DOI:
10.1039/C5RA03481G
(Paper)
RSC Adv., 2015,
5, 36368-36373
In situ polydopamine-assisted deposition of silver nanoparticles on a two dimensional support as an inexpensive and highly efficient SERS substrate†
Received
26th February 2015
, Accepted 13th April 2015
First published on 16th April 2015
Abstract
Fabrication of cheap and disposable substrates with high surface enhanced Raman scattering (SERS) effects is essential for practical SERS application. In this study, versatile substrates (silica plate, polyethylene film and filter paper) were simply modified with polydopamine followed by in situ silver nanoparticle deposition to fabricate a two-dimensional SERS substrate. This kind of SERS substrate has good reproducibility (relative standard deviation (RSD) <8% (spot-to-spot) and <12% (batch-to-batch)) and stability (>89% after 2 months). Using 4-mercaptopyridine (4-Mpy) as the probe molecule, the enhancement factor could reach 107. This facile and general approach can be easily scaled up to fabricate cheap, easily portable, flexible and disposable SERS substrates, which can be applied for on-site SERS detection.
1 Introduction
The surface-enhanced Raman scattering (SERS) technique has emerged as a powerful analytical tool due to its molecular specificity and high sensitivity.1–3 Noble metal nanoparticle suspensions are commonly used in SERS detection due to their ease of preparation and SERS activity, but they tend to agglomerate and even precipitate with time.4 Therefore, a strategy for the incorporation of noble metal nanoparticles on macroscopic substrates as SERS substrates has been developed using a wide range of methods such as lithography techniques,5,6 template-assisted fabrication,7–9 oblique angle deposition,10,11 Ar-ion sputtering12 and galvanic displacement reaction,13,14 etc. Nevertheless, most of those techniques are either complicated or expensive, which are hard to scale up to prepare large quantities of substrates for the practical applications.
The fabrication of stable, general and inexpensive SERS-active substrates in a simple way is essential for SERS application. Dopamine (DA), a biomolecule that contains catechol and amine functional groups,15 has received considerable attention in functional interface preparation due to its unique properties including self-polymerizability, reducibility, reactivity and biocompatibility.16 Especially, DA can self-polymerize into polydopamine (PDA) at alkaline environments and then spontaneously adhere on virtually any surface, which can be beneficial to a facile and efficient approach to modify versatile support surfaces.17,18 What's more, the reducibility of PDA facilitates the further in situ silver reduction.19,20 Our previous work also showed that the as-prepared AgNPs were directly deposited onto PDA surface to realize functionalization.21 Such kind of AgNPs/PDA composites have already been applied in anti-microbial surfaces,22,23 catalysis,24,25 sensor,26–28 as well as environmental fields.29,30 Akin et al.31 deposited AgNPs/PDA on 3-D silicon nanowire (SiNW) arrays to fabricate SERS substrate. A similar research was developed by Zhou et al., who electrochemically deposited flowerlike gold nanostructures on PDA coated indium tin oxide (ITO) for SERS application.32 However, their work relied on the expensive SiNW arrays or the electrochemical approach/conductive ITO film.
In this work, we proposed a low-cost and convenient protocol to prepare AgNPs/PDA substrates with high reproducibility and stability. The resultant AgNPs/PDA substrates were characterized by field emission-scanning electron microscope (FE-SEM), X-ray photoelectron spectroscopy (XPS) and UV-Vis spectroscopy. 4-Mercaptopyridine (4-Mpy) was chosen as probe molecule to study the SERS activity of the two dimension substrates through observing the influence of reduction time, reaction temperature and the concentration of AgNO3 during fabrication process. We also demonstrate the fabrication of AgNPs/PDA coated silica plate, polyethylene (PE) film and filter paper as potential cheap and disposable SERS substrates.
2 Experimental section
2.1. Materials
Tris(hydroxymethyl)aminomethane hydrochloride (Tris, 99%), dopamine hydrochloride (DA, 98%), 4-mercaptopyridine (4-Mpy, 96%) and tetramethylthiuramdisulfide (thiram, 97%) were purchased from Aladdin (Shanghai, China). Silver nitrate (AgNO3, >99%), rhodamine 6G (R6G, 99%) and trisodium citrate dehydrate (C6H5Na3O7·2H2O, ≥99%) were obtained from Sigma-Aldrich (USA). Ethanol absolute (ethanol, ≥99.7%) was purchased from Richjoint Chemical (Shanghai, China). All reagents were used as received without further purification. Milli-Q water (18.2 MΩ cm) was obtained using a Milli-Q system (Millipore). Silica plate (45 mm × 12.5 mm × 1 mm) and glassware were cleaned with aqua regia (HCl
:
HNO3 = 3
:
1) and then thoroughly rinsed with Milli-Q water.
2.2 Fabrication of SERS substrates
Substrates were immersed in DA solution (2 mg mL−1 in 10 mM Tris–HCl buffer, pH 8.0) for 2 h under mechanically stirring at room temperature. Polydopamine (PDA) thin films were formed through spontaneous polymerization of DA and then adhered on the substrates. Then, PDA-coated substrates were thoroughly rinsed with Milli-Q water and dried in the air. Subsequently, PDA-coated substrates were immersed into a 10 mg mL−1 AgNO3 solution in dark for 3 days at 50 °C. The sample, which was denoted as AgNPs/PDA, was thoroughly washed with Milli-Q water and dried for further use.
2.3 Raman Measurements
4-Mpy or thiram was dissolved in ethanol absolute to prepare 10−3 M ethanol solution and was then diluted with ethanol into various concentrations. R6G was dissolved in water to prepare 10−3 M aqueous solution and was then diluted with water into various concentrations. 10 μL of analyte ethanol (4-Mpy or thiram) or water (R6G) solution with different concentration was dropped onto the AgNPs/PDA substrate and allowed to dry before SERS measurement.
2.4 Measurements
UV-Vis spectra were collected using a UV-7504PC Spectroscopy (Shanghai Xinmao Instrument). The micrographs of samples were measured with field emission scanning electron microscopy (FE-SEM, Hitachi S-4800) equipped with an energy dispersive X-ray spectroscopy (EDX, Bruker AXS Microanalysis Gmbh). Surface topology was measured by Atomic force microscope (AFM, NanoScope IIIa/Dimension 3100). X-ray photoelectron spectroscopy (XPS, PHI 5000 Versa Probe) was performed to identify the elementary composition. SERS spectra were recorded using a Jobin Yvon confocal laser Raman system (SuperLabRam II), which was equipped with a He–Ne laser at 632.8 nm with a power of ca. 5 mW. Each spectrum was obtained by three accumulations, and the acquisition time in each case was 8 s.
3 Results and discussion
3.1. Fabrication of AgNPs/PDA substrates
A simple, two step approach was applied to fabricate SERS-active AgNPs/PDA substrate, as shown in Scheme 1. Firstly, DA self-polymerization was performed at ambient conditions to deposit PDA on virtually any substrate. Then AgNPs can be easily deposited on the preformed PDA layer by PDA reduction of silver ions.
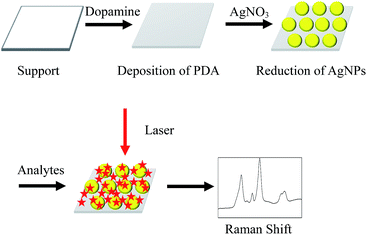 |
| Scheme 1 Schematic illustration of the fabrication of AgNPs/PDA substrate for SERS detection. | |
As a demonstration, AgNPs deposition onto the surface of PDA modified silica were performed to fabricate SERS substrate. Fig. 1A shows the SEM image of PDA-modified silica plate (PDA/silica), revealing no significant morphological change compared to the unmodified one (image not shown). Alternatively, AFM study explored that the PDA/silica substrate became rougher (root-mean-square roughness (RMS) = 3.20 nm) than the unmodified silica plate (RMS = 0.17 nm) (Fig. S1†). UV-Vis spectrum of the PDA/silica substrate (Fig. 1D(b)) showed a weak and monotonic absorption curve, which is similar with literature.33,34 XPS spectrum of PDA/silica substrate clearly revealed the presence of N 1s peak (Fig. 2A), including amines (–C–NH) at ∼400.3 eV, protonated N (C–NH3+) at ∼402.0 eV and aromatic N at ∼399.5 eV through deconvolution analysis (Fig. 2B),35,36 which confirms the successful modification of PDA.
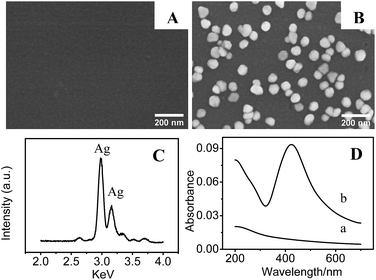 |
| Fig. 1 SEM images of (A) PDA and (B) AgNPs/PDA coated silica. (C) EDX spectrum of the AgNPs/PDA. (D) UV-Vis spectra of (a) PDA and (b) AgNPs/PDA coated silica. | |
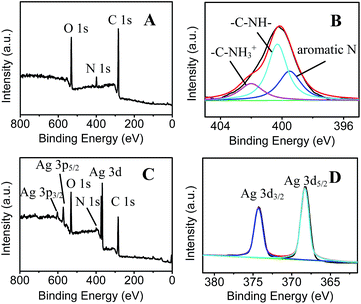 |
| Fig. 2 XPS spectra of (A) PDA and (C) AgNPs/PDA, (B) N 1s high-resolution spectra of PDA and (D) Ag 3d high-resolution spectra of AgNPs/PDA. | |
After immersing the PDA/silica in AgNO3 solution for several days, particles with average diameter less than 100 nm were observed on the surface (Fig. 1B). EDX analysis also depicted the existence of Ag element (Fig. 1C). Furthermore, UV-Vis spectrum presented a broad plasmonic resonance band centered at ∼425 nm (Fig. 1D(b)) from AgNPs. A strong Ag signal peak at 370.0 eV could be found in XPS analysis Fig. 2C and the high-resolution XPS spectra in Fig. 2D exhibits two peaks at 368.2 eV and 374.2 eV assigned to the binding energies of Ag 3d5/2 and Ag 3d3/2, respectively.37 All these results revealed that PDA modification followed by AgNO3 reduction is a facile technique to fabricate AgNPs/PDA/silica composite substrate.
4-Mpy was used as the probe molecule to evaluate the SERS activities of AgNPs/PDA/silica substrate. Interestingly, in Fig. 3a, Raman spectrum of AgNPs/PDA/silica substrate shows no signal, which is regarded as a clear SERS substrate for real sample detection. Compared with the normal Raman spectrum of solid 4-Mpy (Fig. 3b), in Fig. 3d, the relatively broader SERS peaks of 4-Mpy at 430, 708, 1010, 1062, 1096, 1220, 1477, 1583 and 1611 cm−1 are seen. At the same time, there are some shifts between SERS signals and normal Raman signals, and this may caused by chemical enhancement, in particular the increased polarizability.2 Moreover, it can also be seen from Fig. 3 that AgNPs/PDA/silica-based SERS peaks of 4-Mpy is similar to the result obtained from citrate capped Ag colloids (Fig. 3c) and the intensities is much greater than that of the Ag colloids,38 showing it is a good candidate for SERS analysis. In addition, a strong SERS band at 1096 cm−1 corresponding to the ring-breathing/C–S stretching modes indicated the adsorption of 4-Mpy on the AgNPs surface via the sulfur atom.39
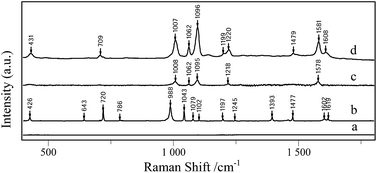 |
| Fig. 3 Raman spectra of (a) AgNPs/PDA substrate, (b) 4-Mpy solid, SERS spectrum of 10−6 M 4-Mpy detect with (c) citrate capped Ag colloids, (d) AgNPs/PDA substrate. | |
3.2. Optimization of AgNPs/PDA/silica substrate
3.2.1 Reaction time. The influence of Ag ion reduction time for AgNPs/PDA/silica on SERS effect was studied. Through observing from Fig. 4A–D, the size, density and the aggregation degree of AgNPs increase with increasing reaction time, but the average distance between AgNPs decreases.40 In the case of one day reaction, as shown in SEM result of Fig. 4A, only small AgNPs with sizes less than 10 nm can be seen at the surface. Accordingly, in Fig. 4E and F, such substrate has very low SERS effect. After reaction lasting for 2 days, the size of AgNPs becomes larger and diameters are ranging from 20 to 50 nm, as seen in Fig. 4B. The density of the AgNPs also increased. The SERS signal of AgNPs/PDA/silica made via 2 day selfdeposition of AgNPs significantly enhanced (Fig. 4E and F). It is well known that the interparticle junctions that are closed enough (less than 10 nm) will generate “hot spots” – highly localized regions of intense local field enhancement caused by surface plasmon resonances, leading to extraordinary SERS signals even to single molecular level.41 While the reaction time lasting 3 days, the size of AgNPs reached ∼60 nm and interparticle gaps became smaller (Fig. 4C), and the highest SERS signal of 4-Mpy recorded from AgNPs (60 nm)/PDA/silica in Fig. 4E hints the production of more hot spots. However, large clots and severe aggregations can be seen with increasing reduction time, perhaps due to Oswald ripening (Fig. 4D).42 This directly resulted in the poor SERS activity. Reduction time of 3 days as the optimal condition was picked out for the following experiment.
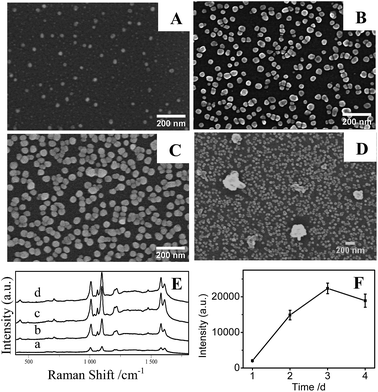 |
| Fig. 4 SEM images of AgNPs on silica prepared with different time (A) 1 day, (B) 2 days, (C) 3 days and (D) 4 days; (E) SERS spectra of 10−6 M 4-Mpy on AgNPs/PDA composite films prepared with different reduction time: (a) 1 day, (b) 2 days, (c) 3 days and (d) 4 days; and (F) the plot of the influence of reduction time on the SERS intensity at 1096 cm−1. | |
3.2.2 The concentration of AgNO3. The influence of AgNO3 concentration on the SERS activity of the substrate was also investigated. It can be clearly seen from Fig. 5 that AgNPs/PDA/silica substrate prepared with the concentration of AgNO3 at 10 mg mL−1 show a due density and distances at hot spots level of AgNPs for producing the best SERS effect. What's more, a weak extinction peak emerged around 650 nm (Fig. 5D(c)), which is believed to be from the strong coupling of closely-packed AgNPs.43,44 However, in the case of using AgNO3 solution with the concentration at 25 mg mL−1, the resultant AgNPs in the substrate were uneven, some are even fused together (pointed by arrow in Fig. 5D). This aggregation and fusing is confirmed by the increased and broadened plasmonic band in the range of 600–800 nm. The random aggregation of AgNPs diminished SERS intensity. So 10 mg mL−1 seems to be a reasonable AgNO3 concentration for preparing the SERS substrate.
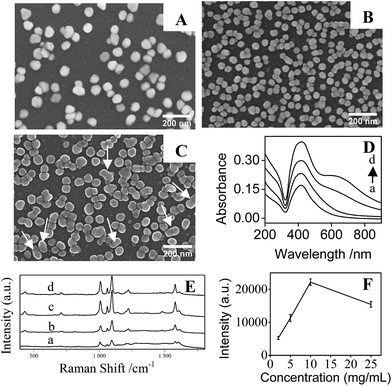 |
| Fig. 5 SEM images of AgNPs on silica prepared with different concentration of AgNO3: (A) 2 mg mL−1, (B) 10 mg mL−1 and (C) 25 mg mL−1; (D) UV-Vis spectra of AgNPs/PDA prepared with different concentration of AgNO3: (a) 2 mg mL−1, (b) 5 mg mL−1, (c) 10 mg mL−1, (d) 25 mg mL−1; (E) SERS spectra of 10−6 M 4-Mpy on AgNPs/PDA composite film prepared with different concentration of AgNO3; and (F) the plot of the influence of AgNO3 on the SERS intensity at 1096 cm−1. | |
In Fig. 6A and B, it is clear that the density of AgNPs on substrates prepared at temperature at 20 °C are lower than that at higher temperature at 50 °C, resulting in a weak LSPR absorption (Fig. 6D(a)) and a low SERS signal (Fig. 6E and F). Correspondingly, AgNPs/PDA/silica substrate made at 50 °C presents a homogeneous and densely-packed state of AgNPs in its SEM image of Fig. 6B and the UV-Vis spectrum in Fig. 6D(b) shows a broadened coupling LSPR band within 600–800 nm, confirming the formation of AgNPs hot-spots with the highest Further increasing temperature up to 80 °C cause the severe aggregation of AgNPs (Fig. 6C), the corresponding UV-Vis spectrum (Fig. 6D(c)) also presents a broad LSPR band within 600–800 nm. These results prove that the increase of temperature can accelerate the growth and fusion of AgNPs at the same time.45 As a consequence, it should pay attention on setting a suitable reaction temperature (herein, 50 °C) for controllably preparing AgNPs/PDA/silica substrate with more hot-spots to generate high SERS intensity (Fig. 6E and F).
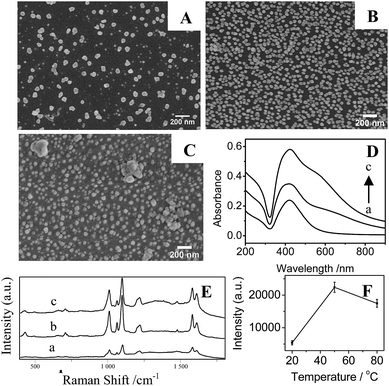 |
| Fig. 6 SEM images of AgNPs on silica prepared by (A) 20 °C, (B) 50 °C and (C) 80 °C, (D) UV-Vis spectra of AgNPs/PDA prepared at different temperatures: (a) 20 °C, (b) 50 °C and (c) 80 °C; (E) SERS spectra of 10−6 M 4-Mpy on AgNPs/PDA composite film prepared with different temperatures: (a) 20 °C, (b) 50 °C and (c) 80 °C; and (F) the plot of the influence of temperature on the SERS intensity at 1096 cm−1. | |
3.3. Examination of SERS performance
Fig. 7A shows that the concentration dependent SERS spectra of 4-Mpy using the optimized AgNPs/PDA/silica as SERS substrate.
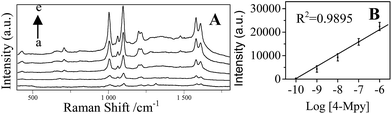 |
| Fig. 7 (A) SERS spectra of 4-Mpy with various concentration (a) 10−10 M, (b) 10−9 M, (c) 10−8 M, (d) 10−7 M and (e) 10−6 M on AgNPs/PDA substrates; (B) the linear relationship between the Raman intensity at 1096 cm−1 and 4-Mpy concentration. | |
As shown in Fig. 7B, a good linear between the Raman intensity at 1096 cm−1 and the logarithm of 4-Mpy concentration is exhibited in the range of 0.1–1000 nM. The minimum detectable concentration was 0.1 nM. The enhancement factor (EF) was calculated according to the Raman intensity at 1096 cm−1 using the following formula:46,47
where
ISERS and
Ibulk are the Raman intensities for the SERS and the normal Raman, respectively.
Nbulk and
NSERS are the number of molecules under laser illumination for bulk sample, and the number of molecules in the substrates, respectively. The EF of optimized AgNPs/PDA/silica substrate could reach 10
7. To evaluate the reproducibility, the SERS spectra of 10
−6 M 4-Mpy are collected from 50 randomly selected sites on the surface of the optimized AgNPs/PDA/silica substrate. Raman spectra with high reproducibility were determined as shown in
Fig. 8A. The average intensities of the two peaks at 1007 cm
−1 (
Fig. 8B) and 1096 cm
−1 (
Fig. 8C) were calculated to be 16
![[thin space (1/6-em)]](https://www.rsc.org/images/entities/char_2009.gif)
443.9 ± 1209.8 (relative standard deviation, RSD = 7.85%) and 22
![[thin space (1/6-em)]](https://www.rsc.org/images/entities/char_2009.gif)
914.6 ± 1489.4 (RSD = 6.50%), respectively. It indicated an excellent SERS reproducibility of AgNPs/PDA/silica substrate. SERS signals (Fig. S2
†) of 4-Mpy recorded from 10 different batches of AgNPs/PDA/Silica demonstrated a low RSD (12.09%).
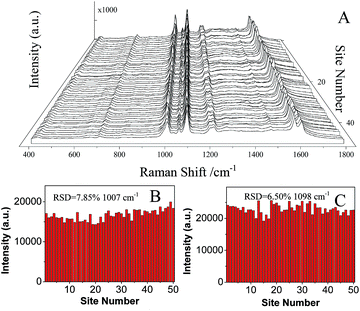 |
| Fig. 8 (A) SERS spectra of 10−6 M 4-Mpy collected on 50 randomly selected spots on AgNPs/PDA substrates. (B and C) the corresponding intensities of the main Raman peaks (B: 1007 cm−1, C: 1096 cm−1). | |
The stability of the AgNPs/PDA/silica substrate was investigated by taking SERS measurement over 2 months period. As shown in Fig. 9A, the major Raman peaks show no obvious shift. The Raman intensity slightly decreased (Fig. 9B) after storage for 2 months at ambient condition but still retained 89%. As two model applications, SERS detections of a pesticide named thiram (Fig. 10) and an organic dye named R6G (Fig. S3†) were conducted after directly dropping them onto the surface of AgNPs/PDA/silica substrate. The limit of detection (LOD) for thiram was as low as 10−7 while LOD for R6G was 10−9.
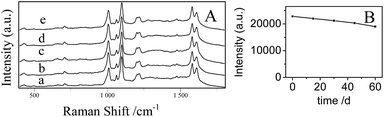 |
| Fig. 9 (A) SERS spectra of 10−6 M 4-Mpy on the AgNPs/PDA substrates with different storage time (a) 0 day, (b) 15 days, (c) 30 days, (d) 45 days and (e) 60 days. (B) SERS intensity of the band of 4-Mpy at 1096 cm−1 with different storage time. | |
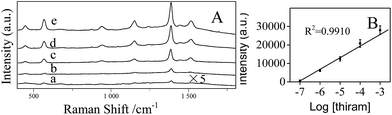 |
| Fig. 10 (A) SERS spectra of thiram with various concentration (a) 10−7 M, (b) 10−6 M, (c) 10−5 M, (d) 10−4 M, (e) 10−3 M on AgNPs/PDA substrates. (B) The linear relationship of Raman intensity at 1373 cm−1 with the thiram concentration. | |
Considering the extensive application using lower cost SERS substrates, polyethylene (PE) films and filter papers as the possible supports were also investigated for making AgNPs/PDA-based SERS substrates. The fabrication processes are the same as AgNPs/PDA/silica. It should be pointing out that normally the modification of some inert materials such as PE can only be achieved in harsh conditions or by expensive equipments, for example, plasma facility. Alternatively, PDA modification followed by AgNPs deposition provides a simple and mild approach to fabricate AgNPs/PDA/PE SERS substrate. Both AgNPs/PDA/PE and AgNPs/PDA/filter substrate exhibited the strong SERS effect (Fig. S4†), which could be used as the promising SERS substrates for real applications.
4. Conclusions
In summary, a facile approach was applied to prepare AgNPs/PDA/silica SERS substrates with Raman enhancement factor of 107 for detecting 4-Mpy. This kind of SERS substrate has good reproducibility (relative standard deviation (RSD) <8% (spot-to-spot) and <12% (batch-to-batch)) and stability (>89% after 2 month). This approach can also be applied to make flexible substrates using PE film and filter paper as the supports. Our results showed that PDA played a key role for developing an easy, general and low cost strategy for the large-scale fabrication of disposable SERS substrates on versatile substrates.
Acknowledgements
The authors are grateful for the financial support provided by Shanghai Municipal Education Commission (Grant no. 13ZZ101) and Science and Technology Commission of Shanghai Municipality (Grant no. 14ZR1430200).
Notes and references
- K. A. Willets, Chem. Soc. Rev., 2014, 43, 3854 RSC
. - S. Schlücker, Angew. Chem., Int. Ed., 2014, 53, 4756 CrossRef PubMed
. - P. L. Stiles, J. A. Dieringer, N. C. Shah and P. V. Duyne, Annu. Rev. Anal. Chem., 2008, 1, 601 CrossRef CAS PubMed
. - Z. Deng, X. X. Chen, Y. R. Wang, E. H. Fang, Z. G. Zhang and X. Chen, Anal. Chem., 2015, 87, 633 CrossRef CAS PubMed
. - S. S. Shankar, L. Rizzello, R. Cingolani, R. Rinaldi and P. P. Pompaet, ACS Nano, 2009, 3, 893 CrossRef CAS PubMed
. - H. Y. Fu, X. Y. Lang, C. Hou, Z. Wen, Y. F. Zhu, M. Zhao, J. C. Li, W. T. Zheng, Y. B. Liu and Q. J. Fu, J. Mater. Chem. C, 2014, 2, 7216 RSC
. - M. A. Khan, T. P. Hogan and B. Shanker, J. Raman Spectrosc., 2009, 40, 1539 CrossRef CAS PubMed
. - J. Hu and C. Y. Zhang, Anal. Chem., 2010, 82, 8991 CrossRef CAS PubMed
. - Y. Ou, L. W. Zhu, W. D. Xiao, H. C. Yang, Q. J. Jiang, X. Li, J. G. Lu, L. S. Wan and Z. K. Xu, J. Phys. Chem. C, 2014, 118, 4403 CAS
. - J. D. Driskell, S. Shanmukh, Y. J. Liu, S. B. Chaney, X. J. Tang, Y. P. Zhao and R. A. Dluhy, J. Phys. Chem. C, 2008, 112, 895 CAS
. - J. L. Abell, J. D. Drikell, R. A. Dluhy, R. A. Tripp and Y. P. Zhao, Biosens. Bioelectron., 2009, 24, 3663 CrossRef CAS PubMed
. - Y. Yang, Z. Y. Li, K. Yamaguchi, M. Tanemura, Z. R. Huang, D. L. Jiang, Y. H. Chen, F. Zhou and M. Nogami, Nanoscale, 2012, 4, 2663 RSC
. - Q. X. Zhang, Y. X. Chen, Z. Guo, H. L. Liu, D. P. Wang and X. J. Huang, ACS Appl. Mater. Interfaces, 2013, 5, 10633 CAS
. - A. Gutes, C. Carraro and R. Maboudian, ACS Appl. Mater. Interfaces, 2009, 1, 2551 CAS
. - H. Lee, S. M. Dellatore, W. M. Miller and P. B. Messersmith, Science, 2007, 318, 426 CrossRef CAS PubMed
. - Y. L. Liu, K. L. Ai and L. H. Lu, Chem. Rev., 2014, 114, 5057 CrossRef CAS PubMed
. - R. A. Zangmeister, T. A. Morris and M. J. Tarlov, Langmuir, 2013, 29, 8619 CrossRef CAS PubMed
. - S. Hong, Y. S. Na, S. Choi, I. T. Song, W. Y. Kim and H. Lee, Adv. Funct. Mater., 2012, 22, 4711 CrossRef CAS PubMed
. - J. Yan, L. P. Yang, M. F. Lin, J. Ma, X. H. Lu and P. S. Lee, Small, 2013, 9, 596 CrossRef CAS PubMed
. - H. L. Xu, X. K. Liu, G. Su, B. Zhang and D. Y. Wang, Langmuir, 2012, 28, 13060 CrossRef CAS PubMed
. - F. Wang, R. Han, G. T. Liu, H. F. Chen, T. R. Ren, H. F. Yang and Y. Wen, J. Electroanal. Chem., 2013, 706, 102 CrossRef CAS PubMed
. - T. S. Sileika, H. D. Kim, P. Maniak and P. B. Messermith, ACS Appl. Mater. Interfaces, 2011, 3, 4602 CAS
. - Z. Zhang, J. Zhang, B. L. Zhang and J. L. Tang, Nanoscale, 2013, 5, 118 RSC
. - J. J. zhou, B. Duan, Z. Fang, J. B. Song, C. X. Wang, P. B. Messersmith and H. W. Duan, Adv. Mater., 2014, 26, 701–705 CrossRef CAS PubMed
. - B. Çelen, D. Ekiz, E. Piskin and G. Demirel, J. Mol. Catal. A: Chem., 2011, 350, 97 CrossRef PubMed
. - Y. Ma, H. Y. Niu, X. L. Zhang and Y. Q. Cai, Analyst, 2011, 136, 4192 RSC
. - X. C. Sun, S. Stagon, H. C. Huang, J. Chen and Y. Lei, RSC Adv., 2014, 4, 23382 RSC
. - K. Jia, M. Y. Khaywah, Y. G. Li, J. L. Bijeon, P. M. Adam, R. Déturche, B. Guelorget, M. Francois, G. Louarn and E. Ionescu, ACS Appl. Mater. Interfaces, 2014, 6, 219 CAS
. - H. Y. Niu, S. H. Wang, T. Zeng, Y. X. Wang, X. L. Zhang, Z. F. Meng and Y. Q. Cai, J. Mater. Chem., 2012, 22, 15644 RSC
. - S. X. Zhang, Y. Y. Zhang, G. M. Bi, J. S. Liu, Z. G. Wang, Q. Xu, H. Xu and X. Y. Li, J. Hazard. Mater., 2014, 270, 27 CrossRef CAS PubMed
. - M. S. Akin, M. Yilmaz, E. Babur, B. Ozdemir, H. Erdogan, U. Tamer and G. Demirel, J. Mater. Chem. B, 2014, 2, 4894 RSC
. - W. C. Ye, D. A. Wang, H. Zhang, F. Zhou and W. M. Liu, Electrochim. Acta, 2010, 55, 2004 CrossRef CAS PubMed
. - V. Ball, I. Nguyen, M. Haupt, C. Oehr, C. Arnoult, V. Toniazzo and D. Ruch, J. Colloid Interface Sci., 2011, 364, 359 CrossRef CAS PubMed
. - F. Bernsmann, A. Ponche, C. Ringwald, J. Hemmerlé, J. Raya, B. Bechinger, J. Voegel, P. Schaaf and V. Ball, J. Phys. Chem. C, 2009, 113, 8234 CAS
. - R. A. Zangmeister, T. A. Morris and M. J. Tarlov, Langmuir, 2013, 29, 8619 CrossRef CAS PubMed
. - Y. H. Ding, L. T. Weng, M. Yang, Z. L. Yang, X. Lu, N. Huang and Y. Leng, Langmuir, 2014, 30, 12258 CrossRef CAS PubMed
. - Y. Cong, T. Xia, M. Zou, Z. N. Li, B. Peng, D. Z. Guo and Z. W. Deng, J. Mater. Chem. B, 2014, 2, 3450 RSC
. - Y. W. Chao, Q. Zhou, Y. Li, Y. R. Yan, Y. Wu and J. W. Zheng, J. Phys. Chem. C, 2007, 111, 16995 Search PubMed
. - J. W. Hu, B. Zhao, W. Q. Xu, B. F. Li and Y. G. Fan, Spectrochim. Acta, Part A, 2002, 58, 2827 CrossRef
. - P. Rajapandiyan and J. Yang, J. Raman Spectrosc., 2014, 45, 574 CrossRef CAS PubMed
. - A. Shiohara, Y. S. Wang and L. M. Marzán, J. Photochem. Photobiol., C, 2014, 21, 2 CrossRef CAS PubMed
. - W. Y. Yang, F. Gao, G. D. Wei and L. An, Cryst. Growth Des., 2010, 10, 29 CAS
. - P. J. G. Goulet, D. S. Santos Jr, R. A. Alvarez-Puebla, O. N. Oliverira and R. F. Aroca, Langmuir, 2005, 21, 5576 CrossRef CAS PubMed
. - A. J. Wang, J. J. Lv, D. L. Zhou, X. X. Weng, S. F. A. Qin and J. J. Feng, New J. Chem., 2014, 38, 3395 RSC
. - Y. Q. Lin, L. B. Li, L. L. Hu, K. Y. Liu and Y. N. Xu, Sens. Actuators, B, 2014, 202, 527 CrossRef CAS PubMed
. - E. C. Le Ru, E. Blackie, M. Meyer and P. G. Etchegoin, J. Phys. Chem. C, 2007, 111, 13974 Search PubMed
. - X. M. Lin, Y. Cui, Y. H. Xu and B. Ren, Anal. Bioanal. Chem., 2009, 394, 1729 CrossRef CAS PubMed
.
Footnote |
† Electronic supplementary information (ESI) available: AFM images, batch-to-batch reproducibility, SERS spectra of R6G, SERS spectra collected on AgNPs/PDA/PE and AgNPs/PDA/filter paper. See DOI: 10.1039/c5ra03481g |
|
This journal is © The Royal Society of Chemistry 2015 |