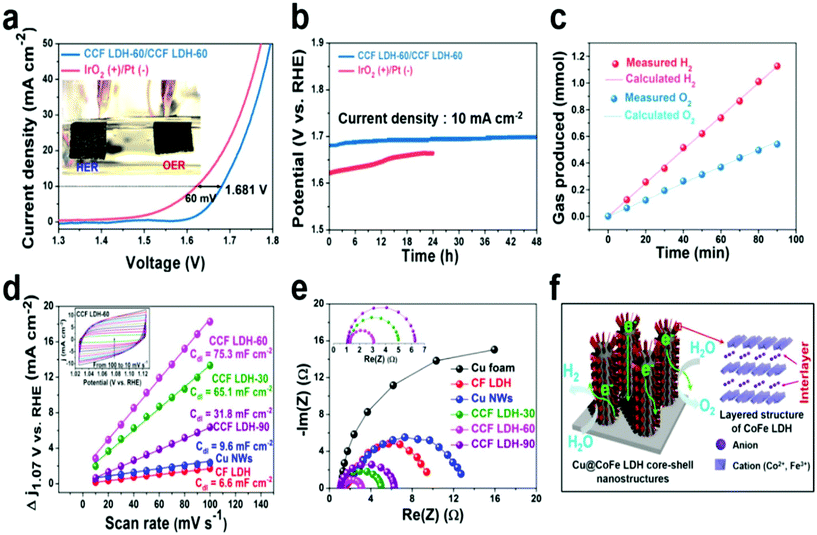 |
| Fig. 19 (a) LSV, (b) GSTAT analysis, (c) faradaic efficiency, (d) ECSA, (e) Nyquist plot, and (f) the structure of Cu@CoFeLDH over Cu foam. Reprinted with permission from ref. 86. Copyright (2017) Elsevier. | |
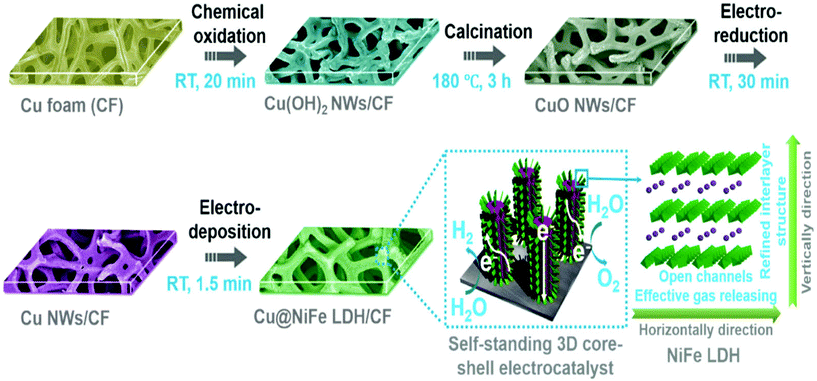 |
| Fig. 20 Schematization of the formation of core–shell Cu@NiFeLDH in Cu foam by the electrodeposition method. Reprinted with permission from ref. 87. Copyright (2017) Royal Society of Chemistry. | |
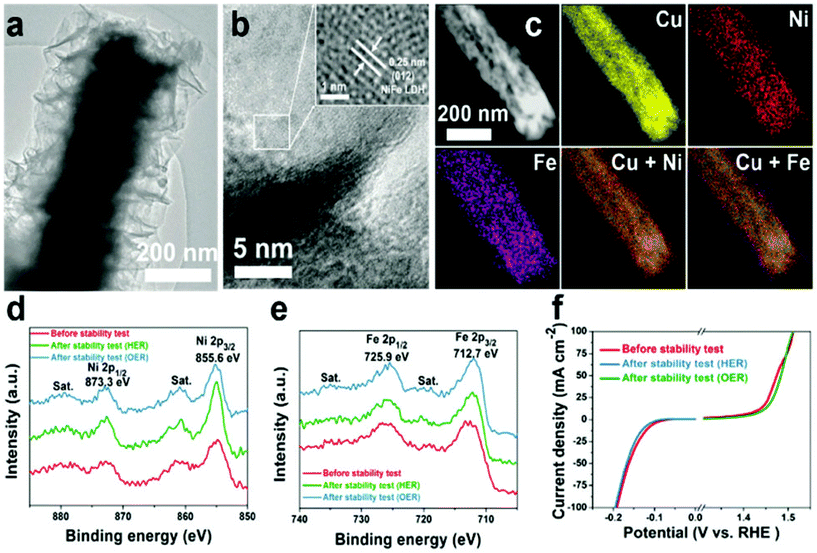 |
| Fig. 21 (a and b) HRTEM images (c) mapping results, (d) Ni 2p XPS, (e) Fe 2p XPS, and (f) the structure of Cu@CoFeLDH over Cu foam. Reprinted with permission from ref. 87. Copyright (2017) Royal Society of Chemistry. | |
Cu as Cu(OH)2 with NiFe LDH has also been found to have superior OER activity in KOH. In a work by Ma et al., unipolar pulse electrodeposition (UPED) was adapted to grow Cu(OH)2@NiFe LDH over carbon paper (Fig. 22).88 The feeding ratios of Ni/Fe were varied and the electricity used during the deposition process was also varied. Moreover, different methods for the deposition of Cu(OH)2 over NiFe LDH/CF for OER activities have been studied here (Fig. 22). Different methods such as UPED, cyclic voltammetry (CV), potentiostatic method (PM), and pulse potentiostatic method (PPM) were carried out for the deposition of Cu(OH)2@NiFe LDH over carbon paper. All these catalysts, prepared by the change in the stoichiometry and by the change in the applied quantity in electricity, have been subjected to OER activity. During deposition, among the quantities of electricity used, 2.5 C showed higher OER activity compared to the other quantities. In a similar way, with different methods adapted for deposition, such as UPED, CV, PM, and PPM, only the UPED method was found to be commendable compared to other methods for the deposition of Cu(OH)2@NiFe LDH. By the comparative studies carried out, in the UPED method, the Ni/Fe ratio of 6
:
4 was the best; the observed overpotential at a current density of 10 mA cm−2 was nearly 283 mV and the observed Tafel slope value was 88 mV dec−1, which is lesser than that of only Cu(OH)2 and NiFe LDH. The stability test carried out by continuous electrolysis for 10 h has summarized the advantageous incorporation of Cu(OH)2 with NiFe LDH. Here, only Cu(OH)2 was degraded during the activity after continuous electrolysis, whereas in Cu(OH)2@NiFe LDH, NiFe LDH protected Cu(OH)2 from leaching. These kinds of addition of Cu-based superstructures along with transition metal-based Ni, Fe, and Co-based LDHs is a strategy to improve the OER and HER activities. Cu itself has been added with highly active Ni as CuNi LDH and studied for OER application in detail. In a work by Xie et al., NiCu LDH was prepared first, and after this, selective vacancies in the brucite layer were made by the removal of Cu(II) with a simple ion-reductive complexation extraction (IRCE) method (Fig. 23).89 This vacancy created NiCux LDH, which was used as a catalyst for OER and delivered tremendous OER activity. The active nature of NiCu LDH for OER is poor, which is similar to that of Ni(OH)2. Less active LDHs such as NiCu LDH can be improved via the exfoliation process to introduce defects; however, the problem with this process is the loss of the mechanical stability of LDH structures, which deteriorates their activity for OER. Here, without affecting the actual structure, vacancies were created exclusively by complexation of an ionic extractant. This is advantageous because there is no disruption of the transition metal-based LDH structures. In reaching 10 mA cm−2 for OER, the overpotential required is nearly 343 mV for only NiCu LDH. For vacancy created SAV-NiCux LDH, the overpotential was reduced to only 290 mV at 10 mA cm−2. The observed Tafel slope for SAV-NiCux LDH is very less with just 45 mV dec−1 compared to that of NiCu LDH, which actually showed 83 mV dec−1. Moreover, in reaching a current density of 100 mA cm−2, the overpotential required for SAV-NiCux LDH was just 355 mV. Based on this, other LDHs can be prepared with Cu-based catalysts and through vacancy engineering without disrupting the LDHs surface, the activities for OER can be enhanced tremendously. Based on this, there are other ways, for instance, the method of synthesis has been modified to prepare highly active NiCu LDH nanostructures. In a work by Zheng et al., the solvothermal approach was followed to directly grow NiCu LDH over carbon cloth.90 This delivered substantial OER activity and required just 290 mV as overpotential for achieving 10 mA cm−2 with a Tafel slope of 42.5 mV dec−1. The stability study further confirmed the robustness of the NiCu LDH for OER. Compared to bimetallic LDH, trimetallic LDH has also been found to show higher OER activity. In a report from Hu et al., N-doped NiZnCu LDH with rGO was prepared over Ni foam and subjected to hybrid water electrolysis (Fig. 24).91 This work is mainly devoted to hybrid electrolysis such as ammonia electrolysis, urea electrolysis, and hydrazine electrolysis methods in order to reduce the energy demand required solely by OER in water electrolysis. For OER, N-NiZnCu LDH/rGO required an overpotential of 460 mV at 100 mA cm−2 and for attaining the same current density in HER, it actually required 196 mV as the overpotential. In a comparative study, to reach 100 mA cm−2, N-NiZnCu LDH/rGO||N-NiZnCu LDH/rGO two-electrode set-up required a voltage of 1.974 V. These observed activities showed the advantage of trimetallic LDH systems with Cu as one of the transition metals for enriching OER and HER. From the observed Cu-based LDH catalysts for OER and HER, it was found that the modification of the structures, method of synthesis, and direct growth over conducting substrates and by creating cationic vacancies on LDHs surfaces with different methods by not disrupting the LDHs surface, it was easy to enrich the activities of Cu-based LDH catalysts for OER, HER, and TWS applications. Moreover, the stability was more pronounced with Cu-based LDHs compared to Cu-based oxides and hydroxides. These observations were evidenced clearly from the long-term stability studies carried out at high current densities for Cu-based LDH structures in OER, HER, and In TWS studies. Still, with engineering at the Cu-based LDH surfaces, the activity can be further enhanced.
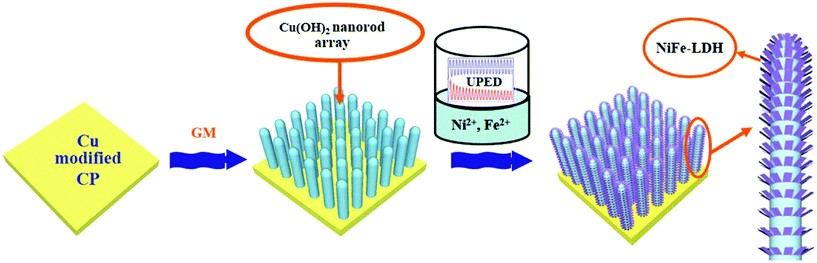 |
| Fig. 22 Schematization of the formation of Cu(OH)2@NiFeLDH in carbon paper by unipolar pulse electrodeposition method. Reprinted with permission from ref. 88. Copyright (2016) Elsevier. | |
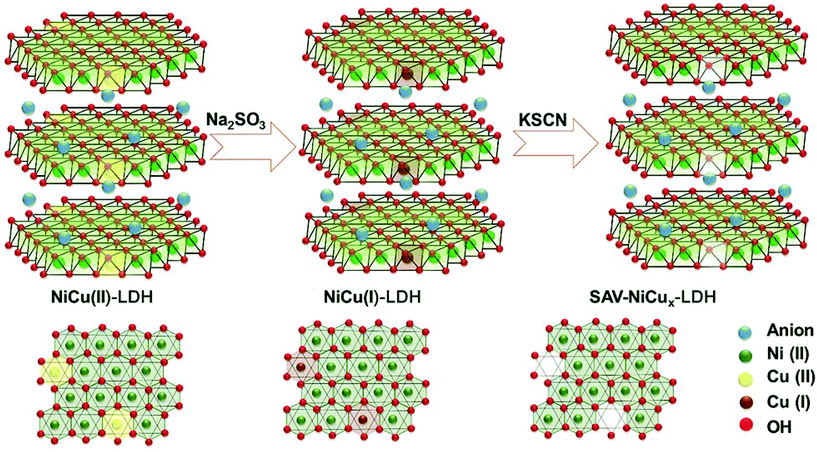 |
| Fig. 23 Creating Cu(II) vacancies with ionic reductive complexation extraction method in NiCu-LDH structures. Reprinted with permission from ref. 89. Copyright (2019) Royal Society of Chemistry. | |
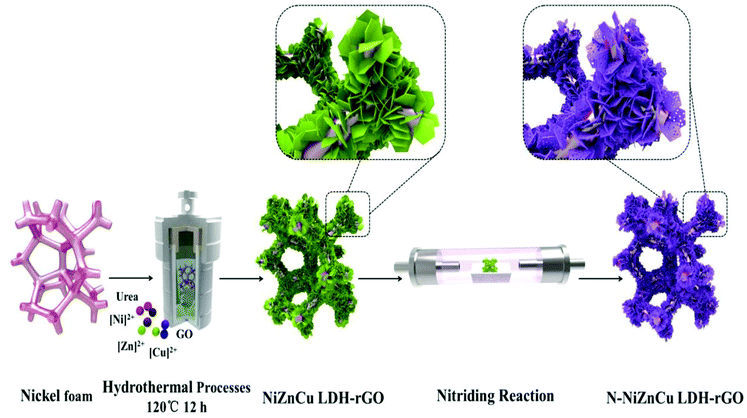 |
| Fig. 24 Schematic representation showing the formation of N-doped NiZnCu LDH-rGO via the hydrothermal process. Reprinted with permission from ref. 91. Copyright (2020) Elsevier. | |
6. Cu-Based sulfides for OER and HER
The sulfides of Cu have also been studied extensively for HER and OER applications at different pH conditions. In particular, the presence of sulfide ions with high electronegativity can offer higher probability for anchoring protons to its surface for effective HER with less applied overpotentials. Moreover, in general, chalcogenide-based Cu catalysts show superionic conductivity, which is highly demanded for electrocatalytic applications. Based on the different stoichiometries, these sulfide-based catalysts act as pre-catalysts and during OER, they become either oxide or hydroxide phase and are highly active compared to bare oxide and hydroxide. This has generated interest in studying chalcogenide-based materials in both OER and HER. With the highly-conducting nature of Cu, different stoichiometries of sulfur, and the tendency of acting as pre-catalysts during OER/HER, working with sulfides of Cu in HER and OER applications is in high demand. In a work by Kale et al., the nanorods of Cu2S were formed through the sacrificial template and applied for OER in 1 M KOH.92 Here, at first, the Cu substrates were treated with (NH4)2S2O8 to form Cu(OH)2, which was grown as nanorods as the (100) direction is more preferred and the chain is extended with square planar coordination with OH− ions. The sulfurization process was carried out by keeping this Cu(OH)2-Cu substrate in the Na2S solution, which successfully formed Cu2S over the Cu substrate. Initially, Cu2O was formed, which was converted to Cu2S as the solubility product of the latter is lesser compared to that of the oxide. The morphological feature, as seen from Fig. 25a–e, showed nanorods and the mapping confirmed the equal distribution of Cu and S over the surface. When Cu2S/Cu was subjected to OER, it just required 270 mV as overpotential to attain 10 mA cm−2. In the case of Cu(OH)2 and bare Cu, the required overpotentials were large, which were 390 mV and 510 mV, respectively. The charge transfer kinetics was fast in Cu2S/Cu with just 128 mV dec−1. The stability was further assured with the chronopotentiometry study and delivered a stable current density at 10 mA cm−2 (Fig. 26a–d). Also, the ion exchange method ensured the easy formation of Cu2S/Cu with appreciable activity. The less-active CuO-based structures can be finely modified with sulfides over CuO with different approaches. Wang et al. showed an anodization-cum-hydrothermal approach for the formation of Cu2S nanorods over Cu foam and applied the same for OER in 1 M KOH.93 Here, initially, the Cu foam was anodized in KOH and annealed further to form Cu2O nanowires. Thiourea was added under the hydrothermal treatment for the formation of Cu2S nanoarrays. This Cu2S-NA/Cu foam was modified with the thiourea concentration used and three different concentrations of thiourea were used (Fig. 27). Cu2S-NA/Cu foam-50 was found to be the one with high OER activity and required an overpotential of 360 mV to deliver 50 mA cm−2 current density. The Tafel slope was lower at 75 mV dec−1 compared to that of others. After this, He et al. highlighted the fastest way for the sulfurization of 3D Cu foam to Cu2S nanosheets arrays via plasma treatment and with the extreme anion exchange, within 5 min of reaction time, it was grown over the surface of Cu foam.94 The OER activity was monitored in 1 M KOH and to reach 20 mA cm−2, Cu2S/CF required just 336 mV as the overpotential. The activities of CuI/CF and Cu2S/CF were compared with ECSA and the observed values were 25.3 and 160.7 mF, respectively, showing a great difference in the active surface area obtained with the sulfurization process via plasma treatment. Further, the observed Rct difference shows values with a huge margin for CuI/CF compared to Cu2S/CF, thus empowering the advantage of the sulfurization process (Fig. 28a–d). The defined structural modifications of CuS (covellite) to Cu2S (chalcocite) have been studied for OER in phosphate buffer solution by An et al. with improved activity.95 Here, in this work, for the first time, CuS was converted into Cu2S, and by the addition of glycine, the activity was further enhanced for OER. Since CuS and Cu2S are semiconducting with Cu vacancies in the lattice, the reduction process to the phase transformation can bring an enhancement in the OER performance. The phase transformation was studied and the hexagonal phase of CuS was found to consist of layers of S-CuS3-Cu-CuS3 and van der Waals layers along the c-axis (Fig. 29a). The reduction was favored with the presence of OAm and when the S atoms in both CuS and Cu2S were observed in the (001) and (110) planes, they were hexagonally close packed (Fig. 29b). The major difference between them is that more Cu cations were inserted into the interstices of the S sites over Cu2S chalcocite structures. These Cu2S NPs were studied for OER in 6 mM glycine in 250 mM phosphate buffer as this particular concentration of glycine ensured a great increase in the activity compared to other concentrations and additives. The onset potential for OER is about 272 mV and also the overpotential at 10 mA cm−2 is comparatively lesser at 428 mV compared to CuS, which actually required an overpotential of 586 mV. Here, the phase transformation and use of glycine to further enhance the OER activity was finely studied to increase the activities of Cu-based sulfides in OER. These sulfide-based Cu catalysts were further studied in HER studies where they showed remarkable activities and stabilities. For HER at different pH conditions (pH = 0 and pH = 14), Bhat et al. prepared micro-hexagons of Cu2S via a hydrothermal approach. The observed overpotentials were 330 mV and 312 mV for reaching a current density of 10 mA cm−2 in alkaline and acidic conditions, respectively.96 Moreover, from the observed potentiostatic and potentiodynamic stability studies, the micro-hexagons of Cu2S delivered prominent stability, showing the robustness of Cu2S in extreme pH conditions for HER application. Further, the HER kinetics can be made superior by the addition of other transition metal-based sulfides along with copper-based sulfides. Wang et al. showed the use of Cu2S with MoS2 over Cu foam for HER.97 Initially, Cu(OH)2 was grown over Cu foam and after this, thiourea and sodium molybdate were added hydrothermally for the formation of Cu2S/MoS2/Cu foam (Fig. 30). Here, the use of thiourea ensured N-doping over MoS2. The Cu2S/MoS2/CF electrode was assessed for HER in KOH and the observed overpotential at 10 mA cm−2 was 91 mV. Also, a current density of 100 mA cm−2 was attained with just 129 mV as the overpotential, which is huge. The activity of Cu2S/MoS2/CF is comparatively higher compared with Cu2S/CF and MoS2/CF. Also, the observed Tafel slope was very less at just 41 mV dec−1 and high durability was achieved with Cu2S/MoS2/CF. Further, the OER and HER applications with these Cu-based sulfides have been observed by combining with other transition metal-based catalysts. In an interesting work by Zhou et al., CoS-shelled Cu2S nanorods were prepared by the room temperature sulfurization technique with Cu(OH)2 NRs/CF and Cu(OH)2 NRs@Co2CO3(OH)2/CF (Fig. 31).98 The prepared catalysts were subjected for OER and HER studies in 1 M KOH. The synergistic effect in the presence of both CoS and Cu2S ensured higher OER and HER activity in alkaline media. For OER, Cu2S NRs@CoS/CF just required an overpotential of 275 mV at 50 mA cm−2. Also, facile charge transfer kinetics is observed with a lower Tafel slope value of 54 mV dec−1. Similarly, in HER, Cu2S NRs@CoS/CF reached a current density of 50 mA cm−2 with a lesser overpotential of 324 mV and the charge transfer kinetics was facile with the Tafel slope of 121 mV dec−1. Here also, in alkaline electrolyte for both OER and HER, as a pivotal criterion, robustness was much pronounced with the composites of Cu2S and CoS with the conducting Cu foam as the 3D support. From the observed copper-based sulfides for OER and HER, the activity was enhanced by means of employing conducting 3D networks such as Cu foam, substantial growth of hydroxides to sulfides via different approaches, phase transformation, method of sulfurization, and by compositing with other transition metal-based sulfides of interest. However, the activity and stability of the sulfides of Cu are more pronounced when compared to the oxides of the same, as observed from the earlier discussions.
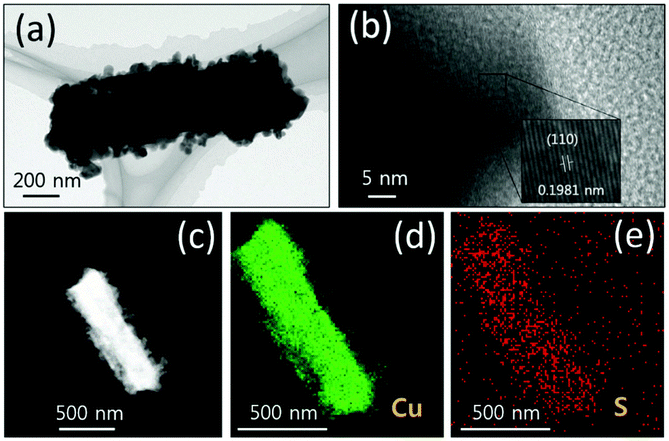 |
| Fig. 25 HRTEM imaging and HAADF mapping of Cu2S over the Cu substrate. Reprinted with permission from ref. 92. Copyright (2020) Royal Society of Chemistry. | |
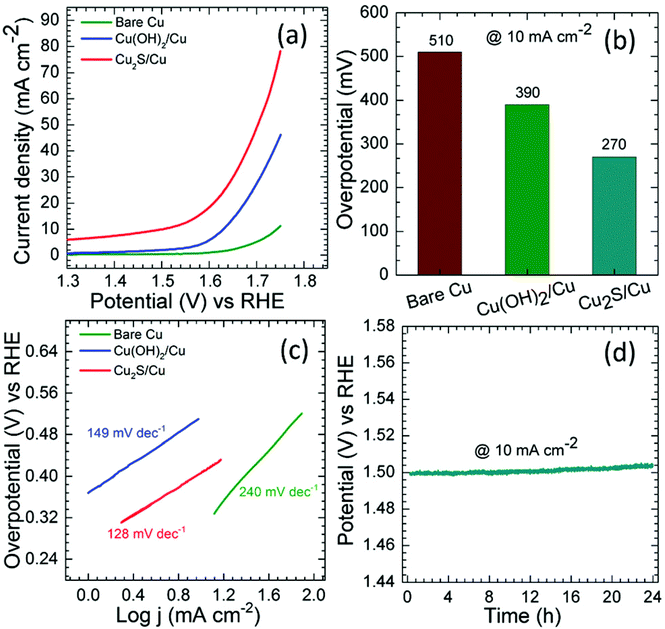 |
| Fig. 26 (a) LSV, (b) overpotential at 10 mA cm−2, (c) Tafel slope, and (d) chronopotentiometry. Reprinted with permission from ref. 92. Copyright (2020) Royal Society of Chemistry. | |
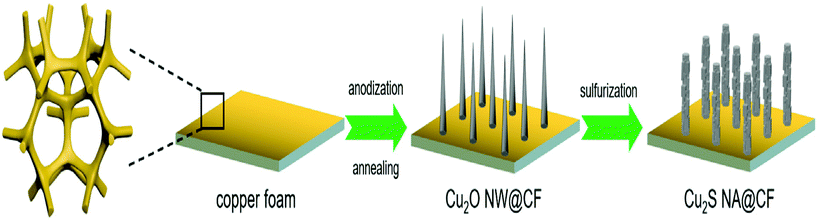 |
| Fig. 27 Schematic representation for the formation of Cu2S nanoarrays over Cu foam. Reprinted with permission from ref. 93. Copyright (2020) Elsevier. | |
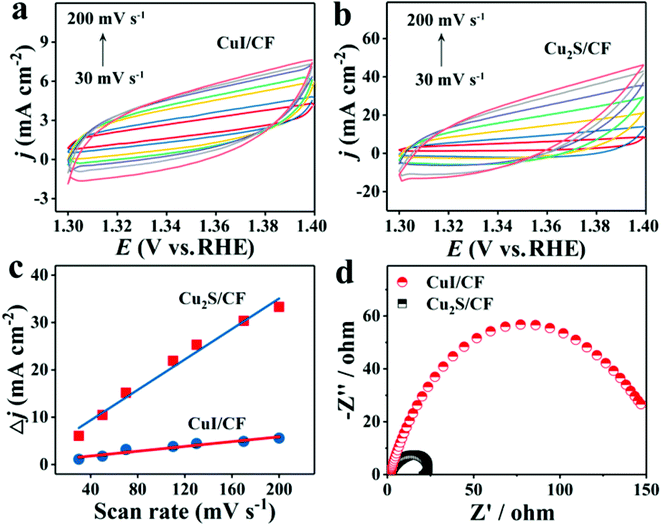 |
| Fig. 28 (a–c) ECSA determination with Cdl in the non-faradaic region and (d) the EIS. Reprinted with permission from ref. 94. Copyright (2018) American Chemical Society. | |
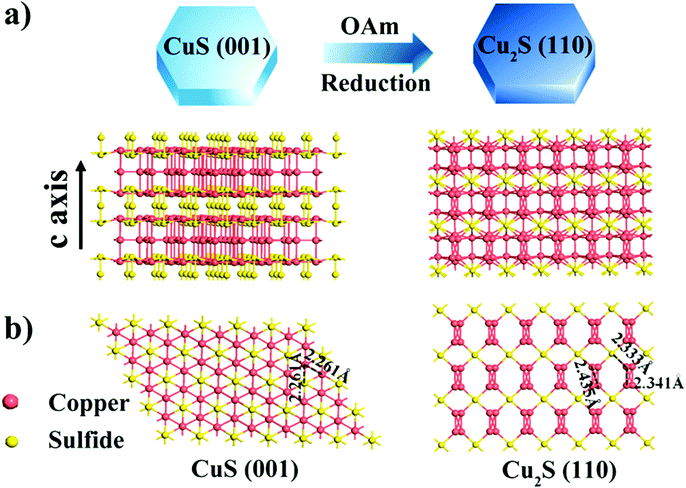 |
| Fig. 29 (a) Structural models of covellite CuS and chalcocite Cu2S NPs. (b) Side views of CuS (001) and Cu2S (110) planes. Reprinted with permission from ref. 95. Copyright (2015) American Chemical Society. | |
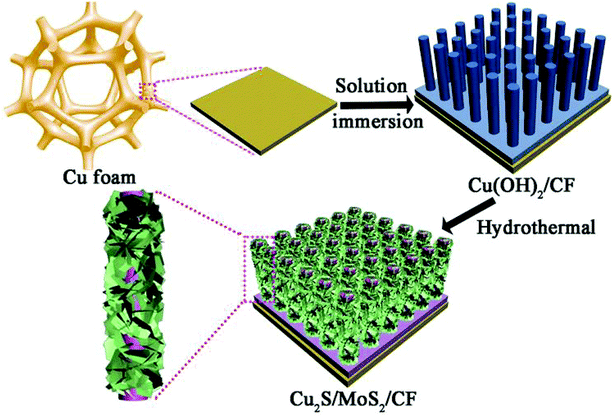 |
| Fig. 30 Scheme showing the formation of Cu2S/MoS2/CF via the hydrothermal approach. Reprinted with permission from ref. 97. Copyright (2018) Wiley-VCH. | |
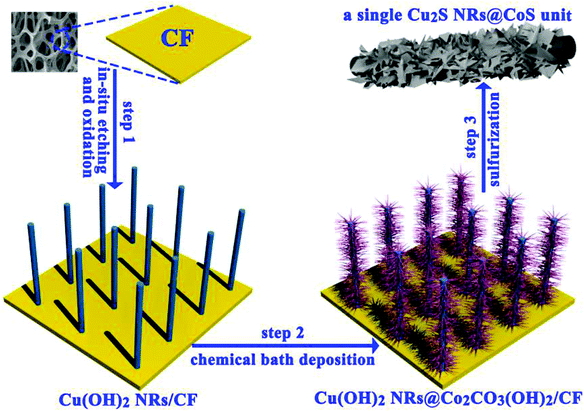 |
| Fig. 31 Schematic representation of CoS-shelled Cu2S nanorods over Cu foam. Reprinted with permission from ref. 98. Copyright (2018) Elsevier. | |
7. Cu-Based selenides for OER and HER
Selenides of Cu have also been found to show considerable enhancement in OER and HER with their high electrical conductivity. The study of copper-based selenides has shown impressive HER and OER activities. In a work reported by Wang et al., copper selenide-derived copper oxide has been used as a better catalyst for OER in 1 M KOH.99 Here, the Cu2Se/Cu foam was prepared by reacting Cu(OH)2/CF with NaBH4 and Se at room temperature. After this, the electrochemical oxidation resulted in the formation of oxides of Cu over Cu2Se/Cu foam. This catalyst delivered tremendous OER activity in 1 M KOH compared to Cu(OH)2 and CuO/CF. The CuO-A/CF delivered superior activity with a low overpotential of 297 mV to meet 10 mA cm−2, whereas CuO–C and Cu(OH)2 required 330 and 379 mV overpotentials at the same current density, respectively. The facile charge transfer kinetics was evidenced with Tafel analysis and CuO-A/CF showed a relatively lesser value of 72.8 mV dec−1. The stability was further assured with galvanostatic analysis for 50 h of reaction time with less degradation in activity. ECSA was comparatively higher with CuO-A/CF and Rct was too less compared to others (Fig. 32a–f). This study showed the influence of in situ generated surface oxide of Cu in enhancing copper-based selenides in OER. In another work, Shi et al. showed Cu2Se/CF via the solution immersion method and applied it for OER in 1 M KOH.100 Here, the pretreated Cu foam is taken for selenization and immersed in a mixture of NaBH4 and Se, and with the variation in copper precursors and selenide times, different catalysts were prepared and analyzed for OER (Fig. 33). In OER, for reaching 10 mA cm−2, Cu2Se/CF required just 200 mV as the overpotential to meet 10 mA cm−2, which is huge compared to other selenide-based catalysts. Also, the observed Tafel slope value was lesser with just 62.4 mV dec−1. This activity of Cu2Se/CF is higher compared to bare CF and Cu(OH)2/CF, showing the poor activity of metallic and hydroxides compared to the selenized one. Moreover, after subjecting Cu2Se/CF in OER, the electrode turns black, which could be ascribed to the surface oxide formed during the OER study. Hence, these copper-based selenide catalysts thus act as pre-catalysts and during OER, the oxide phase formed acts as a real catalyst. The comparative studies on copper selenides for OER were carried out by Masud et al., where chemical vapor deposition (CVD), electrodeposition (ED), and hydrothermal techniques were used.101 In all the methods, Cu2Se was successfully formed and analyzed for OER in 1 M KOH. From the observed studies, Cu2Se exhibited superior activity and durability. The observed OER activity was very facile in the electrodeposited one and the required overpotential of 270 mV at a current density of 10 mA cm−2. This study concluded that the method of synthesis does not influence the activity but the intrinsic catalytic activity of a material does. Also, the observed charge transfer kinetics is highly facile in the electrodeposited one with a value of 48.1 mV dec−1. Moreover, the durability was further verified by the chronoamperometry study and the change in activity, as observed from the polarization study after the stability, was negligible, hence, assuring Cu-based selenides for OER in an alkaline environment (Fig. 34a–d). The HER studies of copper selenides were also found to be superior and the conducting Cu support with electronegative Se can bring effective H+ ions incorporation to have high rate HER. Hence, copper-based selenides are mostly demanded for HER application. Similar to the above discussions, the successful initial conversion of Cu to Cu(OH)2 and subsequent selenization with the precursors NaBH4 and Se could cause selenization over the Cu foam. In a work reported by Zhang et al., Cu2−xSe/CF was prepared by in situ anion exchange method where NaBH4 and Se tend to give Se ions, which exchanged OH− ions from Cu(OH)2/CF.102 Cu2−xSe/CF was then subjected to acid for HER applications. To reach a current density of 100 mA cm−2, it just required 313 mV as the overpotential. The observed Tafel slope from the CV study was 90.77 mV dec−1 and from log
Rct−1, the observed Tafel slope was 84.4 mV dec−1 for Cu2−xSe/CF. The durability at 50 mA cm−2 for 24 h was assessed and observed to be highly stable even in an acidic environment at such a high cathodic current density. This observation made clear that Cu-based selenides are promising for HER under acidic conditions with pronounced activity and stability. The high overpotential requirement with Cu foam is reduced much and even up to 1 V reduction in overpotential was observed after selenization by a hydrothermal approach, as reported by Anantharaj et al.103 The Cu foam was taken and treated with NaBH4 and Se in both the hydrothermal and wet-chemical approach (Fig. 35). The prepared catalysts were screened for HER in 0.5 M H2SO4. Here, just by the selenization of Cu, the overpotential was reduced remarkably. Here, the observed charge transfer resistance was nearly 0.3–0.6 Ω; moreover, the Tafel slope values are nearly 32–35 mV dec−1, empowering similar kinetics to that of Pt/C-based catalysts in HER. Also, the stability was further confirmed.
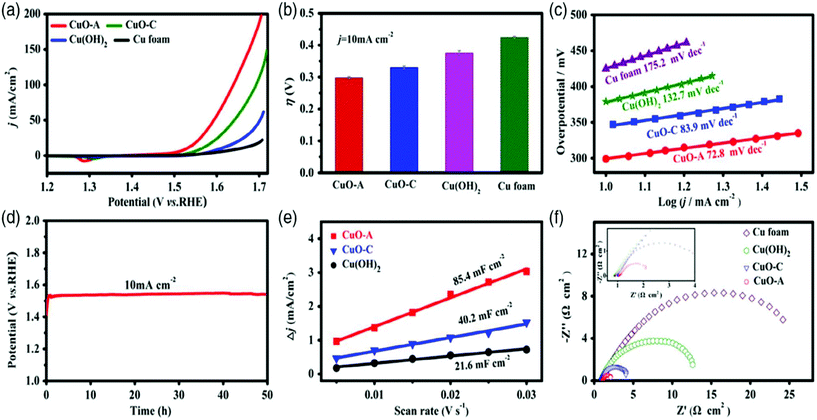 |
| Fig. 32 (a) LSV, (b) overpotential at 10 mA cm−2, (c) Tafel slope, (d) chronopotentiometry, (e) ECSA, and (f) EIS results of CuO-A, CuO–C, and Cu(OH)2. Reprinted with permission from ref. 99. Copyright (2020) Wiley-VCH. | |
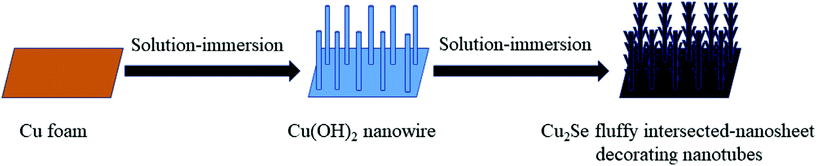 |
| Fig. 33 Schematic representation for the formation of Cu2Se/CF by the solution immersion method. Reprinted with permission from ref. 100. Copyright (2019) Elsevier. | |
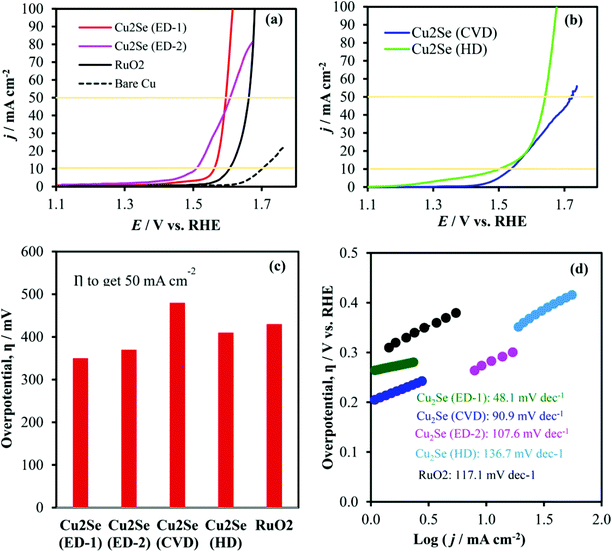 |
| Fig. 34 (a) and (b) LSV, (c) overpotential at 50 mA cm−2, and (d) the Tafel slope results of Cu2Se catalysts. Reprinted with permission from ref. 101. Copyright (2018) American Chemical Society. | |
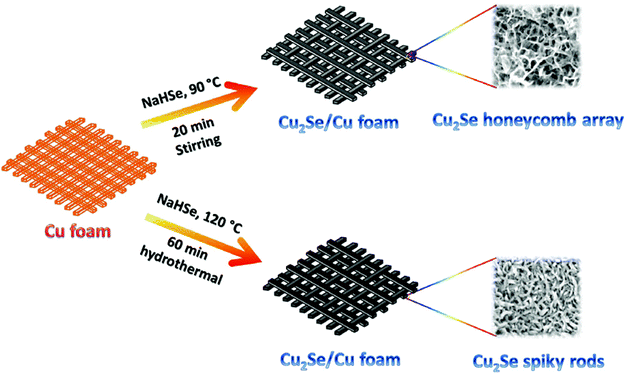 |
| Fig. 35 Selenization of Cu in both wet-chemical and hydrothermal methods. | |
The electrodeposition method for forming bimetallic selenides along with Cu is crucial to relate the HER activity in alkaline medium. In an interesting report, Gao et al. highlighted the potentiostatic electrodeposition of Ni–Se–Cu over Ni foam, which is applied as a catalyst for HER in alkaline electrolyte.104 Previous reports have highlighted the conducting support to be Cu foam and here in this work, Ni foam was studied. Interestingly, Ni–Se–Cu showed superior HER activity and to reach 10 mA cm−2, it just needed 136 mV as the overpotential. Further, stability was achieved in both galvanostatic and potentiodynamic conditions. The presence of Cu here ensured high electrical conductivity. When compared with only Ni–Se over Ni foam, Ni–Se–Cu/NF has been found to be superior. The electrocatalytic OER and HER activities of Cu-based selenides were seen individually and inferred to have highly engaging activity and stability. Hence, it is highly desirable to choose copper-based selenides in TWS application. In a work by Chakraborty et al., CuSe was electrophoretically deposited over NF and studied for both OER and HER in KOH electrolyte.105 Initially, CuSe was formed by high temperature annealing and after this, it was coated over NF by the electrophoretic deposition method. For the OER study, CuSe/NF just required an overpotential of 297 mV at 10 mA cm−2. The observed Tafel slope was about 89 mV dec−1 compared to NF and CuO/NF catalysts. Here, during OER, in situ generated Cu(OH)2 layer acted as a real catalyst surface for facilitating O–O bond formation. Similarly, for HER, the required overpotential at 10 mA cm−2 was nearly 162 mV and the Tafel slope value was nearly 129 mV dec−1. Here, during HER, the amorphous Cu(OH)2/CuOx phase formed acted as the real catalyst. Hence, along with Se precursors, it could easily pull the protons to the electrode surface to facilitate facile electron transfer for HER. After having come to know the superior OER and HER kinetics, CuSe/NF as the anode and cathode was directed for TWS in 1 M KOH (Fig. 36a–d). To reach the benchmarking current density of 10 mA cm−2, it just required a voltage of 1.68 V. These observations have given a view that in the future, copper-based selenides can be promising candidates for generating hydrogen economically. Also, from the overall view on Cu-based selenides, by utilizing suitable electrodes with the employment of pioneering approaches, it can be further developed from its current stage. With the assurance of activity and durability in both HER and OER at extreme pH conditions, copper-based selenides can be studied in detail for their high rate water electrolysis.
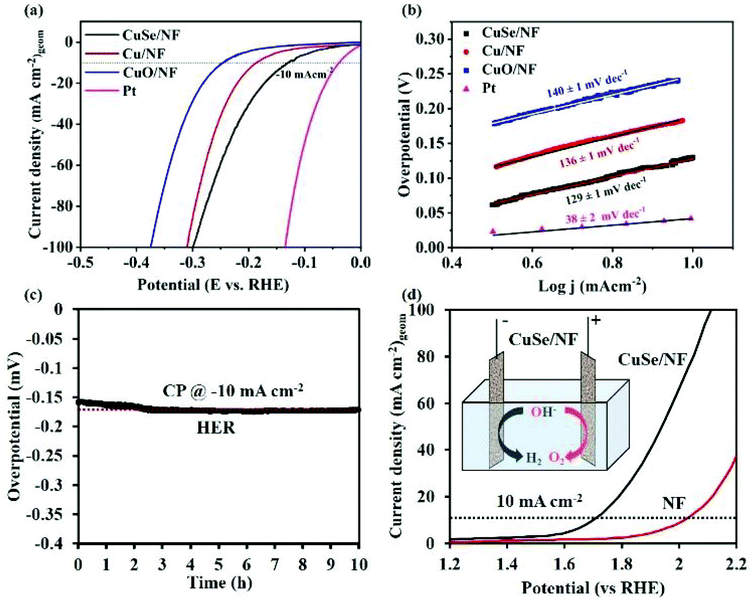 |
| Fig. 36 (a) LSV, (b) Tafel slope, (c) galvanostatic study, and (d) TWS study of CuSe/NF catalysts. Reprinted with permission from ref. 104. Copyright (2020) Wiley-VCH. | |
8. Cu-Based tellurides for HER
Cu-Based tellurides have not been explored much compared to the sulfides and selenides. Recently, Kumaravel et al. highlighted the formation of Cu2−xTe catalysts for HER in acidic electrolyte.106 A comparative study was carried out to relate the activities and here, wet-chemical and hydrothermal methods were adapted to prepare Cu2−xTe catalysts. NaBH4 and Te salts were taken with Cu metal precursors and at both room temperature and hydrothermal conditions, Cu2−xTe was formed successfully for the first time. The equal distribution of Cu and Te can be evidenced from the observed HAADF mapping mode (Fig. 37a–e). Still, the oxide formation is inevitable here, which could retard the potential in HER studies. After this, HER study was carried out to relate the performance of telluride-based Cu for delivering activity and durability. The HER performance is remarkable and in reaching the current density of 10 mA cm−2, the prepared Cu2−xTe/hyd showed high activity by acquiring an overpotential of 347 mV, whereas Cu2−xTe/wc required 432 mV for the same current density. Similarly, the facile charge transport phenomenon was encountered more in Cu2−xTe/hyd, which showed a Tafel slope value of 188 mV dec−1. From the EIS and ECSA calculations, it has been found that Cu2−xTe/hyd exhibited enhanced electron transfer kinetics with a highly exposed active surface area. The stability was assessed further with potentiostatic analysis, which showed high stability for 12 h with less deviation in the activity (Fig. 38a–d). This study proved the necessity of Cu-based tellurides for the water reduction potential with the adaptation of the following different synthetic strategies. The activities of these kinds of Cu-based tellurides can further be improved by adopting conducting supports, growing over 3D structures, increasing the metallic sites, and by extending with other transition metal-based tellurides, as we can see from the previous discussions on Cu-based selenides. Therefore, it is highly desirable to work on Cu = based tellurides to bring down the energy inputs for both OER and HER. In a work by Majhi et al., nanocomposites of CoTe2@CuTe and FeTe2@CuTe were developed by the hydrothermal method and assessed for HER in extreme pH conditions such as acidic, alkaline, and also in neutral electrolytes.107 In the HER studies under acidic conditions, the CoTe2@CuTe catalyst just required 58.1 mV as the overpotential to reach 10 mA cm−2, whereas FeTe2@CuTe required an 86 mV overpotential. When they compared this with the selenides, namely, CoSe2@ CuSe2 and FeSe2@ CuSe2, still the overpotential required was large with the values of 117 and 127.7 mV, respectively. This showed the influence of copper-based tellurides with other transition metal tellurides in HER compared to the selenide counterpart of the same metals. Under neutral conditions as well, CoTe2@CuTe required a lesser overpotential of 125 mV compared to that of FeTe2@CuTe of about 203 mV at the same current density. Here too, the selenide counterparts required higher overpotentials of 225 and 252 mV, which is far higher than that required by tellurides. Under alkaline conditions, CoTe2@CuTe and FeTe2@CuTe required overpotentials of 106 and 122 mV at 10 mA cm−2, whereas selenides of the same required 129 and 180 mV overpotentials. The extended activity with CoTe2@CuTe and FeTe2@CuTe has been attributed to the high ECSA and BET surface area compared to that of the selenides and the activities are consistent with that of the tellurides at pH 0, 7, and 14. Moreover, the synergy between the tellurides of Co, Cu, and Fe is comparable to the selenides; hence, these telluride-based composites are highly demanded to work in HER in the future. Here, this study has enabled high activity and durability with nanocomposites of Cu-based tellurides with both Co and Fe. In the future, other transition metal-based tellurides can be adjoined with these Cu-based tellurides for extended activity in HER applications in acidic environments.
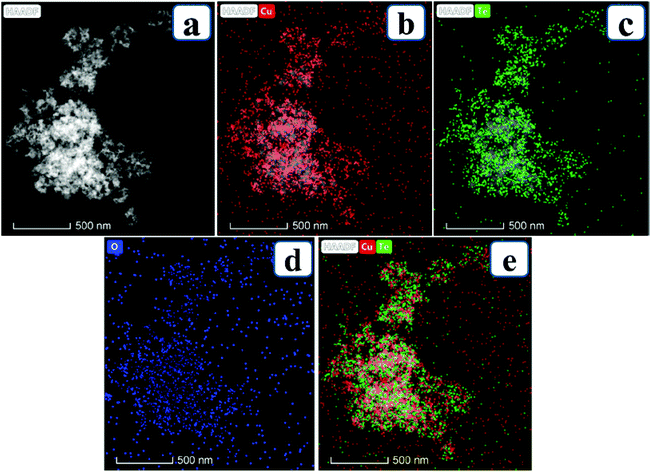 |
| Fig. 37 HAADF color mapping for confirming the presence of Cu and Te in Cu2−xTe. | |
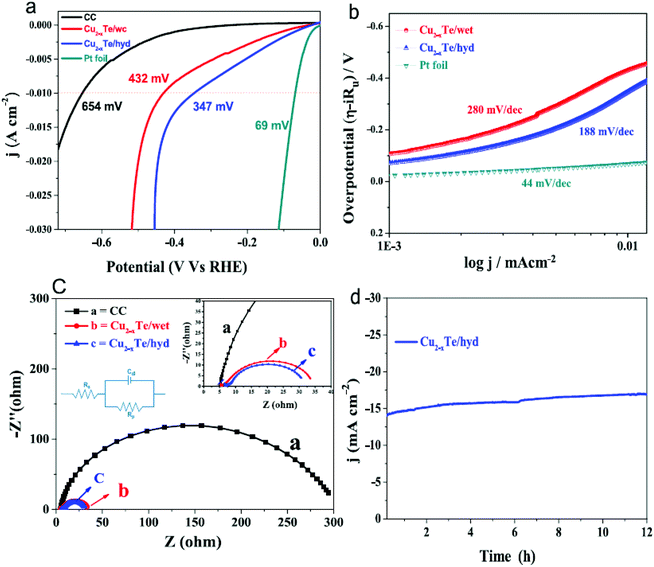 |
| Fig. 38 (a) LSV, (b) Tafel slope, (c) EIS study, and (d) PSTAT study. | |
9. Cu-Based phosphides for OER and HER
Phosphides of Cu have been studied extensively for electrocatalytic water splitting because of its metalloid nature with high electrical conductivity properties. Phosphide-based transition metal catalysts have been found to show enhancement in the OER because of the synergistic enhancement. In HER as well, the presence of phosphide (P3−) ions can effectively anchor protons with a high rate activity. Tian et al. showed the formation of Cu3P nanowire arrays over Cu foam, which has been used as an electrode for HER in acid.108 The increased HER activity was ascribed to the Cu and pendant base P, which could effectively catalyze the reaction with ease. For comparison, Cu3P microparticles were also compared. For reaching 10 mA cm−2 current density, Cu3P NA/CF showed an onset potential of 62 mV, whereas Cu3P MP/CF required 190 mV overpotential as the onset potential. The electron transport was facile in Cu3P NA/CF, which showed 67 mV dec−1, whereas Cu3P MP/CF demanded 96 mV dec−1. These findings exhibit the effect of the nanostructures, which have a large number of electrochemically accessible active sites for HER. From the stability study, it was confirmed that these Cu3P-based catalysts could show high rate stability and the LSV curve after cycling showed very less degradation of the activity (Fig. 39). The HER activity of these Cu-based phosphides can be enhanced by the addition of other transition metal-based phosphides. In an interesting work carried out by Chu et al., the HER activity was enhanced with Ni2−xCux-P nanosheets in alkaline medium.109 Here, NiCu LDH was formulated over Ni foam, which on subsequent phosphorization resulted in Ni2−xCux-P/NF and was applied for HER (Fig. 40). The NiCu LDH is poor in HER and required an overpotential of 136 mV. Also, only Ni2P and Cu3P required comparatively low overpotentials of 103 mV and 117 mV, respectively. This is still higher than that of NiCu LDH, thus showing improvisation in the activity with phosphorization. The stoichiometries of Ni and Cu were varied in the synthesis of Ni2−xCux-P/NF and among them, Ni1.8Cu0.8P/NF showed the highest activity and the required a very small overpotential of 78 mV for 10 mA cm−2. Also, the observed Tafel slope is very less at 70 mV dec−1 compared to that of NiCu LDH and mono-metallic phosphides. Moreover, the stability of these bimetallic phosphides is highly admirable and no noticeable deviation was observed after the cycling study. Here, the influence Cu is to modify the Fermi level and to effectively assist the desorption of H2 molecule. From these observations of Ni2−xCux-P/NF as effective catalysts in HER, other transition metals can also be added and with a variation in the stoichiometry, the HER activity can be enhanced in the future. Since other transition metal-based phosphides such as Ni and Co have been studied extensively for both OER and HER, the TWS with Cu-based phosphides will be interesting to apply in order to obtain a reduction in the overpotentials for OER and HER. Han et al. have developed Cu3P/NF by hydrothermal treatment and subsequently phosphorization at high temperatures (Fig. 41a and b).110 For OER, Cu3P/NF required an overpotential of 320 mV at 10 mA cm−2 with a Tafel slope of 54 mV dec−1 in 1 M KOH. Similarly, for HER, the demanded overpotential was just 105 mV and the Tafel slope was nearly 42 mV dec−1. Due to the outstanding OER and HER activities of Cu3P/NF, it has been applied in TWS by keeping it as both the anode and the cathode. The catalytic current density of 10 mA cm−2 was achieved at an overvoltage of 1.67 V, indicating only 440 mV as the overpotential. Moreover, the onset overpotential was just 270 mV for TWS with Cu3P/NF electrodes (Fig. 42a–d). Further, bulk electrolysis for 10 h further confirmed the robustness of Cu3P/NF in industrial water splitting. From this study, the utilization of Cu3P as effective alternative for the other transition metal-based catalysts such as Ni and Co has been explored. From the observed results, it can be seen that the phosphides of Cu can be the better alternative for replacing scarce metals. Moreover, their stability in acidic and alkaline environments further assists their usage as promising alternatives. Moreover, these Cu-based phosphides can be added with other transition metal-based catalysts with different approaches and hence, TWS with Cu-based catalysts can be a better alternative for scarce metals in the future. The activities of Cu-based catalysts for OER and HER have been tabulated and are provided herein as Tables 1 and 2, respectively.
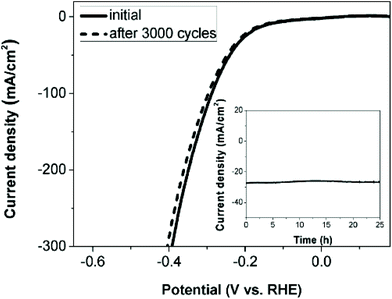 |
| Fig. 39 Stability study of Cu3P/CF electrodes during cycling and PSTAT analysis. Reprinted with permission from ref. 108. Copyright (2014) Wiley-VCH. | |
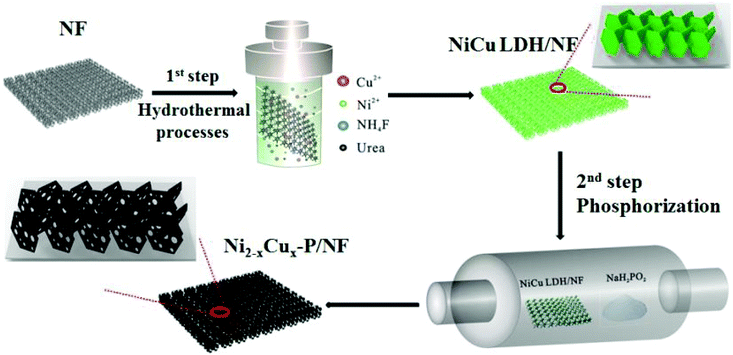 |
| Fig. 40 Scheme showing the formation of NiCu phosphides over NF for HER. Reprinted with permission from ref. 109. Copyright (2018) Elsevier. | |
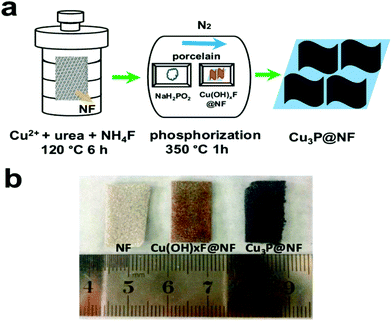 |
| Fig. 41 Scheme showing the formation of Cu3P/NF over NF for TWS. Reprinted with permission from ref. 110. Copyright (2016) American Chemical Society. | |
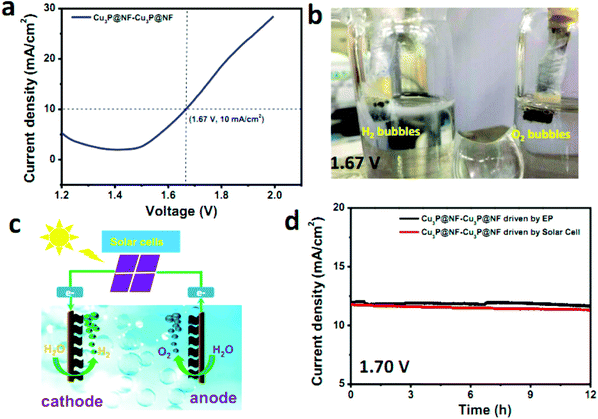 |
| Fig. 42 (a) LSV and (b) the two-electrode set-up for Cu3P catalysts in KOH. (c) The scheme and (d) PSTAT analysis for 12 h. Reprinted with permission from ref. 110. Copyright (2016) American Chemical Society. | |
Table 1 Comparison table of previously reported Cu-based nanostructures for oxygen evolution reaction
S. no. |
Catalyst |
Study and medium |
Overpotential (10 mA cm−2) |
Tafel slope (mV dec−1) |
Ref. |
1 |
CuO nanowires |
OER, 1 M KOH |
430 (1 mA cm−2) |
61.4 |
77
|
2 |
CuO nanosheets bundles |
OER, 1 M KOH |
350 |
59 |
78
|
3 |
CuO NPs |
OER, 1 M NaOH |
290 |
64 |
79
|
4 |
CuO nanosheets |
OER, 1 M KOH |
320 |
135 |
67
|
5 |
CuO nanocubes |
OER, 1 M KOH |
320 |
149 |
67
|
6 |
CuO nanoleaves |
OER, 1 M KOH |
280 |
106 |
67
|
7 |
CuO nanoflowers |
OER, 1 M KOH |
270 |
84 |
67
|
8 |
HS-CuO–C/CF |
OER, 1 M KOH |
286 |
66.3 |
80
|
9 |
CuO-FTO |
OER, 0.2 M phosphate buffer |
500 (1 mA cm−2) |
57 |
81
|
10 |
Co-CuO NA/CF |
OER, 1 M KOH |
299 (50 mA cm−2) |
134 |
71
|
11 |
NiCuOx NPs |
OER, 1 M Na2CO3 |
320 (onset overpotential) |
120 |
82
|
15 |
Cu@CoFe LDH |
OER, 1 M KOH |
240 |
44.4 |
86
|
17 |
Cu@CoFe LDH |
TWS, 1 M KOH |
451 |
— |
86
|
18 |
Cu-NiFe LDH |
OER, 1 M KOH |
199 |
27.8 |
87
|
20 |
Cu-NiFe LDH |
TWS, 1 M KOH |
310 |
— |
87
|
21 |
Cu(OH)2-NiFe LDH |
OER, 1 M KOH |
283 |
88 |
88
|
22 |
SAV-NiCux LDH |
OER, 1 M KOH |
290 |
45 |
89
|
23 |
NiCu LDH/CC |
OER, 1 M KOH |
290 |
42.5 |
90
|
24 |
Cu2S nanorods |
OER, 1 M KOH |
270 |
128 |
92
|
25 |
Cu2S NA-50@CF |
OER, 1 M KOH |
360 (50 mA cm−2) |
75 |
93
|
26 |
Cu2S/TiO2/Cu2S |
OER, 1 M KOH |
284 |
72 |
76
|
27 |
Cu2S microhexagons |
OER, 1 M KOH |
330 |
106.93 |
96
|
29 |
Cu2S NRs@CoS/CF |
OER, 1 M KOH |
275 |
54 |
98
|
32 |
Selenized Cu3Sn@Cu foam |
OER, 1 M KOH |
384 (50 mA cm−2) |
177 |
111
|
33 |
Cu2Se-A/CF |
OER, 1 M KOH |
297 |
72.8 |
99
|
34 |
Cu2Se/CF |
OER, 1 M KOH |
200 |
150 |
100
|
36 |
CuSe/NF |
OER, 1 M KOH |
297 |
89 |
105
|
37 |
CuSe/NF |
OER, 1 M KOH |
162 |
128 |
105
|
38 |
CuSe/NF |
TWS, 1 M KOH |
450 |
— |
105
|
41 |
CoTe2@CuTe |
OER, 1 M PBS |
106 |
67.6 |
107
|
45 |
Cu3P/NF |
OER, 1 M KOH |
320 |
54 |
110
|
47 |
Cu3P/NF |
TWS, 1 M KOH |
440 |
— |
110
|
Table 2 Comparison table of previously reported Cu-based nanostructures for hydrogen evolution reaction
S. no |
Catalyst |
Study and medium |
Overpotential (10 mA cm−2) |
Tafel slope (mV dec−1) |
Ref. |
12 |
Cu/Cu2O–CuO/rGO/CF |
HER, 1 M KOH |
105 |
12 |
84
|
13 |
Cu(poly)-polished |
HER, 1 M KOH |
320 (5 mA cm−2) |
13 |
83
|
14 |
Co3O4–CuO |
HER, 1 M KOH |
288 |
14 |
85
|
16 |
Cu@CoFe LDH |
HER, 1 M KOH |
171 |
16 |
86
|
19 |
Cu-NiFe LDH |
HER, 1 M KOH |
116 |
19 |
87
|
28 |
Cu2S microhexagons |
HER, 1 M KOH |
312 |
28 |
96
|
30 |
Cu2S NRs@CoS/CF |
HER, 1 M KOH |
235 |
30 |
98
|
31 |
Cu2S/MoS2 |
HER, 1 M KOH |
91 |
31 |
97
|
35 |
Cu2Se-ch/CF |
HER, 0.5 M H2SO4 |
212 ± 5 |
35 |
69
|
39 |
Cu2−xTe/hyd |
HER, 0.5 M H2SO4 |
347 |
39 |
106
|
40 |
CoTe2@CuTe |
HER, 0.5 M H2SO4 |
68 |
59.94 |
107
|
42 |
CoTe2@CuTe |
HER, 1 M KOH |
125.8 |
69.53 |
107
|
43 |
Cu3P NW/CF |
HER, 0.5 M H2SO4 |
143 |
67 |
108
|
44 |
Ni1.8Cu0.2-P |
HER, 1 M KOH |
78 |
70 |
109
|
46 |
Cu3P/NF |
HER, 1 M KOH |
105 |
42 |
110
|
10. Conclusions and future perspectives
Here, in this review, the utilization of Cu-based catalysts for OER, HER, and TWS have been extensively investigated. The nanostructures of Cu-based oxides, hydroxides, sulfides, selenides, tellurides, and phosphides have been studied and their merits have been explored in detail. The possibilities of enhancing the activity and durability with Cu-based catalysts were studied, such as enhancing the activity with the formation of nanostructures, growing over 3D conducting supports, enhancing the electrical conductivity with graphene, increasing the metallic active sites by different methods such as electrodeposition, adding other transition metals, and also by varying the stoichiometric ratios. Following are the summarized important observations for the Cu-based catalysts.
• From the observed findings, Cu-based oxides and hydroxides have shown comparably lesser activity in OER and HER. Even though the stability is assured at low current densities, when moving to high current densities, the observed deviation in the activity is still noticed. Growth in conducting supports can bring high stability under prolonged exposure. In Cu-based oxides, CuO and Cu(OH)2 showed commendable activity for OER because of the formation of metastable CuIII ions. In case of HER for Cu-based oxides, since the electrical conductivity is poor, the activity was comparatively lesser.
• Similarly, LDH-based materials of Cu with other transition metals such as Ni have been studied and here, the activity and durability were enhanced for OER. The engineering of Cu with other highly active NiFe and NiCo LDHs was studied and even in TWS, the activity and durability were much pronounced with the presence of Cu, which enhanced the electrical conductivity.
• In case of sulfides, the activity in OER and HER is high compared to oxide-based catalysts. Even though sulfides act as pre-catalysts and it is the oxide or hydroxide phase that is formed during the OER that acts as a real catalyst, the presence of sulfide ions along with the oxide/hydroxide surface could enhance the activity. Moreover, sulfides of Cu were added with other transition metal-based sulfides and applied for both OER and HER with high activity and durability.
• For selenides, the activities are large for OER and HER and with the different methods of approach of selenization, Cu-based selenides have been observed to be highly active catalysts for OER, HER, and in TWS studies. Here also, the influence in OER and HER was enhanced by compositing with other highly active selenides of interest such as Ni, which is highly active in water splitting, as far as 3d transition metal-based catalysts are concerned.
• Tellurides of Cu have been recently explored for HER studies and composites of CuTe with other 3d transition metal-based tellurides such as Ni and Fe under different pH conditions. Still, tellurides of Cu have shown high rate activity and stability but it has to be explored further in the future.
• Cu-Based phosphides have been explored for both OER and HER with impressive activity and durability. Direct growth over 3D conducting supports has ensured an increase in the activity. Because of the synergistic enhancement in OER with phosphide-based catalysts and HER activity with the phosphide ions, total water splitting was ensured with high rate endurance compared to that of oxides and hydroxides.
In the future, Cu-based catalysts can be studied with pioneering modifications such as increase in the metal active sites, doping with other active elements, with conducting supports, and growth over conducting 3D supports. Moreover, vacancy creation in Cu-based structures, especially in sulfides, can be done without disrupting the structure. Selenide-based catalysts can be further enhanced with the stoichiometric variations and the method of synthesis adopted, and also by combining with other active elements. The active nature of phosphides is witnessed well with other transition metal-based catalysts and in Cu-based phosphides as well, enhanced activity and durability have been achieved. From this comprehensive overview on Cu-based catalysts, it is well understood that Cu can be an effective material for empowering water splitting with high activities and stabilities. Further, in different forms such as oxides, hydroxides, LDHs, sulfides, selenides, and phosphides, it has shown prominence as an alternative to noble metals. Hence, in the future, with Cu-based nanostructures, there are many possibilities by which the electrocatalytic activities can be enhanced such as doping with other metals, in situ growth on 3D metal foams, and with conducting supports. There are more structural modifications that can be done to increase the surface active sites with Cu-based catalysts and in the future, these can act as promising alternatives to noble elements for the viable hydrogen production with electrocatalytic water splitting.
Conflicts of interest
There are no conflicts to declare.
Acknowledgements
We wish to acknowledge Dr N. Kalaiselvi, Director, CSIR-CECRI for their continuous support and encouragement. K. Karthick and Sam Sankar wish to acknowledge UGC for SRF award. Arun Karmakar and Sangeetha Kumaravel wish to acknowledge CSIR-JRF and DST INSPIRE fellowship, respectively. S. Kundu wishes to acknowledge the Department of Science and Technology (DST) for HCF (Hydrogen and Fuel Cell) research funding with the number # DST/TMD/HFC/2K18/60 on 7-10-2019 having CSIR-CECRI OM number 18-29-03/(31/19)-TTBD-CSIR-CECRI on 24/10/2019.
References
- N. L. Garland, D. C. Papageorgopoulos and J. M. Stanford, Hydrogen and Fuel Cell Technology: Progress, challenges, and future Directions, Energy Procedia, 2012, 28, 2–11 CrossRef CAS.
- M. Momirlan and T. N. Veziroglu, Current status of hydrogen energy, Renewable Sustainable Energy Rev., 2002, 6, 141–179 CrossRef CAS.
- J. L. Kolar, Alternative energy technologies, Environ. Qual. Manag., 2000, 10, 45–54 CrossRef.
- S. Shafiee and E. Topal, When will fossil fuel reserves be diminished?, Energy Policy, 2009, 37, 181–189 CrossRef.
- J. R. Rostrup-Nielsen, Fuels and energy for the future: the role of catalysis, Catal. Rev. – Sci. Eng., 2004, 46, 247–270 CrossRef CAS.
- S. Anantharaj, S. R. Ede, K. Sakthikumar, K. Karthick, S. Mishra and S. Kundu, Recent trends and perspectives in electrochemical water splitting with an emphasis on sulfide, selenide, and phosphide catalysts of Fe, Co, and Ni: a review, ACS Catal., 2016, 6, 8069–8097 CrossRef CAS.
- T. Reier, M. Oezaslan and P. Strasser, Electrocatalytic oxygen evolution reaction (OER) on Ru, Ir, and Pt catalysts: a comparative study of nanoparticles and bulk materials, ACS Catal., 2012, 2, 1765–1772 CrossRef CAS.
- S. Fierro, T. Nagel, H. Baltruschat and C. Comninellis, Investigation of the oxygen evolution reaction on Ti/IrO2 electrodes using isotope labelling and on-line mass spectrometry, Electrochem. Commun., 2007, 9, 1969–1974 CrossRef CAS.
- W. Hu, Y. Wang, X. Hu, Y. Zhou and S. Chen, Three-dimensional ordered macroporous IrO2 as electrocatalyst for oxygen evolution reaction in acidic medium, J. Mater. Chem., 2012, 22, 6010–6016 RSC.
- A. T. Marshall and R. G. Haverkamp, Electrocatalytic activity of IrO2–RuO2 supported on Sb-doped SnO2 nanoparticles, Electrochim. Acta, 2010, 55, 1978–1984 CrossRef CAS.
- J. X. Feng, S. Y. Tong, Y. X. Tong and G. R. Li, Pt-like hydrogen evolution electrocatalysis on PANI/CoP hybrid nanowires by weakening the shackles of hydrogen ions on the surfaces of catalysts, J. Am. Chem. Soc., 2018, 140, 5118–5126 CrossRef CAS.
- L. Han, S. Dong and E. Wang, Transition metal (Co, Ni, and Fe) based electrocatalysts for the water oxidation reaction, Adv. Mater., 2016, 28, 9266–9291 CrossRef CAS.
- W. T. Hong, M. Risch, K. A. Stoerzinger, A. Grimaud, J. Suntivich and Y. Shao-Horn, Toward the rational design of non-precious transition metal oxides for oxygen electrocatalysis, Energy Environ. Sci., 2015, 8, 1404–1427 RSC.
- S. Anantharaj, K. Karthick and S. Kundu, Evolution of layered double hydroxides (LDH) as high performance water oxidation electrocatalysts: A review with insights on structure, activity and mechanism, Mater. Today Energy, 2017, 6, 1–26 CrossRef.
- J. Landon, E. Demeter, N. Inoglu, C. Keturakis, I. E. Wachs, R. Vasic, A. I. Frenkel and J. R. Kitchin, Spectroscopic characterization of mixed Fe–Ni oxide electrocatalysts for the oxygen evolution reaction in alkaline electrolytes, ACS Catal., 2012, 2, 1793–1801 CrossRef CAS.
- M. Gong, W. Zhou, M. C. Tsai, J. Zhou, M. Guan, M. C. Lin, B. Zhang, Y. Hu, D. Y. Wang, J. Yang, S. J. Pennycook, B. J. Hwang and H. Dai, Nanoscale nickel oxide/nickel heterostructures for active hydrogen evolution electrocatalysis, Nat. Commun., 2014, 5, 1–6 Search PubMed.
- J. Yin, P. Zhou, L. An, L. Huang, C. Shao, J. Wang, H. Liu and P. Xi, Self-supported nanoporous NiCo2O4 nanowires with cobalt–nickel layered oxide nanosheets for overall water splitting, Nanoscale, 2016, 8, 1390–1400 RSC.
- J. Chi, H. Yu, G. Li, L. Fu, J. Jia, X. Gao, B. Yi and Z. Shao, Nickel/cobalt oxide as a highly efficient OER electrocatalyst in an alkaline polymer electrolyte water electrolyzer, RSC Adv., 2016, 6, 90397–90400 RSC.
- C. Zhang, B. Xin, S. Duan, A. Jiang, B. Zhang, Z. Li and J. Hao, Controllable 1D and 2D cobalt oxide and cobalt selenide nanostructures as highly efficient electrocatalysts for the oxygen evolution reaction, Chem. – Asian J., 2018, 13, 2700–2707 CrossRef CAS.
- L. Liardet and X. Hu, Amorphous cobalt vanadium oxide as a highly active electrocatalyst for oxygen evolution, ACS Catal., 2018, 8, 644–650 CrossRef CAS.
- K. Fominykh, P. Chernev, I. Zaharieva, J. Sicklinger, G. Stefanic, M. Doblinger, A. Muller, A. Pokharel, S. Bocklein, C. Scheu, T. Bein and D. Fattakhova-Rohlfing, Iron-doped nickel oxide nanocrystals as highly efficient electrocatalysts for alkaline water splitting, ACS Nano, 2015, 9, 5180–5188 CrossRef CAS.
- J. Qi, W. Zhang, R. Xiang, K. Liu, H. Y. Wang, M. Chen, Y. Han and R. Cao, Porous Nickel-Iron Oxide as a Highly Efficient Electrocatalyst for Oxygen Evolution Reaction, Adv. Sci., 2015, 2, 1–8 Search PubMed.
- B. Bayatsarmadi, Y. Zheng, C. S. S. Casari, V. Russo and S. Qiao, Pulsed laser deposition of porous N-carbon supported cobalt (oxide) thin films for highly efficient oxygen evolution, Chem. Commun., 2016, 52, 11947–11950 RSC.
- H. Jin, J. Wang, D. Su, Z. Wei, Z. Pang and Y. Wang, In situ cobalt–cobalt oxide/N-doped carbon hybrids as superior bifunctional electrocatalysts for hydrogen and oxygen evolution, J. Am. Chem. Soc., 2015, 137, 2688–2694 CrossRef CAS.
- T. Gao, Z. Jin, M. Liao, J. Xiao, H. Yuan and D. Xiao, A trimetallic V–Co–Fe oxide nanoparticle as an efficient and stable electrocatalyst for oxygen evolution reaction, J. Mater. Chem. A, 2015, 3, 17763–17770 RSC.
- M. Gong, Y. Li, H. Wang, Y. Liang, J. Z. Wu, J. Zhou, J. Wang, T. Regier, F. Wei and H. Dai, An advanced Ni–Fe layered double hydroxide electrocatalyst for water oxidation, J. Am. Chem. Soc., 2013, 135, 8452–8455 CrossRef CAS.
- K. Y. Ma, J. P. Cheng, F. Liu and X. Bin Zhang, Co-Fe layered double hydroxides nanosheets vertically grown on carbon fiber cloth for electrochemical capacitors, J. Alloys Compd., 2016, 679, 277–284 CrossRef CAS.
- H. Liang, A. N. Gandi, C. Xia, M. N. Hedhili, D. H. Anjum, U. Schwingenschlogl and H. N. Alshareef, Amorphous NiFe-OH/NiFeP electrocatalyst fabricated at low temperature for water oxidation applications, ACS Energy Lett., 2017, 2, 1035–1042 CrossRef CAS.
- M. S. Burke, M. G. Kast, L. Trotochaud, A. M. Smith and S. W. Boettcher, Cobalt–iron (oxy) hydroxide oxygen evolution electrocatalysts: the role of structure and composition on activity, stability, and mechanism, J. Am. Chem. Soc., 2015, 137, 3638–3648 CrossRef CAS.
- X. L. Guo, X. Y. Liu, X. D. Hao, S. J. Zhu, F. Dong, Z. Q. Wen and Y. X. Zhang, Nickel-manganese layered double hydroxide nanosheets supported on nickel foam for high-performance supercapacitor electrode materials, Electrochim. Acta, 2016, 194, 179–186 CrossRef CAS.
- Y. Ni, L. Yao, Y. Wang, B. Liu, M. Cao and C. Hu, Construction of hierarchically porous graphitized carbon-supported NiFe layered double hydroxides with a core–shell structure as an enhanced electrocatalyst for the oxygen evolution reaction, Nanoscale, 2017, 9, 11596–11604 RSC.
- H. M. Sun, Y. X. Ye, Z. F. Tian, S. L. Wu, J. Liu and C. H. Liang, Ni 3+ doped cobalt–nickel layered double hydroxides as high-performance electrode materials for supercapacitors, RSC Adv., 2017, 7, 49010–49014 RSC.
- L. J. Foruzin, Z. Rezvani, Y. H. Shishavan and B. Habibi, Ni2Zn0. 5Fe-LDH modified carbon paste electrode as an efficient electrocatalyst for water oxidation in neutral media, Int. J. Hydrogen Energy, 2017, 1–11 Search PubMed.
- M. S. Burke, M. G. Kast, L. Trotochaud, A. M. Smith and S. W. Boettcher, Cobalt–iron (oxy) hydroxide oxygen evolution electrocatalysts: the role of structure and composition on activity, stability, and mechanism, J. Am. Chem. Soc., 2015, 137, 3638–3648 CrossRef CAS.
- W. Ma, R. Ma, C. Wang, J. Liang, X. Liu, K. Zhou and T. Sasaki, A superlattice of alternately stacked Ni–Fe hydroxide nanosheets and graphene for efficient splitting of water, ACS Nano, 2015, 9, 1977–1984 CrossRef CAS.
- X. Gao, X. Long, H. Yu, X. Pan and Z. Yi, Ni nanoparticles decorated NiFe layered double hydroxide as bifunctional electrochemical catalyst, J. Electrochem. Soc., 2017, 164, H307–H310 CrossRef CAS.
- P. F. Liu, S. Yang, B. Zhang and H. G. Yang, Defect-rich ultrathin cobalt–iron layered double hydroxide for electrochemical overall water splitting, ACS Appl. Mater. Interfaces, 2016, 8, 34474–34481 CrossRef CAS.
- H. Chen, L. Hu, M. Chen, Y. Yan and L. Wu, Nickel–cobalt layered double hydroxide nanosheets for high performance supercapacitor electrode materials, Adv. Funct. Mater., 2014, 24, 934–942 CrossRef CAS.
- Y. Shi and B. Zhang, Recent advances in transition metal phosphide nanomaterials: synthesis and applications in hydrogen evolution reaction, Chem. Soc. Rev., 2016, 45, 1529–1541 RSC.
- S. Jin, Are metal chalcogenides, nitrides, and phosphides oxygen evolution catalysts or bifunctional catalysts?, ACS Energy Lett., 2017, 2, 1937–1938 CrossRef CAS.
- A. Sumboja, T. An, H. Y. Goh, M. Lübke, D. P. Howard, Y. Xu, A. D. Handoko, Y. Zong and Z. Liu, One-step facile synthesis of cobalt phosphides for hydrogen evolution reaction catalysts in acidic and alkaline medium, ACS Appl. Mater. Interfaces, 2018, 10, 15673–15680 CrossRef CAS.
- X. Zou and Y. Zhang, Noble metal-free hydrogen evolution catalysts for water splitting, Chem. Soc. Rev., 2015, 44, 5148–5180 RSC.
- M. Zeng and Y. Li, Recent advances in heterogeneous electrocatalysts for the hydrogen evolution reaction, J. Mater. Chem. A, 2015, 3, 14942–14962 RSC.
- J. Xu, J. Li, D. Xiong, B. Zhang, Y. Liu, K. H. Wu, I. Amorim, W. Li and L. Liu, Trends in activity for the oxygen evolution reaction on transition metal (M = Fe, Co, Ni) phosphide pre-catalysts, Chem. Sci., 2018, 9, 3470–3476 RSC.
- J. F. Callejas, J. M. McEnaney, C. G. Read, J. C. Crompton, A. J. Biacchi, E. J. Popczun, T. R. Gordon, N. S. Lewis and R. E. Schaak, Electrocatalytic and photocatalytic hydrogen production from acidic and neutral-pH aqueous solutions using iron phosphide nanoparticles, ACS Nano, 2014, 8, 11101–11107 CrossRef CAS.
- J. Joo, T. Kim, J. Lee, S. Il Choi and K. Lee, Morphology-Controlled Metal Sulfides and Phosphides for Electrochemical Water Splitting, Adv. Mater., 2019, 31, 1–23 CrossRef.
- T. Guo, Y. Song, Z. Sun, Y. Wu, Y. Xia, Y. Li, J. Sun, K. Jiang, S. Dou and J. Sun, Bio-templated formation of defect-abundant VS2 as a bifunctional material toward high-performance hydrogen evolution reactions and lithium−sulfur batteries, J. Energy Chem., 2020, 42, 34–42 CrossRef.
- J. Huang, S. Wen, G. Chen, W. Chen, G. Wang, H. Fan, D. Chen, C. Song, M. Li, X. Wang, L. Li, M. Tao, B. Li, X. Wang and K. Ostrikov, Multiphase Ni-Fe-selenide nanosheets for highly-efficient and ultra-stable water electrolysis, Appl. Catal., B, 2020, 277, 119220 CrossRef CAS.
- Z. Gao, J. Qi, M. Chen, W. Zhang and R. Cao, An Electrodeposited NiSe for Electrocatalytic Hydrogen and Oxygen Evolution Reactions in Alkaline Solution, Electrochim. Acta, 2017, 224, 412–418 CrossRef CAS.
- Q. Zhao, D. Zhong, L. Liu, D. Li, G. Hao and J. Li, Facile fabrication of robust 3D Fe-NiSe nanowires supported on nickel foam as a highly efficient, durable oxygen evolution catalyst, J. Mater. Chem. A, 2017, 5, 14639–14645 RSC.
- Y. Shi, Y. Wan, R. Liu, B. Tu and D. Zhao, Synthesis of highly ordered mesoporous crystalline WS2 and MoS2 via a high-temperature reductive sulfuration route, J. Am. Chem. Soc., 2007, 129, 9522–9531 CrossRef CAS.
- Q. Liu, Q. Shang, A. Khalil, Q. Fang, S. Chen, Q. He, T. Xiang, D. Liu, Q. Zhang, Y. Luo and L. Song, In situ Integration of a Metallic 1T-MoS2/CdS Heterostructure as a means To Promote Visible-Light-Driven Photocatalytic Hydrogen Evolution, ChemCatChem, 2016, 8, 2614–2619 CrossRef CAS.
- Y. Yang, K. Zhang, H. Lin, X. Li, H. C. Chan, L. Yang and Q. Gao, Heteronanorods as Efficient and Stable Bifunctional Electrocatalysts for Overall Water Splitting, ACS Catal., 2017, 7, 2357–2366 CrossRef CAS.
- Q. Liu, Q. Fang, W. Chu, Y. Wan, X. Li, W. Xu, M. Habib, S. Tao, Y. Zhou, D. Liu, T. Xiang, A. Khalil, X. Wu, M. Chhowalla, P. M. Ajayan and L. Song, Electron-Doped 1T-MoS2 via Interface Engineering for Enhanced Electrocatalytic Hydrogen Evolution, Chem. Mater., 2017, 29, 4738–4744 CrossRef CAS.
- Q. He, X. Chen, S. Chen, L. Liu, F. Zhou, X. B. Li and G. Wang, Electrochemical Hydrogen Evolution at the Interface of Monolayer VS2 and Water from First-Principles Calculations, ACS Appl. Mater. Interfaces, 2019, 11, 2944–2949 CrossRef CAS.
- H. Wu, X. Lu, G. Zheng and G. W. Ho, Topotactic Engineering of Ultrathin 2D Nonlayered Nickel Selenides for Full Water Electrolysis, Adv. Energy Mater., 2018, 8, 1–9 Search PubMed.
- J. Y. Zhang, L. Lv, Y. Tian, Z. Li, X. Ao, Y. Lan, J. Ji and C. Wang, Rational design of cobalt-iron selenides for highly efficient electrochemical water oxidation, ACS Appl. Mater. Interfaces, 2017, 9, 33833–33840 CrossRef CAS.
- X. Xu, F. Song and X. Hu, A nickel iron diselenide-derived efficient oxygen-evolution catalyst, Nat. Commun., 2016, 7, 1–7 Search PubMed.
- Q. Zhang, W. Chen, G. Chen, J. Huang, C. Song, S. Chu, R. Zhang, G. Wang, C. Li and K. K. Ostrikov, Bi-metallic nitroxide nanodot-decorated tri-metallic sulphide nanosheets by on-electrode plasma-hydrothermal sprouting for overall water splitting, Appl. Catal., B, 2020, 261, 118254 CrossRef CAS.
- D. Chen, Z. Xu, W. Chen, G. Chen, J. Huang, J. Huang, C. Song, C. Li, K. (Ken) Ostrikov and K. (Ken) Ostrikov, Just add water to split water: ultrahigh-performance bifunctional electrocatalysts fabricated using eco-friendly heterointerfacing of NiCo diselenides, J. Mater. Chem. A, 2020, 8, 12035–12044 RSC.
- G. Wang, W. Chen, G. Chen, J. Huang, C. Song, D. Chen, Y. Du, C. Li and K. K. Ostrikov, Trimetallic Mo–Ni–Co selenides nanorod electrocatalysts for highly-efficient and ultra-stable hydrogen evolution, Nano Energy, 2020, 71, 104637 CrossRef CAS.
- D. Chen, Z. Xu, W. Chen, G. Chen, J. Huang, C. Song, K. Zheng, Z. Zhang, X. Hu, H. Choi and K. (Ken) Ostrikov, Mulberry–Inspired Nickel–Niobium Phosphide on Plasma–Defect–Engineered Carbon Support for High–Performance Hydrogen Evolution, Small, 2020, 2004843 CrossRef CAS.
- Y. Lee, J. Suntivich, K. J. May, E. E. Perry and Y. Shao-Horn, Synthesis and Activities of Rutile IrO2 and RuO2 Nanoparticles for Oxygen Evolution in Acid and Alkaline Solutions, J. Phys. Chem. Lett., 2012, 3, 399–404 CrossRef CAS.
- J. Zhang, T. Wang, D. Pohl, B. Rellinghaus, R. Dong, S. Liu, X. Zhuang and X. Feng, Interface Engineering of MoS2/Ni3S2 Heterostructures for Highly Enhanced Electrochemical Overall-Water-Splitting Activity, Angew. Chem., Int. Ed., 2016, 55, 6702–6707 CrossRef CAS.
- C. C. Hou, C. J. Wang, Q. Q. Chen, X. J. Lv, W. F. Fu and Y. Chen, Rapid synthesis of ultralong Fe(OH)3:Cu(OH)2 core-shell nanowires self-supported on copper foam as a highly efficient 3D electrode for water oxidation, Chem. Commun., 2016, 52, 14470–14473 RSC.
- Z. Chen, C. X. Kronawitter, X. Yang, Y. W. Yeh, N. Yao and B. E. Koel, The promoting effect of tetravalent cerium on the oxygen evolution activity of copper oxide catalysts, Phys. Chem. Chem. Phys., 2017, 19, 31545–31552 RSC.
- F. Arshad, A. Munir, Q. Q. Kashif, T. ul Haq, J. Iqbal, F. Sher and I. Hussain, Controlled development of higher-dimensional nanostructured copper oxide thin films as binder free electrocatalysts for oxygen evolution reaction, Int. J. Hydrogen Energy, 2020, 45, 16583–16590 CrossRef CAS.
- J. Kang, J. Chen, J. Sheng, J. Xie, X. Z. Fu, R. Sun and C. P. Wong, Pd Nanoparticle-Interspersed Hierarchical Copper Hydroxide@Nickel Cobalt Hydroxide Carbonate Tubular Arrays as Efficient Electrocatalysts for Oxygen Evolution Reaction, ACS Sustainable Chem. Eng., 2019, 7, 16459–16466 CrossRef CAS.
- S. Anantharaj, T. S. Amarnath, E. Subhashini, S. Chatterjee, K. C. Swaathini, K. Karthick and S. Kundu, Shrinking the Hydrogen Overpotential of Cu by 1 v and Imparting Ultralow Charge Transfer Resistance for Enhanced H2 Evolution, ACS Catal., 2018, 8, 5686–5697 CrossRef CAS.
- L. Gan, L. Hu, H. An, J. Fang, Y. Lai and J. Li, Sequential Engineering of Ternary CuFeNi with a Vertically Layered Structure for Efficient and Bifunctional Catalysis of the Oxygen and Hydrogen Evolution Reactions, ACS Appl. Mater. Interfaces, 2018, 10, 41465–41470 CrossRef CAS.
- X. Xiong, C. You, Z. Liu, A. M. Asiri and X. Sun, Co-Doped CuO Nanoarray: An Efficient Oxygen Evolution Reaction Electrocatalyst with Enhanced Activity, ACS Sustainable Chem. Eng., 2018, 6, 2883–2887 CrossRef CAS.
- S. Anantharaj, S. R. Ede, K. Karthick, S. Sam Sankar, K. Sangeetha, P. E. Karthik and S. Kundu, Precision and correctness in the evaluation of electrocatalytic water splitting: Revisiting activity parameters with a critical assessment, Energy Environ. Sci., 2018, 11, 744–771 RSC.
- H. Dau, C. Limberg, T. Reier, M. Risch, S. Roggan and P. Strasser, The Mechanism of Water Oxidation:
From Electrolysis via Homogeneous to Biological Catalysis, ChemCatChem, 2010, 2, 724–761 CrossRef CAS.
- J. O. Bockris and E. C. Potter, The Mechanism of the Cathodic Hydrogen Evolution Reaction, J. Electrochem. Soc., 2007, 99, 169 CrossRef.
- S. Anantharaj and S. Kundu, Do the Evaluation Parameters Reflect Intrinsic Activity of Electrocatalysts in Electrochemical Water Splitting?, ACS Energy Lett., 2019, 1260–1264 CrossRef CAS.
- Y. Deng, A. D. Handoko, Y. Du, S. Xi and B. S. Yeo, In Situ Raman Spectroscopy of Copper and Copper Oxide Surfaces during Electrochemical Oxygen Evolution Reaction: Identification of CuIII Oxides as Catalytically Active Species, ACS Catal., 2016, 6, 2473–2481 CrossRef CAS.
- X. Liu, S. Cui, Z. Sun, Y. Ren, X. Zhang and P. Du, Self-Supported Copper Oxide Electrocatalyst for Water Oxidation at Low Overpotential and Confirmation of Its Robustness by Cu K-Edge X-ray Absorption Spectroscopy, J. Phys. Chem. C, 2016, 120, 831–840 CrossRef CAS.
- S. M. Pawar, B. S. Pawar, B. Hou, J. Kim, A. T. Aqueel Ahmed, H. S. Chavan, Y. Jo, S. Cho, A. I. Inamdar, J. L. Gunjakar, H. Kim, S. Cha and H. Im, Self-assembled two-dimensional copper oxide nanosheet bundles as an efficient oxygen evolution reaction (OER) electrocatalyst for water splitting applications, J. Mater. Chem. A, 2017, 5, 12747–12751 RSC.
- T. N. Huan, G. Rousse, S. Zanna, I. T. Lucas, X. Xu, N. Menguy, V. Mougel and M. Fontecave, A Dendritic Nanostructured Copper Oxide Electrocatalyst for the Oxygen Evolution Reaction, Angew. Chem., Int. Ed., 2017, 56, 4792–4796 CrossRef CAS.
- B. Zhang, C. Li, G. Yang, K. Huang, J. Wu, Z. Li, X. Cao, D. Peng, S. Hao and Y. Huang, Nanostructured CuO/C Hollow Shell@3D Copper Dendrites as a Highly Efficient Electrocatalyst for Oxygen Evolution Reaction, ACS Appl. Mater. Interfaces, 2018, 10, 23807–23812 CrossRef CAS.
- J. Wang, L. Zhu, L. Ji and Z. Chen, Preparation of nanostructured Cu(OH)2 and CuO electrocatalysts for water oxidation by electrophoresis deposition, J. Mater. Res., 2018, 33, 581–589 CrossRef CAS.
- L. Wang, X. Ge, Y. Li, J. Liu, L. Huang, L. Feng and Y. Wang, Nickel enhanced the catalytic activities of amorphous copper for the oxygen evolution reaction, J. Mater. Chem. A, 2017, 5, 4331–4334 RSC.
- P. Farinazzo Bergamo Dias Martins, P. Papa Lopes, E. A. Ticianelli, V. R. Stamenkovic, N. M. Markovic and D. Strmcnik, Hydrogen evolution reaction on copper: Promoting water dissociation by tuning the surface oxophilicity, Electrochem. Commun., 2019, 100, 30–33 CrossRef.
- L. Ye and Z. Wen, Self-supported three-dimensional Cu/Cu2O-CuO/rGO nanowire array electrodes for an efficient hydrogen evolution reaction, Chem. Commun., 2018, 54, 6388–6391 RSC.
- A. Tahira, Z. H. Ibupoto, M. Willander and O. Nur, Advanced Co3O4–CuO nano-composite based electrocatalyst for efficient hydrogen evolution reaction in alkaline media, Int. J. Hydrogen Energy, 2019, 44, 26148–26157 CrossRef CAS.
- L. Yu, H. Zhou, J. Sun, F. Qin, D. Luo, L. Xie, F. Yu, J. Bao, Y. Li, Y. Yu, S. Chen and Z. Ren, Hierarchical Cu@CoFe layered double hydroxide core-shell nanoarchitectures as bifunctional electrocatalysts for efficient overall water splitting, Nano Energy, 2017, 41, 327–336 CrossRef CAS.
- L. Yu, H. Zhou, J. Sun, F. Qin, F. Yu, J. Bao, Y. Yu, S. Chen and Z. Ren, Cu nanowires shelled with NiFe layered double hydroxide nanosheets as bifunctional electrocatalysts for overall water splitting, Energy Environ. Sci., 2017, 10, 1820–1827 RSC.
- X. Ma, X. Li, A. D. Jagadale, X. Hao, A. Abudula and G. Guan, Fabrication of Cu(OH)2@NiFe-layered double hydroxide catalyst array for electrochemical water splitting, Int. J. Hydrogen Energy, 2016, 41, 14553–14561 CrossRef CAS.
- Y. S. Xie, Z. Wang, M. Ju, X. Long and S. Yang, Dispersing transition metal vacancies in layered double hydroxides by ionic reductive complexation extraction for efficient water oxidation, Chem. Sci., 2019, 10, 8354–8359 RSC.
- Y. Zheng, J. Qiao, J. Yuan, J. Shen, A. jun Wang, P. Gong, X. Weng and L. Niu, Three-dimensional NiCu layered double hydroxide nanosheets array on carbon cloth for enhanced oxygen evolution, Electrochim. Acta, 2018, 282, 735–742 CrossRef CAS.
- S. Hu, Y. Tan, C. Feng, H. Wu, J. Zhang and H. Mei, Synthesis of N doped NiZnCu-layered double hydroxides with reduced graphene oxide on nickel foam as versatile electrocatalysts for hydrogen production in hybrid-water electrolysis, J. Power Sources, 2020, 453, 227872 CrossRef CAS.
- S. B. Kale, P. T. Babar, J. H. Kim and C. D. Lokhande, Synthesis of one dimensional Cu2S nanorods using a self-grown sacrificial template for the electrocatalytic oxygen evolution reaction (OER), New J. Chem., 2020, 44, 8771–8777 RSC.
- Y. Wang, Z. Ge, X. Li, J. Zhao, B. Ma and Y. Chen, Cu2S nanorod arrays with coarse surfaces to enhance the electrochemically active surface area for water oxidation, J. Colloid Interface Sci., 2020, 567, 308–315 CrossRef CAS.
- L. He, D. Zhou, Y. Lin, R. Ge, X. Hou, X. Sun and C. Zheng, Ultrarapid in Situ Synthesis of Cu2S Nanosheet Arrays on Copper Foam with Room-Temperature-Active Iodine Plasma for Efficient and Cost-Effective Oxygen Evolution, ACS Catal., 2018, 8, 3859–3864 CrossRef CAS.
- L. An, P. Zhou, J. Yin, H. Liu, F. Chen, H. Liu, Y. Du and P. Xi, Phase transformation fabrication of a Cu2S nanoplate as an efficient catalyst for water oxidation with glycine, Inorg. Chem., 2015, 54, 3281–3289 CrossRef CAS.
- K. S. Bhat and H. S. Nagaraja, Hydrogen evolution reaction at extreme pH conditions of copper sulfide micro-hexagons, J. Sci.: Adv. Mater. Devices, 2020, 3, 361–367 Search PubMed.
- X. Wang, J. Wang, X. Zhang, Q. Tian, M. Liu, N. Cai, Y. Xue, W. Chen, W. Li and F. Yu, Nitrogen-Doped Cu2S/MoS2 Heterojunction Nanorod Arrays on Copper Foam for Efficient Hydrogen Evolution Reaction, ChemCatChem, 2019, 11, 1354–1361 CrossRef CAS.
- Q. Zhou, T. T. Li, J. Wang, F. Guo and Y. Q. Zheng, Hierarchical Cu2S NRs@CoS core-shell structure and its derivative towards synergistic electrocatalytic water splitting, Electrochim. Acta, 2019, 296, 1035–1041 CrossRef CAS.
- X. Wang, X. Hou, H. Lee, L. Lu, X. Wu and L. Sun, Copper Selenide–Derived Copper Oxide Nanoplates as a Durable and Efficient Electrocatalyst for Oxygen Evolution Reaction, Energy Technol., 2020, 8, 1–6 CAS.
- W. Shi and J. Lian, Facile synthesis of copper selenide with fluffy intersected-nanosheets decorating nanotubes structure for efficient oxygen evolution reaction, Int. J. Hydrogen Energy, 2019, 44, 22983–22990 CrossRef CAS.
- J. Masud, W. P. R. Liyanage, X. Cao, A. Saxena and M. Nath, Copper Selenides as High-Efficiency Electrocatalysts for Oxygen Evolution Reaction, ACS Appl. Energy Mater., 2018, 1, 4075–4083 CrossRef CAS.
- W. Zhang, D. Fu, Y. Bai and C. Yuan, In situ anion exchange synthesis of copper selenide electrode as electrocatalyst for hydrogen evolution reaction, Int. J. Hydrogen Energy, 2017, 42, 10925–10930 CrossRef CAS.
- S. Anantharaj, T. S. Amarnath, E. Subhashini, S. Chatterjee, K. C. Swaathini, K. Karthick and S. Kundu, Shrinking the Hydrogen Overpotential of Cu by 1 v and Imparting Ultralow Charge Transfer Resistance for Enhanced H2 Evolution, ACS Catal., 2018, 8, 5686–5697 CrossRef CAS.
- Y. Gao, Y. Wu, H. He and W. Tan, Potentiostatic electrodeposition of Ni–Se–Cu on nickel foam as an electrocatalyst for hydrogen evolution reaction, J. Colloid Interface Sci., 2020, 578, 555–564 CrossRef CAS.
- B. Chakraborty, R. Beltrán-Suito, V. Hlukhyy, J. Schmidt, P. W. Menezes and M. Driess, Crystalline Copper Selenide as a Reliable Non-Noble Electro(pre)catalyst for Overall Water Splitting, ChemSusChem, 2020, 13, 3222–3229 CrossRef CAS.
- S. Kumaravel, K. Karthick, P. Thiruvengetam, J. M. Johny, S. S. Sankar and S. Kundu, Tuning Cu Overvoltage for a Copper-Telluride System in Electrocatalytic Water Reduction and Feasible Feedstock Conversion: A New Approach, Inorg. Chem., 2020, 59, 11129–11141 CrossRef CAS.
- K. C. Majhi and M. Yadav, Transition metal chalcogenides based nanocomposites as efficient electrocatalyst for hydrogen evolution reaction over the entire pH range, Int. J. Hydrogen Energy, 2020, 46, 24219–24231 CrossRef.
- J. Tian, Q. Liu, N. Cheng, A. M. Asiri and X. Sun, Self-Supported Cu3P Nanowire Arrays as an Integrated High-Performance Three-Dimensional Cathode for Generating Hydrogen from Water, Angew. Chemie, 2014, 126, 9731–9735 CrossRef.
- S. Chu, W. Chen, G. Chen, J. Huang, R. Zhang, C. Song, X. Wang, C. Li and K. (Ken) Ostrikov, Holey Ni-Cu phosphide nanosheets as a highly efficient and stable electrocatalyst for hydrogen evolution, Appl. Catal., B, 2019, 243, 537–545 CrossRef CAS.
- A. Han, H. Zhang, R. Yuan, H. Ji and P. Du, Crystalline copper phosphide nanosheets as an efficient janus catalyst for overall water splitting, ACS Appl. Mater. Interfaces, 2017, 9, 2240–2248 CrossRef CAS.
- K. Karthick, S. Anantharaj, S. Patchaiammal, S. N. Jagadeesan, P. Kumar, S. R. Ede, D. K. Pattanayak and S. Kundu, Advanced Cu3Sn and Selenized Cu3Sn@Cu Foam as Electrocatalysts for Water Oxidation under Alkaline and Near-Neutral Conditions, Inorg. Chem., 2019, 58, 9490–9499 CrossRef CAS.
|
This journal is © the Partner Organisations 2021 |