DOI:
10.1039/D4SD00262H
(Critical Review)
Sens. Diagn., 2025,
4, 7-23
Dengue-virosensor: advancement of dengue virus-based biosensors
Received
23rd July 2024
, Accepted 22nd October 2024
First published on 22nd November 2024
Abstract
Dengue is one of the world's fastest-growing health issues, affecting primarily tropical and sub-tropical countries. Dengue infection is spread via mosquitoes, i.e., Aedes, and caused by the dengue virus (DENV), a single-stranded RNA virus having 4 serotypes, i.e., DENV 1–4, and any one of the 4-serotypes can cause dengue fever. Dengue symptoms can range from asymptomatic primary infection to fatal secondary infections, such as dengue shock syndrome (DSS) and dengue hemorrhagic fever (DHF). Since there are no dengue vaccinations or antiviral treatments available, total bed rest, proper hydration, and the use of analgesics for symptomatic pain relief are usually prescribed, which make recovery both difficult and time-consuming. This mandates the development of early detection methods for DENV so that the disease can be stopped before it spreads. For example, traditional laboratory-based diagnostic approaches for detecting dengue infection have one or more flaws. This includes cross-reactivity with other flaviviruses, the need for several samples for serological assays, and the high cost and complexity of PCR processes. Thus, biosensors have garnered considerably greater attention because of their simple fabrication, ease of use, ultra-sensitivity, selectivity, and low cost of production. This review starts with an introduction and a discussion on the conventional methods used for dengue virus detection, along with their limitations. Later, recently developed and futuristic biosensors are summarized before and finally closing this review with a brief conclusion.
1. Introduction
The dengue RNA virus of the Flaviviridae family, a member of the genus Flavivirus, causes severe infection, i.e. dengue fever, which is spread through Aedes aegypti and Aedes albopictus.1Aedes aegypti is predominately present in tropical and sub-tropical areas of the world2 and is found in large numbers in these locations during the rainy season. As a result, the disease is more prevalent during the rainy season.3Aedes aegypti is a domestic species that is especially sensitive to dengue virus infection and feeds on human blood primarily during the day. It is also well acclimated to civilized regions since it breeds in clean stagnant water in artificial containers.4 Various other severe diseases caused by viruses such as chikungunya (CHIK), Zika (ZIK), and yellow fever (YF) are also spread through Aedes aegypti. Among them, dengue viruses cause the dengue fever, which is also called break-bone fever, water poison or cramp-like seizures.5 About 50–100 million dengue fever cases are reported every year in 100 nations, according to WHO estimations.6 From 2014 to 2015, the number of dengue-infected persons in India had increased, with Delhi being hit the hardest with 1800 cases, all of these statistics raise concerns.7 India was once again added to the list of dengue-affected countries in 2020, with a similar spike in cases in Sudan, Yemen, Bangladesh, Brazil, Cook Islands, Timor-Leste, Ecuador, Mauritania, Singapore, Indonesia, Maldives, Mayotte (Fr), Nepal, Sri Lanka, and Thailand. In 2021, dengue fever was highlighted as a serious issue in Colombia, Reunion Island, Paraguay, Brazil, the Cook Islands, Kenya, Peru, and Fiji.8 DENV-1, -2, -3, and -4 are closely related to each other but are different genetically, isolating them as different DENV serotypes.9 The nucleotide sequences of these 4-dengue virus-serotypes vary by 25–35 base pairs, and each serotype can cause dengue fever. Among all four serotypes of dengue, DENV serotype 4 is the most distinct, followed by DENV serotype 3, while DENV serotypes 1 and 2 are more closely related to each other. All serotype infections give the serotype long-term immunity but have limited transitional immunity to the other three. Secondary infection with various serotypes has been linked to more severe dengue, as per epidemiological studies.10 The physiology of dengue virus involves the 3 structural proteins, C, prM, and E, which co-translate and post-translate to form the full infectious virus particle, also known as a virion. To construct the nucleocapsid, the C (capsid) protein surrounds the viral genomic RNA. This nucleocapsid is encased in a lipid bilayer that contains the viral pre-membrane protein, also called the prM protein and envelope protein, i.e., the E-protein. The 7 nonstructural proteins (NS1/NS2A/NS2B/NS3/NS4A/NS4B/NS5) are expressed in infected cells and are necessary for viral replication, virion assembly, and immune evasion. Nonstructural proteins are typically present in the cytoplasm, where they provide replication products that contribute to the generation of viral RNA. Dengue virus NS1, on the other hand, is formed as a hydrophilic membrane linked homodimer in the endoplasmic reticulum. Because a mutation in the NS1 protein influences RNA production, studying the three-dimensional (3D) structures of the NS1 protein and the viral NS1–NS2A protein catalytic domain can aid in understanding the NS1 subunit's shape and involvement in viral pathogenesis. NS2B acts as a chaperone, aiding the NS3 component in folding into its active shape. It also participates in substrate–enzyme interactions as well as membrane attachment. Dengue virus NS3 and NS5 nonstructural proteins have several roles and are involved in enzymatic activity. NS4 is divided into two subunits: NS4A and NS4B. NS4A modulates intracellular membranes, and its C-terminus assists in NS4B subunit translocation. However, the function of the NS4B subunit is unknown, although a recent investigation found it may operate as an interferon competitor.11 When the dengue virus (DENV) enters the host, the immune system initiates an innate response that restricts viral multiplication. B-cells are then stimulated and generate antibodies. In an initial infection, IgM antibodies arise first to neutralize the virus, followed by IgG antibodies that offer long-term protection. In a subsequent infection, IgG antibodies from the prior exposure respond fast, but they may occasionally worsen the condition via antibody-dependent enhancement (ADE), potentially leading to more severe disease. IgM denotes primary infection, whereas IgG shows a previous or secondary infection.12 Dengue virus can produce mild to severe effects in humans, ranging from fever with some pathology manifestations to severe cases (DSS/DHF).13 A dengue-based infection is diagrammatically represented in Fig. 1. There are three different pathologies associated with dengue fever: Dengue fever (DF), which leads to severe involuntary shaking, which is manifested by joint pain and head/nausea/fever; DSS, which is characterized by acute plasma leakage, and DHF is accompanied by high-grade fever, hepatomegaly, and a hemorrhagic phenomenon. Secondary infection from specific subtypes increases the risk of DHF and DSS.14 Increased urbanization, population expansion, migration, and international travel, as well as the challenges of efficient vector management, are thought to be contributing to the rise in DF, while climate change also is potentially playing a role in the global transmission of DF.15 Dengue illness is a major public health issue in numerous areas. Presently, there is no effective medicine16 or effective vaccine licensed to fight against dengue.17 Sanofi Pasteur's Dengvaxia, i.e., CYD-TDV, is the only licensed vaccine for dengue, but it is not very effective at controlling the number of dengue cases.18 An appropriate diagnosis can play a promising role in dengue treatment. As a result, there are a range of conventional techniques accessible for dengue diagnosis, such as PCR, ELISA, virus isolation, IgM detection, and many more, but these traditional approaches each have various limitations, such as PCR being very expensive and requiring trained personnel, ELISA having very low specificity, virus isolation taking a long time to yield results, and IgM testing requiring two or more samples, as well as having a high possibility of cross-contamination. To address such restrictions, a new approach involving biosensing is emerging, which offers numerous benefits, like- early detection, cheap cost, ease in handling, no need for a trained expert, highly stable, on-site detection, high specificity, and high sensitivity. Table 1 summarizes the advantages and disadvantages of different dengue-based diagnostic methods. The WHO emphasizes that diagnostic tools, including biosensors, must meet the “ASSURED” (Affordable, Sensitive, Specific, User-friendly, Rapid and robust, Equipment-free, and Deliverable to end-users) criteria. These criteria can ensure that diagnostics are effective and accessible, predominantly in low-resource settings. Biosensor methods can enhance dengue confirmation by enabling the rapid, sensitive, and specific detection of dengue virus antigens (like NS1) or viral RNA.19 Several researchers, including Parkash et al., Chen et al., and Hasan et al., have demonstrated that utilizing a biosensor can efficiently detect the virus for the early diagnosis of dengue antigens and antibodies.20–22 They can enable faster diagnosis compared to traditional methods, such as RT-PCR, with potential for point-of-care use, especially in resource-limited regions. Biosensors can also improve the sensitivity and specificity, reduce false results, and can be designed for the multiplex detection of multiple viral markers. In alignment with the WHO's ASSURED criteria, biosensors can be affordable, portable, and robust enough to function without complex equipment, making them a valuable tool for supporting or complementing existing dengue detection techniques, as recommended by WHO guidelines for effective disease management.23 Biosensors also come in a wide variety of types, such as electrochemical biosensors, optical biosensors, LFA, colorimetric, and smartphone-based biosensors. All the mentioned biosensors could detect dengue virus antigen with its nonstructural protein NS-1 and other nonstructural proteins, such as NS2a, NS2b, NS3, NS4a, NS4b, and NS5. Various literature published on dengue diagnosis, such as Omar and their co-workers24 presented the SPR-based diagnosis approach for the E-protein of DENV. In this work, they discussed the SPR-based approach and its applications in dengue diagnosis, while Deng et al. created an impedimetric-based biosensor for dengue diagnosis, and to make it more sensitive they used a nanoporous alumina membrane.25 Kavitha et al.26 summarized the different nanocomposite structures to construct
effective electrochemical biosensors for the highly effective analysis of various pathogens. Recently in 2023, Thergarajan et al.27 systematically presented a review on diagnostic approaches for dengue infection, where they effectively discussed the conventional methods and highlighted biosensors based on the dengue virus. The present work is one of the first reviews on dengue diagnosis covering all the possible diagnosis approaches and that present novel and futuristic biosensors, such as robotic biosensors, lab-on-a-drone, and internet-based biosensors, for dengue diagnosis, and much more attractively presented in a simplified manner. So, in this review, we discussed newly developed biosensors for detecting the dengue virus, which is important in dengue treatment.
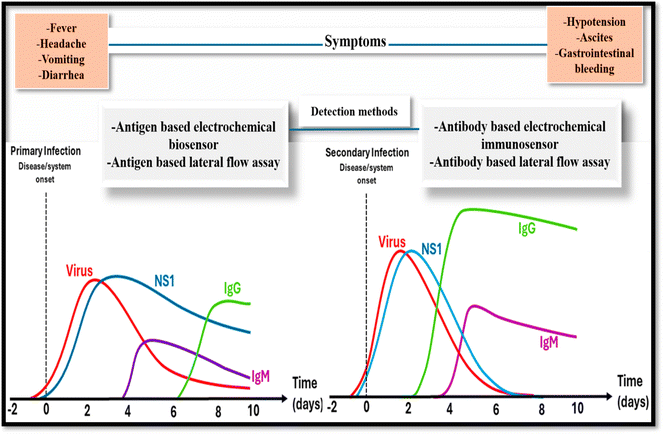 |
| Fig. 1 Diagrammatic representation showing the different phases of dengue virus-based infections, including, primary and secondary infections, along with the dengue symptoms and their detection methods. | |
Table 1 Advantages and disadvantages of various dengue based diagnostic methods
S. no |
Diagnostic method |
Advantages |
Limitations |
Ref. |
1 |
Hemagglutination-inhibition test (HI-test) |
-This method is a simple, fast, and sensitive test, especially for the dengue virus as it can easily detect the antibodies, i.e., less than 1 mg per ml |
-Poor specificity, requires sample in pairs and cannot differentiate among infecting-serotypes (dengue virus serotypes 1/2/3/4) |
28, 29 |
-No additional equipment is required and the reagents can be easily prepared |
-Necessity for chemical pretreatment to eliminate nonspecific inhibitors of haemagglutination and absorption with red blood cells to eliminate nonspecific agglutinins |
2 |
PCR |
-One of the most popular tests |
-Requires expensive instruments, reagents, and expertise to operate |
30
|
-DNA can be amplified to produce cDNA from a target RNA via a reverse transcription reaction process |
-False-negative outcomes possible due to variations of the serotypes |
-Globally, PCR has been widely employed for the sensitive, fast, and accurate diagnosis of DENV |
3 |
ELISA |
-Useful test for routine dengue diagnosis |
Time-consuming and costly |
31
|
-Helpful for anti-dengue IgM and IgG detection |
-Requires training to perform the assay |
4 |
Virus isolation |
One of the most sensitive and reliable approaches to confirm the dengue virus infection, specifically when serum samples are collected before the fever subsides |
-Unable to determine among primary and secondary infections, costly |
32
|
5 |
PRNT |
-Gold standard is to determine the serologic test outcomes of flaviviruses |
-Requires live virus |
33
|
-It also helps in the identification of false/positive outcomes of IgM |
-Expensive instruments needed, such as BSL-3 |
6. |
Biosensors |
-Extremely sensitive |
-Low stability and reproducibility |
34
|
-Speedy |
-Low capability to cope with complex clinical samples |
-Easy to control |
-Poor sensitivity in nonselective binding |
-Inexpensive |
-Costly sensor fabrication procedure |
-Label-free |
-Difficult pretreatment stages |
-Low volume of sample required |
-Sophisticated apparatus |
-On-site detection |
-Depends on sample preparation |
-Less invasive |
2. Biosensors
The word “biosensor” denotes an innovative and powerful analytical gadget that integrates a bio-sensing component and has an extensive uses. Clark and Lyons developed the 1st biosensor in 1962 to determine glucose in biological samples. Since that day, remarkable advances have been achieved in biosensor applications and technology involving novel strategies, such as electro-chemistry/nanotechnology, and bio-electronics.35 Biosensors are practical tools specifically designed to diagnose the biological analytes via transforming a biological item (for example, protein, DNA, or RNA) into an electrical response that is easily diagnosed and evaluated. A biosensor consists of three parts: a recognition component, a signal transducer, and a response amplifier that transmits and shows the outcomes. The recognition element identifies a signal in the form of an analyte in the environment, later the transducer changes the signal to a measured electric output.36 The critical points of biosensors – simple to use: many biosensors are designed in such a manner that they do not require any complex equipment and are simple to use;37technical advantages: biosensors provide one-step detection, which means they can offer data for single use rather than numerous times; fast analysis: it provides data in minutes, allowing quicker and more accurate identification.38
Multiple biosensors categories are designed to detect dengue virus, such as electrochemical, SPR- optical, LFA, colorimetric, and mobile-based biosensors. These different types of dengue biosensors are conferred below and these different biosensors are summarized in Table 3.
2.1. Classical electrochemical biosensors
These represent a type of sensor having an electrochemical transducer and chemically modified electrode, which is electrically conducting, semiconducting, or ionic conducting substances treated through a biological layer called a chemically modified electrode (CME) (Fig. 2). That is why these are also referred to as a self-contained integrated devices.39 Such types of biosensors have several specific benefits, including they can be specially prepared to satisfy the demands for compact size, inexpensive, require less volume and power criteria of dispersed testing. They have tremendous potential for a variety of environmental and biological science applications.40 Many authors have designed traditional electrochemical biosensors to detect DENV, such as Siew et al.,41 constructed an impedimetric immunosensor by employing graphene/titanium dioxide nanocomposites to modify the screen-printed electrode, as these nanocomposites increased the electrochemical performance of electrodes and also provided a specific surface area. A unique probe, i.e., DENV-EDIII protein, which is a plant-derivative and effectively detects dengue virus-IgG, showing a linearity of 62.5 to 200 ng ml−1 with a lower limit i.e. 2.81 ng ml−1, and also displayed an excellent selectivity to discriminate the dengue virus IgG from Zika virus. The reliability of the obtained outcomes of the electrochemical–immunosensor platform was successfully validated against the serum of mice and compared to ELISA. Solanki et al.42 demonstrated an immunosensor to detect NS1 protein by employing reduced graphene oxide nanosheet-based electrodes. In this, they used Hummer's technique to synthesize graphene oxide and then deposited it on the glass substrates that were coated with indium tin oxide with the help of Langmuir–Blodgett deposition. In this study, the spiked-sera samples and standard samples were successfully used to explore the performance of the constructed bio-electrodes to detect antigens of NS1 with the LOD of 0.069 and 0.081 ng ml−1, individually, and both samples also showed a remarkable sensitivity, i.e., 8.41 and 36.75 Ω per ng ml, correspondingly along with the detection range, i.e. 101–107 ng ml−1. Lee et al. coupled electrochemical biosensor with the emerging effective CRISPR technique.43 In this work, they electrochemically detected the dengue fever viral RNA by constructing a new reaction called CRISPR/Cpf1. The CRISPR/CRISPR from the Prevotella and Francisella-1 (CRISPR/Cpf1) system, which involved the CRISPR RNA (crRNA) and Cpf1 enzymatic protein, identifies a target sequence and randomly cut a nonspecific ssDNA with targeted DNA, and dengue virus RNA could also be quantified through utilizing this biological process by adding methylene blue and conjugate with functionalized Au-NPs, which is used to improve the electrochemical response in a highly selective mode. The established electrochemical biosensor demonstrated an ultrasensitive diagnosis of DENV-4 at concentrations as low as 100 fM without the need for any RNA amplification process. A dengue case study was recently conceptualized by Piedimonte et al.,44 by developing a differential impedance sensing system for the serological diagnosis of viruses depending on the impedance difference between Au microelectrodes after target antibodies were captured and amplify the signal via hybridized with nano-beads. The platform of the microfluidics core has a Differential Impedance Sensing (DIS) design, enabling comparison between a reference and an active sensor. This setup is capable of achieving NP precision in the range of few tens. The sensor, which was modified using a co-poly layer containing a synthetic peptide-probe, demonstrated a LOD of less than 100 pg ml−1 when tested with an antibody (Ig-G) model spiked in a buffer, which validated with human serum (positive) to anti-DENV-antibody. These are the reports on traditional pattern-based electrochemical biosensors that effectively detects dengue virus with high specificity.
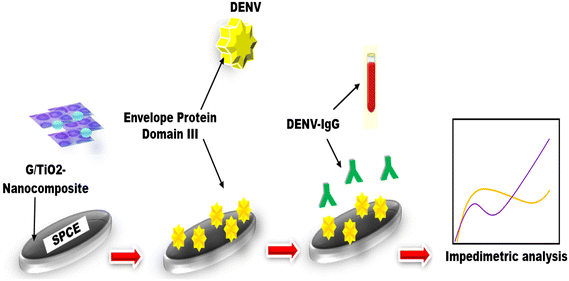 |
| Fig. 2 Diagrammatic representation of a dengue virus-based impedimetric immunosensor. | |
2.1.1 Different electrode setups of electrochemical biosensors for dengue virus determination.
Electrodes are the chief and essential modules of electrochemical biosensors on which the process of detection takes place, and they come in a large variety of setups, such as bulky electrode, screen-printed electrode, paper electrode, pencil electrode, and two- and three-electrode setups (Table 2).
Table 2 Comparison of different electrode setups of electrochemical dengue biosensors and their different features, such as cost, usage, setup, response, LOD, and biomarkers45,46
S. no |
Criteria |
Bulky electrode setup |
Screen-printed electrode setup |
Paper-electrode setup |
Two-electrode setup |
Three-electrode setup |
1. |
Availability |
Commercial |
Commercial |
In-house fabrication |
In-house fabrication/commercial |
In-house fabrication/commercial |
2. |
Usage |
Re-usable |
Re-usable |
Disposable |
Disposable/re-usable |
Disposable/re-usable |
3. |
Substrate |
Glass |
Plastic |
Paper/plastic |
Paper/plastic |
Paper/plastic |
4. |
Volume/sample |
50–60 μL |
15–25 μL |
20–30 μL |
20–30 μL |
20–30 μL |
5. |
Price/set (INR) |
100 000 |
1500 |
3–5 |
3–5 |
3–5 |
6. |
Setup |
3-electrode bulky setup |
3-electrode setup |
3-electrode setup |
2-electrode setup |
3-electrode setup |
-1. WE (gold, pencil, etc.) |
-2. CE (graphene) |
-3. RE (silver) |
7. |
Response |
Decent |
Highly accurate |
Excellent |
Very weak |
Very good |
8. |
Biomarker |
NS1 |
Dengue virus antigen (DENV-Ag) |
Dengue virus antigen (DENV-Ag) |
Dengue |
Dengue |
9. |
LOD |
22 pg ml−1 |
0.1 μg ml−1 |
0.1 μg ml−1 |
0.1 μg ml−1 |
0.1 μg ml−1 |
Table 3 Summary of various developed biosensors to detect DENV
Biosensor |
Principle |
Biomarker |
LOD |
Ref. |
Electrochemical |
Impediometric/immunosensor |
DENV-IgG |
2.81 ng ml−1 |
41
|
Immunosensor |
NS1 |
0.069–0.081 ng ml−1 |
42
|
CRISPR/Cpf1 |
Dengue fever viral RNA |
100 fM |
43
|
Differential impedance sensing system |
Dengue virus |
Less than 0.1 ng ml−1 |
44
|
Optical SPR |
Optical based on SPR |
DENV (E-protein) |
0.001 nM/1 pM |
47
|
SPR-optical biosensor |
Dengue virus-2 (E-protein) |
0.08–0.5 pM |
48
|
SPR-optical biosensor |
Dengue virus-2 (E-protein) |
0.08 pM |
49
|
Plasmonic biosensor coupled with on-chip microfluidic-plasma separator |
DENV biomarker (NS-1 protein) |
100–10 000 ng ml−1 |
50
|
Immunosensor based on LSPR |
NS-1 antigen |
70 ± 10 ng ml−1 |
51
|
Portable fiberoptic-SPR platform |
DENV-NS-1 protein |
60 ng ml−1 |
52
|
Colorimetric |
Colorimetric-RT-LAMP |
All serotypes of dengue virus |
N/A |
53
|
Mixing method |
Chikungunya/dengue antibody – IgG/IgM |
Detect within 30 min |
54
|
Colorimetric assay |
DENV-NS-1 |
N/A |
55
|
Colorimetric assay |
Detect protein content in urine samples with IgG/IgM |
N/A |
56
|
LFA |
Magneto-enzyme LFIA |
NS1 protein |
0.25 ng ml−1 |
57
|
LFA-NASBA |
Dengue-1 (RNA) |
1.2 × 104 pfu ml−1 |
58
|
Lateral flow dipstick-RT-RPA |
Dengue serotypes (1/2/3/4) |
10 copies RNA molecules |
59
|
LFIA-paper–plastic microfluidic hybrid chip |
DENV-NS1 antigen |
84.66 ng ml−1 |
60
|
LFIA |
NS-1 |
N/A |
61
|
Microfluidic biosensor |
Microfluidic-based biosensor based on virus-bound magnetic bead complexes |
IgG and IgM |
0.021 ng |
62
|
Microfluidic biosensor with fluorescence |
Dengue virus |
0.125 nM |
63
|
Smartphone based |
Smartphone-based lab-on-a-chip platform |
DENV serotypes |
Quantitative detection in under 15 min |
64
|
RT-LAMP-QUASR-smartphone |
Chikungunya/dengue/Zika |
22 pfu ml−1 |
65
|
Multiplexed-RT-LAMP-hands-free smartphone |
Zika/chikungunya/dengue |
1.56 × 105 pfu ml−1 |
66
|
Mobile app-MOS/DISapp |
Dengue virus |
N/A |
67
|
Smartphone-GPS/ML |
Dengue virus |
N/A |
68
|
ACER model |
Dengue hemorrhagic fever |
N/A |
69
|
a) Electrochemical cell/bulky electrode setup.
Electrodes significantly influence the performance of the electrochemical cell-based biosensor platforms. Most electrochemical cells have 3 electrodes. A reference electrode (RE), typically Ag/AgCl, is positioned away from the reaction site to generate a potential proportional to a stable, known solution. RE ensures that the measurements are normalized. It provides the current, applied to the working electrode (WE). The working electrode, sensing or redox, is the transduction element in biological processes. Both the counter electrode (CE) and WE must be conductive and chemically stable. Common materials for these electrodes include gold, silver, platinum, silicon, carbon, and graphene, depending on the analyte and reaction type.70 Various scientists have used bulky electrochemical cell-based electrode setups to detect dengue virus via electrochemical biosensors. Junior et al.71 constructed an aptasensor using a bulky electrode setup to determine the two DENV serotypes. In this study, they proposed an electrochemical DNA aptasensor to detect NS1 serotypes (Dengue viruses 1/4) in undiluted human serum by employing gold electrodes, and to achieve self-assembled monolayer, the aptamer and MCH, i.e., 6-mercapto-1-hexanol, were co-immobilized. The surface validations were confirmed through electrochemical impedance spectroscopy and AFM. This proposed theory successfully detect NS1 through the advantage of DNA-aptamer, with a linearity of 10 pg to 1 μg ml−1 and has a good LOD of 22 pg ml−1, it also shows excellent selectivity when tested with a negative control, i.e., E-protein. In another study, Kim et al.72 also utilized bulky electrochemical cells to detect dengue fever biomarker (NS1) having a lower great detection limit (1.48 μg ml−1) with the help of electrochemical measurement techniques such as EIS and SWV. In this work, they used gold electrodes, which improved the developed electrochemical biosensor which is highly sensitive to dengue diagnosis. The reports mentioned above validate that a bulky electrode setup can make the electrochemical biosensor more appropriate for the highly sensitive dengue biosensor. However there are some significant limitations of such setups, such as these are constructed utilizing highly expensive electrodes and also utilizing solution in large amounts, difficulty in handling, and many more, which make this setup more expensive. So, scientists introduced screen-printed electrodes as these electrodes were meet more advantages as compared to a bulky setups of an electrochemical biosensors.
b) Screen-printed electrode setup.
The first commercially available screen-printed electrodes were employed to test for diabetes.73 The screen-printed electrodes construction is typically based on the decorative coatings deposition upon smooth surface (e.g., glass). The paste mixture is squeezed via the screens for the required electrode configuration. The screens must be designed according to the use and dimensions needed. The paste is often a combination of components that can transmit electrons, such as gold, silver, or carbon. Tracks or connections are often made of silver.74 Researchers introduced commercialized screen-printed electrodes to overcome the limitations of bulky electrode setup, as screen-printed electrodes meet more benefits, such as low price, require a lower volume of sample, and are portable, and miniaturized setup. One of the significant rewards of the screen-printed electrode is that it can be manufactured with the help of a simple printing method in a short period, while a bulky setup takes multiple expensive instruments to manufacture and takes so much time and technicians.75 Screen-printed electrodes were successfully used in dengue biosensors with a great limit of detection by Alhazmi et al.,76 who utilized a screen-printed electrode by purchasing from PlamSens BV (Houten, Netherlands), in which the working and counter electrodes is made up of carbon and a pseudo-reference electrode made of silver. In this work, they detect the dengue virus antigen with an LOD of 0.16 nM within five minutes using a potentiostat. In another study, Parkash et al.77 validated that the screen-printed electrode can be a capable contender for effectively recognizing dengue virus. They developed an immunosensor-based screen-printed electrode to detect NS-1, which showed a significant response of 0.03 μg ml−1.
c) Paper electrode-based setup.
Commercialized screen electrodes were expensive, could not be purchased in bulk, couldn't be stored for a long time, were toxic to the environment as these electrodes are made up of plastic, and took so much time to purchase from the companies, which also doesn't meet with the POC-based devices criteria. To overcome such limitations, scientists proposed screen-printed electrodes in the lab and used paper substrates instead of plastic, designed as it is based on a wooden screen frame, and the track of electrodes is printed by squeezing the conductive ink on the cellulose paper sheet.78 The prepared electrodes were eco-friendly, inexpensive, could be prepared in 5 minutes, easy to handle, could be modified as required, size can be adjustable, and could effectively detect the dengue virus.79 In a recent report, researchers self-fabricated different paper electrode setups in their lab to construct an aptasensor against DENV-antigen. There only very few reports on paper electrode-based electrochemical dengue biosensors, but other flaviviruses were also effectively detected via paper electrodes, such as by Bishoyi et al.,80 who constructing a paper electrode setup to determine the Zika virus, having a lower limit of detection i.e., 1 mg ml−1. Recently, the chikungunya virus was also detected by Sharma et al.,81 contrived the paper-based electrodes by utilizing a wooden frame and carbon conductive ink and used it to construct an immunosensor that successfully diagnosed the chikungunya virus with high specificity and sensitivity.
Japanese paper-art-based dengue biosensor (origami and kirigami).
Advancing the biosensor doesn't mean involving expensive and sophisticated technology only, using simple paper can also improve the sensing technology in terms of low cost, time-saving, highly sensitive, and selective solution. Such paper techniques were associated with origami, an ancient art form originating from Japan that involves folded paper pieces.82 So simple paper folding can lead to the development of an effectively shaped platform. No reports on the origami-based electrochemical biosensors are available, and only a very few reports were reported based on paper analytical biosensor devices. Biswas et al.83 constructed an origami-based multiplex paper-plastic strip for the detection of all 4 serotypes on a multi-folded origami strip and validated their results with a meager amount of solution (50 μL) of human blood serum and detected within 30 min, showing a great response as well as high specificity. Hasan et al.84 employed an origami approach obtaining for functionalized paper electrode to detect dengue antigen, which show suitable LOD i.e., 0.0001 mg ml−1. Further, an improvised the origami biosensor by incorporating the kirigami approach, which improved sensor sensitivity.85
d) Pencil graphite electrode-based setup (PGE).
Lead present in pencil is composed of natural graphite material, known for its outstanding conductivity, so researchers also devised a pencil idea to employ in the diagnostic field.86 Various researchers used pencil electrodes to create electrochemical biosensors, such as Souza et al., who employed a pencil as a working electrode to diagnose dengue virus (type-1), using a detection limit i.e., 0.92 nM. In this work, they used average pencil lead (4B-type) as a working electrode and a screen-printed electrode as a reference electrode under gold wire, and the working electrode was secured vertically and dipped in a buffer.87 The developed pencil-based platform can diagnose with great sensitivity and affinity.
e) Two- and three-electrode setups.
Generally, electrode setups are employed in two forms of electrode platforms, the first one is a 2-electrode setup, and the other is a 3-electrode setup. In the 2-E setup, only two types of electrodes were present (working and counter electrodes), in the 3-E setup, three different electrodes were present (working, counter, and reference electrodes). Among these, a three-electrode setup is considered more effective than a 2-electrode setup.88,89 Recently, a team of researchers also proved that the three-electrode system is more effective. In their work, they successfully detected dengue antigen by fabricating two types of paper electrodes (2–3 electrode setups) and compared their results in context with dengue virus diagnosis. Both electrode setups detected dengue antigens with a LOD of 0.1 μg ml−1, but both these electrode setups show different current ranges. The three-electrode assembly has a significantly higher current series, reaching from 55.53–322.21 μA, than the two-electrode arrangement, which showing a current variety of 0.85–4.54 μA. Based on the results of this work, the three-electrode system had a worthy range of current intensification that was approximately fifty-times greater than the two-electrode system, which had a weak current reaction. As a result, the three-electrode system has arisen as a feasible choice for highly effective dengue virus analysis, and disease diagnosis in general.90
The above-mentioned electrochemical biosensors have several limitations, including a bulky electrode configuration and conventional approach that did not suit for POC-based devices and a lower potential for commercialization. To overcome such limitations, different and smart technologies were included to advance the electrochemical dengue biosensors to make it more effective by detecting all serotypes on a single platform, as well as the incorporating of 3D printer manufacturing for reshaping the biosensor and also integrating smartphone technology, which provides on-site detection with great portability. Hence All of these incorporations with electrochemical dengue biosensors make them a more eligible candidate towards susceptible detection and commercialization.
3. Advanced electrochemical biosensors for dengue virus detection
In this part, we covered advanced electrochemical dengue biosensors, like multiplex biosensors, 3D printer biosensors, QR-code-linked patients-verified biosensors, smartphone biosensors, and controlling applications that demonstrate remarkable sensitivity and have a strong potential towards commercialization.
3.1. Multiplex platforms for dengue virus detection
The multiplex platform is one of the advanced techniques as such platforms can detect any serotypes of dengue virus and other viruses from the same family, which can be easily detected on a single device with no cross-reactivity.91 Many authors have designed multiplex platform-based electrochemical biosensors to detect DENV. Singhal C. et al.92 demonstrated an electrochemical multiplexed nanosensor based on paper to detect all the serotypes of dengue (DENV-1/2/3/4) on a single device by employing a nanocomposite of graphene oxide/silicon dioxide, which significantly increased the response of this multiplexed paper-based nanosensor due to its excellent conductivity. This proposed theory revealed that all the serotypes of dengue could also be detected via the multiplexed paper-based genosensor in a low range of 100 pM–100 μM, and this theory was the first reported study that predicted the pervasiveness of the dengue shock syndrome/hemorrhagic fever. Not only dengue serotypes but also other viruses can be detected simultaneously. Sampaio et al.93 also developed a multiplex chip to detect different flaviviruses, i.e., Zika and dengue. In this work, they detected both viruses with the help of an electrochemical biosensor. They connected them with a multiplex chip containing two different working electrodes for Zika and dengue, efficiently differentiating both viruses and showing great responses. The proposed platform provides a time-saving diagnosis of both viruses on a single chip, which helps the individual get both reports on a single test. Besides dengue, the chikungunya virus comes from the same family and has a similar infection mechanism, to date, no electrochemical multiplex biosensors have been reported for the simultaneous detection of dengue and chikungunya virus on a single platform.
3.2. 3D-printer-based biosensors
The 3D printer is one of the next-generation printing machines capable of developing innovative prototypes utilized in various fields (Fig. 3).94 Nowadays 3D printers are also used in the diagnostic field to construct various innovative designed biosensors.95 To date, no 3D printer-based electrochemical biosensors have been reported. Only a few reports have illustrated the use of 3D printers in constructing microfluidic biosensors for the detection of dengue, such as Suvanasuthi et al.,96 utilizing commercialized 3D printer-associated PLA (polylactic acid) and wax filaments to construct hydrophobic barriers on a microfluidic paper analytical device. This is the novel design for non-immobilized reactions into a prototype sensor platform. The developed prototype can detect and identify all the serotypes of the dengue virus within a very short time. In 2024, Hasan et al. developed a 3D-printed biosensor for detecting dengue antigen.97
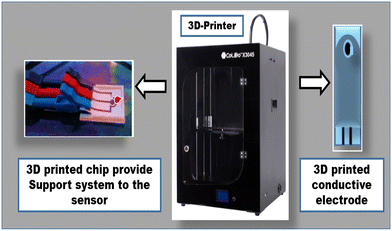 |
| Fig. 3 3D-printer-based prototypes for various sensing applications. 3D-printed conductive electrode helps to make the sensor more sensitive, while it can also provide a support system to the developed sensing platform to avoid manual handling errors. | |
3.3. QR-code-based biosensors
Quick response (QR) codes debuted in the 1990s with several benefits over ordinary barcodes, including the capacity to encode (store) a more significant amount of detail, be faster and simpler to read, and be digitally scanned using a smartphone camera. A conventional QR code is a two-dimensional matrix of numerous tiny blocks organized in a square-shaped grid, with three big squares in each corner to identify the location, size, angle, and exterior form. When a QR code is scanned, the reader (for example, a smartphone) first recognizes the patterns of the three squares before reading the data encoded within it in every possible direction. The widespread usage of smartphones makes QR codes a potential doorway to the internet of things i.e., (IoT) beyond the reach of every single person,98 Because of the widespread use of smartphones, digital codes may now provide individual users with access to the Internet of Things (IoT), and they are also now being used in biosensors. Hasan et al.99 created a QR code by using Internet setting applications to develop an immunosensor for dengue diagnosis. This study, identified multiple serotypes of dengue virus antigen isolated directly from patient serum on a single platform with reasonable detection limit (LOD) of 12.5 ng ml−1. The proposed study demonstrated that the constructed biosensor has various properties, including patient data storage, early detection of dengue viral antigen, and the capacity to identify dengue serotypes from each other. Additionally, the sensor has a high practical application because the antigen was obtained from a dengue patient.
3.4. Smartphone-based dengue biosensors and controlling apps
Smartphones are recognized as unique point-of-care (POC) devices in nanobiosensing due to their popularity, mobility, small components, data gathering capabilities, transmission capacities, effective camera lenses, and inexpensive budget. However, the main requirement for smartphone-based biosensors in making is their ease of use. As per the reports, several approaches were outlined in the proceeding years, but lack this feature. Currently, the usage of smartphones for this purpose has drawn much interest, since it simplifies the diagnosis procedure and eliminate the need for sophisticated devices.100 To our knowledge, no smartphone-based biosensor utilizes a paper electrode chip in dengue biosensors on smartphone mobile handsets. However, a few reports are available on smartphone-based dengue biosensors (Fig. 4).
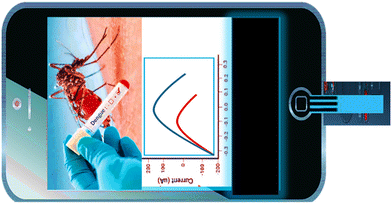 |
| Fig. 4 General diagram of smartphone-based dengue diagnostic. | |
Smartphone-based diagnostic and monitoring are also capable of managing virus-related diseases, as proved by Moser et al.,64 who utilized a handheld smartphone-based lab-on-a-chip platform for the detection of DENV serotypes. In their work, the authors present the translation of smartphone-connected portable lab-on-a-chip (LoC) technology for the quantitative detection of two dengue based on a mix of complementary metal-oxide-semiconductor (CMOS) microchip technology to combine an array of 78 × 56 potentiometric sensors, as well as a label-free RT-LAMP test, the platform connects with a smartphone app, for epidemiological monitoring, synchronizing findings in real time with a secure cloud server housed by Amazon Web Services (AWS). With generated DENV-1 and DENV-2 RNA and extracted RNA from 9 DENV-2 clinical isolates, the assay on the LoC platform (RT-eLAMP) was demonstrated equal performance to gold-standard fluorescence-based real-time equipment (RT-qLAMP), reaching quantitative detection in under 15 min. The developed smart devices were performed at Imperial College London, UK, and Kaohsiung Medical Hospital, Taiwan, to confirm the platform's mobility and geo-tagging capabilities. This method has a substantial strong prospective for use at Point of care (POC) in low-resource situations and demonstrated the potential of real-time cloud synchronization of analytic records for dengue virus surveillance. In another report by Priye et al.,65 simultaneously detected chikungunya/dengue/Zika by combining RT-LAMP with a newly proposed method called QUASR, i.e., quenching of unincorporated amplification via a smartphone, which demonstrated a limit of detection of 22 pfu/ml with the range of linearity from 102–103 pfu. Another author, Ganguli et al.66 demonstrated the multiplexed diagnosis of Zika along with chikungunya and dengue (type 1 and 2) from whole blood by employing a diagnostic card along with RT-LAMP and also fabricating the suitable sequences of primers which are utilized on the printed micro-chip. In the proposed theory, the detection was confirmed with the help of a commercial hands-free smartphone, which amplifies the reaction, followed by displaying the diagnostic readout, having a Zika virus LOD, i.e., 1.56 e5 pfu ml−1. Another author, Babu et al.67 demonstrated two apps (MOSapp/DISapp) based on smartphones to prevent, predict, educate, and control dengue by employing a mosquito perception index (MPI). Both applications support the previously proposed geospatially enabled method called EWARS, i.e., early warning and adaptive response system, in 2008. These two developed apps feed their respective data into the EWARS model, creating risk maps to monitor resource optimization and strengthen disease surveillance, prevention, and response. So, it is hoped that such a method will help to fill gaps in India's dengue surveillance and control system. Sajid et al.68 established an android application for early diagnosis/controlling/tracking of dengue-related issues by using smartphones, GPS and ML. In this proposed theory, they conducted the pilot research with 80 applicants is being done. It was discovered that smartphone technology in conjunction with GPS, can aid in early diagnosis of specific symptoms. Moreover, using GPS expertise is beneficial for surveillance throughout an outbreak. So, this theory concluded that such models can potentially manage the overall burden related to dengue. Recently, Somprasong et al.69 prevented and controlled dengue hemorrhagic fever in a Thailand village i.e., Ban Saikluay, by developing a novel and suitable model called ACER. In their proposed theory, they used the ASER model, especially the villages of south Thailand by employing a mosquito application based on the smartphone. This is a good initiative for highly dengue-influenced areas, which helps them overcome outbreaks of virus-related risks. However, it also requires more experimentation and government support, especially for such rural areas. Recently, Hasan et al.101 integrated a smartphone with an aptasensor for detecting dengue virus. The proposed study successfully detect the target, showing great portability and accuracy for commercialization.
4. Other essential biosensors for dengue diagnosis with novel detection principles
4.1. Optical SPR-based dengue biosensors
Detecting analytes in optical biosensors is aided by optical fibers based on absorption, light scattering, or fluorescence. Waveguide devices and optical fibers are utilized in these optical biosensors and improving sensor performance by enhancing the contact between the light (guiding) and the surface (sensor). Numerous recorded wavelengths can be used to identify different sorts of analytes. An optical biosensor detects and measures changes in a sample's properties, including fluorescence, phase shift, absorbance, and reflectance.102 Numerous studies based on SPR-optical biosensors for the identification of dengue virus have been demonstrated by a team of researchers such as, Omar et al.47 detect DENV (E-protein) by developing an optical sensor based on the sensitive performance of SPR by employing a composite CdS-NH2GO thin film covalently attached this composite with IgM (monoclonal-antibody) by the help of coupled EDC/NHS to which significantly helps in the analysis of E-protein having detection limit of 0.001 nM/1 pM and the enactment of Au/CdS-NH2GO/EDC-NHC/IgM film was positively achieved. Hence it proved that the proposed platform has a tremendous binding affinity and sensitivity toward the dengue virus E-protein. Omar et al.48 detected dengue virus-2 (E-protein) via SPR-optical biosensor by employing a self-assembled DSU/NH2rGO-PAMAM nanocomposite thin film and characterization on this by XRD/FTIR. This proposed sensor shows good linearity, i.e., R2 = 0.92, with an LOD of 0.08–0.5 pM, the lowest detection reported to date. Utilizing the Langmuir model, the equilibrium association constant was calculated to be 6.6844 TM-1 (R2 = 0.99). With multiple competitors' involvement, the SPR sensor demonstrated an excellent selectivity for dengue virus type-2 (E-protein). In another study, Omar et al.49 improvised the selectivity/sensitivity of the same SPR-optical biosensor to diagnose dengue type-2 (E-prt) by employing a nanocomposite of PAMAM dendrimer biopolymer thin film, with the LOD of 0.00008 nM (0.08 pM), which is the lowest concentration was obtained (sensitivity = 333.896° nM−1). The performance of the SPR-optical biosensor was improved as it combined with the nanocomposite thin film (PAMAM dendrimer bipolymer) and also displayed a tremendous binding affinity, i.e., 9.345 TM−1, with the storage of seven days, which indicates decent stability toward the dengue virus. So, the outcome of this proposed sensor confirmed that coupling of SPR with a PAMAM-based nanocomposite thin film (Au/DSU/NH2r GO – PAMAM/IGM) has become favorable diagnostic device in the future for the identification of dengue virus-2 (E-protein) with excellent selectivity and sensitivity. Another author, Vázquez-Guardado et al.50 detected the dengue virus biomarker (NS1-nonstructural protein) in the blood (bovine) by establishing a plasmonic biosensor based on modified ssDNA (synthetic), which provides a great affinity to the NS1-protein found in the genome of the virus in a range of 0.1 to 10 μg ml−1. In this study, the developed plasmonic biosensor was successfully combined with an on-chip microfluidic–plasma separator, which led to significant innovation in building up early diagnostic approaches for infectious diseases. Also, Mahmood et al.51 established a new platform of immunosensor (label-free) based on the (LSPR) term by employing Au-nanosphere probe and Ig-G (monoclonal anti-dengue antibody) to functionalize the platform. And the outcomes this proposed system were performed by numerical calculations, which successfully evaluated the performance of this sensor and detected the NS1-antigen (dengue) with a range as low as 0.07 ± 0.01 μg ml−1. Hence, this integrated immunosensor with LSPR (localized surface plasmon resonance) has excellent potential in the field of plasmonic application for the detecting of infectious diseases. Recently, Gahlaut et al.52 detected the DENV (NS1-protein) at an early stage by using a portable fiber-optic-SPR platform involving the specific binding of an antibody–antigen to detect NS1 (antigen). A self-assembled monolayer of alkanethiols on the surface of Ag-coated unclad fiber helpes promote antibody binding. SPR was used in a wavelength interrogation mode to diagnose antigen (NS1) with a variety of 0.2–2.0 μg ml−1. The resonance wavelength of the 40 nm thick Ag-coated optical fiber was about 500 nm and a variation in the resonant wavelength was observed for every attachment step on the fiber. The sensitivity was determined to be 54.7 nm (μg−1 ml−1) at the lowest concentration of the NS1-antigen with an identification limit of 0.06 μg ml−1, and verified outcomes in samples of red blood serum. This proposed platform could helps mass detected DENV, especially during a pandemic.
4.2. Colorimetric-based dengue biosensors
Colorimetry is defined as the measuring color in a solution. The main schematic of a colorimetric biosensor is shown in Fig. 5. The test produced visible result to the naked eye, encouraging its usage as a large-volume, automated drug-screening system.103
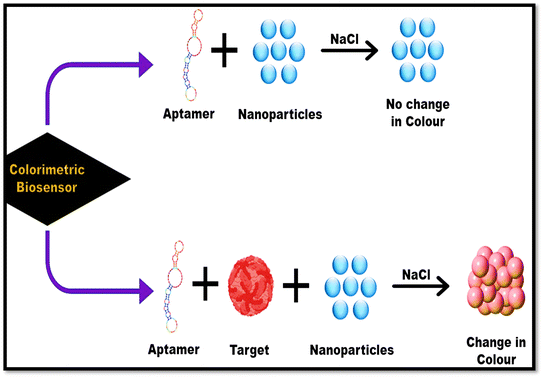 |
| Fig. 5 Diagrammatic representation of a colorimetric biosensor. | |
Many authors worldwide have established colorimetric sensors for the practical analysis of dengue diseases, Carrillo et al.,53 demonstrated a simple colorimetric biosensor coupled with the assay called RT-LAMP (i.e., reverse transcription loop-mediated isothermal amplification, for the easy analysis all four serotypes of DENV at an early stage). All the necessary reactions took place in a single tube by adding all the components, such as reverse-transcriptase/primers/DNA polymerase/samples (serotypes of DENV), then evaluated the process by using different times/temperatures and successfully optimized at 65 °C temperature for 60 min which led to change in the color of the mixture and can be detected via the naked eye that is mainly after the mixing of neutral red dye/visible pH indicator dye, due to amplification, this indicator dye changes its color so that the amplification can work under significantly less buffering circumstances. Hence this proposed theory revealed that the easy ColorRT-LAMP assay could be an ideal option to detect and diagnose dengue compared with q RT-PCR, because ColorRT-LAMP shows excellent specificity, can easily differentiate the dengue virus serotypes and Zika virus, and can also be used to test cross-reactions. So, this fast/easy biosensor has the potential to identify dengue at an initial phase. Colorimetric is capable of detecting dengue, and can detect chikungunya along with dengue, as proved by Wang et al.,54 using chikungunya/dengue antibody (IgG/IgM) within 30 min in human samples. In this study, multiplexed diagnosis of dengue and chikungunya was successfully evaluated based on mixing methods leading to a color change. So, it can be very beneficial in managing the diagnostic burden of infectious diseases. Ramírez-Navarro et al.55 established a colorimetric assay against DENV (NS1) by employing an immuno-conjugate of magnetite-NPs combined with anti-NS1 antibodies. In this, they utilized antibodies against the antigen (NS1) by immobilizing them on superparamagnetic iron-oxide NPs (SPIONS, ∼20 nm), which helps in protein detection. Once the magnetic immuno-nano platform is mixed with serum (infected), it attached to the protein (NS1) and can be readily detached using an external field of the magnet, later the retrieved immuno-conjugate is moved into a well comprising 2nd-immobilized-antibody (NS1) to establish an enzyme-linked immunosorbent setup. Whenever the NS1 protein exists, its interaction with Perls reagent causes a color shift to blue, compatible with the developing of a SPION-antibody-NS1 antigen–antibody conjugate, which verifies infection. There were no false positives when NS1 was absent or another antibody and protein (NS1) were introduced to the assay. So, in the future, this proposed assay can lead to an in situ bimolecular diagnosis assay for infectious diseases. Sometimes DENV can cause proteinuria in patients, due to plasma protein leakage through protein filtration in the kidneys. Charisma et al.56 recently established a colorimetric assay to determine the protein in the sample of urine in patients. This study detected protein content in patients with IgG/IgM dengue or evaluated the protein content in urine samples from people lacking DEN-infection. They also identified the connection between March and June 2020 at Vita Medika Kepung's Clinical Laboratory and Inpatient Clinic in Kediri Regency. In this, the approach, consecutive sampling was adopted. Firstly, two groups were involved, i.e., non-dengue/positive dengue, and the outcomes revealed that proteinuria was identified in 26% of those surveyed, secondary DEN infection and serological test (positive) of IgG and IgM. Only IgG was identified in 24 (92.3%) of participants, and positive IgG and IgM were reported in 2 (7.7%) survey participants, as demonstrated by the p-value of 0.000 in the chi-square tes and a prevalence ratio (PR) of 11
987. Furthermore, chi-square test findings showed that more excellent urine protein or albumin levels were associated with severe illness, with p-values = 0.012 and 0.025 (p 0.05) and PR values of 3.333 or 2.800. According to the findings of this theory, there is a association between high amounts of protein or albumin in urine and the severity of sickness in individuals with DENV infection. Furthermore, a spike in urine protein or albumin concentrations was typically associated with a reduced serum or albumin concentrations. So, this study reported that colorimetric assays has a great potential to detect dengue-related issues suffered by dengue patients.
4.3. LFA-based dengue biosensor
Nowadays, paper-based sensors have shown promise as POC diagnostics since they are affordable, portable, and disposable by burning and have the potential to pump fluids through capillary action. The advent of paper microfluidics has reignited interest in the lateral flow assay (LFA) method which has long been used in point-of-care assays. New studies verified that LFA could produce a much improved detection limit and be utilized as a systems for multiplexing analysis.104 The LFA is executed across a strip, with various pieces integrated on a plastic base. The components of this platform are the sample pad, conjugate pad, nitrocellulose membrane, and adsorption pad. The nitrocellulose membrane is then detached into the test, and control lines. When a liquid sample runs through the strip, pre-immobilized mixtures in numerous strip pads become active (Fig. 6).105
 |
| Fig. 6 Diagrammatic representation of dengue virus-based LFA. | |
Diagnosis of dengue infection is possible with an LFA biosensor, as proved by the team of scientists. Like Tran et al.,57 detected the NS1 protein through a magneto-enzyme lateral flow immuno-assay by employing superparamagnetic NPs as labels and biotin streptavidin, which mainly aided amplifying the signal and helps in early detection of all serotypes of DENV with LOD i.e., 0.25 ng ml−1 for dengue virus 1 and 3, 0.1 ng ml−1 for dengue virus 2, and 1.0 ng ml−1 for dengue virus 4 and this proposed platform it is susceptible and specific as it did not show any cross-reactivity toward JEV/hepatitis – band C and Zika virus. Another author, Yrad et al.58 visually detected of dengue-1 (RNA) via an LFA-based biosensor by employing gold NPs, which was capped with dextrin (first reported to date). The identification relied on a nucleic acid sandwich-type hybridization between a gold nanoparticles-labeled DNA reporter probe, DEN-1 target RNA, and a DENV-1 specific DNA capture probe mounted on a nitrocellulose membrane. A +ve assessment resulted in a test line as red on the lateral flow biosensor strip, allowing for optical identification. This proposed platform had a cut-off value of 0.01 μM utilizing a synthetic DEN-1 target. The biosensor's proof-of-concept implementation identified DENV-1 in the serum of humans, showing a cut-off value of 1.2 × 104 pfu ml−1. Combined with NASBA, i.e., nucleic acid sequence-based amplification, isolated viral RNA was identified upon lateral flow biosensor within 20 min. Combining LFA with other techniques can lead to great diagnostic approaches against dengue infections, demonstrated by Xi and their co-workers,59 demonstrated a lateral flow dipstick coupled with the practical method called RT-RPA, i.e., reverse transcription-recombinase polymerase amplification, which effectively diagnosed all serotypes of dengue virus (1/2/3/4) with an LOD of 10 copies of RNA molecules. This RT-RPA-LFD approach is particular as it does not show any reactions toward other human pathogens. The outcomes of this coupled lateral flow dipstick assay are much faster than those RT-qPCR in terms of clinical samples. This proposed biosensor no expensive instruments, making it more beneficial in terms of budget. Another coupling approach of LFA was established by Yuzon and co-workers.60 In this work they integrated a lateral flow immunoassay through a paper-plastic microfluidic hybrid chip identify DENV-NS1 (antigen) with a limit of detection of 84.66 ng ml−1. Three materials were used to fabricate the microfluidic: PMMA-sheet/NC-membrane/CA-film. The procedure entails printing a wax design on the CA film and oven-baking the pattern with supported NC membrane, at 120 °C for 2.5 min. The porous structure of the NC membrane permits the wax design to penetrate its pores at the time of baking period, therefore, binding the backed NC membrane to the CA film. The etched PMMA layer was attached to strengthen the structure. The layer's etched hole provided an area for the conjugate pad to be integrated into the platform. Furthermore, the antibodies necessary for the chip's integration with the later flow immunoassay were chosen using an ELISA. Recently, Axelrod et al.61 detected the NS1 via a lateral flow immunoassay. This theory provides a novel strategy for an LFIA system. All the membranes on this platform are cheap and straightforward. The third membrane consisted of a test and control line and is used here as a functional capture layer for filtering molecules out of the stream, and the 4th membrane doubled as a test pad, and a dry substrate generated the signal and delivered pulling forces for liquid samples. This method was evaluated and establish to be valuable against dengue-NS1 proteins, however, it easy adapt for other analytes. This novel arrangement permits the usage of the substrate in a one-step lateral flow assay, and therefore also enables quantitation.
4.4. Microfluidic-based biosensors for dengue diagnosis
Microfluidic systems involve sample handling, reagent mixing, separation, and identification procedures on a single chip. Such miniaturization shows several potential benefits, including minimal reagent and power consumption, less handling of hazardous chemicals, rapid reaction times, mobility and diversity in design, and parallel operating capacity (Fig. 7).106,107 A detailed summary of breakthroughs in theoretical knowledge, expertise, and uses in the arena of μTAS and microfluidics is presented by Reyes and their co-workers. Traditional diagnosis approaches, such as PCR, electrophoresis, immunoassays and many more have been miniaturized into a chip arrangement, demonstrating proof of principle. Microfluidics is undoubtedly one of the distinct chip-based biosensors, but integrating various techniques and functional elements into a single chip to carry out comprehensive sample analysis is undoubtedly the most challenging aspect of microfluidics.108 Few reports are based on microfluidic biosensors against dengue virus, such as Lee and their co-workers,62 developed a microfluidic-based biosensor based on virus-bound magnetic bead complexes and integrating it with 1-way micropumps, 4-membrane type micro-mixer, 2-way micropumps, and an on-chip micro coil array and utilized this system for the fast and immediate finding of dengue IgG and IgM within 30 min, automatically showing great LOD i.e., 21 pg. In another study, Zaytseva et al.63 constructed a microfluidic biosensor with fluorescence finding of the dengue virus RNA (specific serotype), with the LOD of 0.125 nM in a very short time, i.e., 20 min. In this platform, the microfluidic chip was based on a PDMS substrate on which microchannels were fabricated, and glass substrates were employed to seal the channels, which made this system more advanced in terms of rapid and sensitive detection.
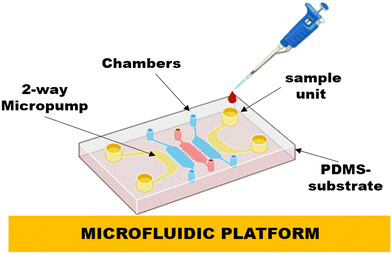 |
| Fig. 7 Diagrammatic representation of dengue virus-based microfluidic platform. | |
The existing dengue biosensors provided good results for dengue diagnosis, favoring affordability and accuracy. As the world moves toward next-generation technology, researchers are focus on making the sensor more smart and effective by building futuristic dengue biosensors using next-generation approaches, such as robotics, drones, satellite, airspace, and Internet technology.
5. Future applications for dengue virus diagnosis and management
The future of biosensors looks very bright due to their excellent integration capability. Scientists are already tried their best to make this diagnostic devices more advanced towards effective and innovative dengue diagnosis. This section summarizes different next-generation dengue biosensors to boost the future of dengue diagnosis, such as lab-on-a-drone biosensors, robotic biosensors, internet technology-based biosensors, and satellites for dengue management.
In the future, various platforms can be integrated with traditional dengue biosensors. Recently, the world has suffered from a deadly, coronavirus, which is highly infectious. A main issue was that individuals could not leave their homes and that no medical personnel were permitted inside, especially in high alert zones. So, to overcome that limitation, unmanned biosensors were much needed to detect infectious viruses in their home during quarantine. Drone biosensors, hexacopters, unmanned systems, aerial systems, and lab-on-air and lab-on-drone based dengue biosensors are example. Drones were the primary air carriers in such biosensors, and they might be used with miniature biosensors to reach the alert zone and identify infected persons in a single trip, allowing people to take their dengue test locally rather than traveling to hospitals.109 Other than drone technology, robotics technology is also popular and an advanced technology that is utilized in various fields, such as electronics/software engineering companies, and medical surgery and treatments. Currently, researchers try to use this technology in the diagnosis sector as well, and a few reports are available on robotics-based biosensors, such as food tasting and liquid handling biosensors.110,111 But till now no robotic-based biosensors are reported for dengue virus detection, so using robot-based dengue biosensors will provide numerous benefits, such as it reduces the labor load in laboratories, increasing the number of experiments, accelerates the analysis advanced procedures, allows for bigger experimentation, fast sensing, and eliminate the essential for additional laboratory specialists in the investigational laboratory to analyses results.112 Other than this, thanks to internet technology/5G technology, people are now able to receive treatment at home in addition to receiving diagnoses. This has led to the development of new terms like telemedicine, e-prescriptions, e-health, IoMT, and AI-based dengue treatments. These techniques will enhance dengue virus detection and management, with compensation like on-site analysis, multitasking, high-grade pictures, and data examination via AI, and they can upload data for cloud figuring. Today, 5G skills is growing, greatly benefiting the healthcare industry and allowing for telemedicine.113 Telemedicine allows patients to obtain medical maintenance without visiting a clinic.114 Customers will be able to receive tests at a sensible cost from a drugstore near their homes shortly. Users can able to conduct a dengue test at home in minutes and then submit the consequences to the doctor through a video call in seconds, dramatically reducing diagnostic, and cure time, and the infectious threat disease spreading in public. If the test findings are unusual, the doctor can generate an e-prescription. In more critical cases, the doctor may order further challenging if the consequences show that a severe problem exists. IoMT also helps dengue biosensors as it fulfills the criteria of POC-based biosensors and compromises wireless-based processes and connectivity with POCT biosensors with health experts and health centers. Hence coupling dengue virus and disease management with Internet technology will effectively diagnosis and the manage the infections with proper investigations using such approaches.115 Satellite-based dengue management improve dengue fever control and prevention by leveraging satellite technology. Satellites aid in identifying mosquito breeding locations by monitoring environmental factors, such as weather patterns and land usage. They give essential disease transmission and population density mapping, allowing targeted treatments. Predictive modeling with satellite data enables anticipating probable epidemics and delivering early warnings. During an emergency, satellite imaging help with resource allocation and damage assessment. Furthermore, satellite-based visualizations are utilized to educate the public on preventive actions. Overall, this method increases the effectiveness of dengue control by implementing timely and data-driven interventions.116,117
6. Conclusion and future perspective
DENV is one of the central transmissible infections, and it continues to produce significant outbreaks and fatalities in many countries. Since there is currently no treatment or vaccination to reduce the disease's impact, early detection and sensitive diagnostics are crucial to the illness's care. From a diagnostic point of view, conventional methods are effectively used in many laboratories, such as HI assays, PCR, ELISA, PRNT, and virus isolation. However, these conventional methods are costly and time-consuming and show cross-reactivity with other flaviviruses. To lower such restriction the biosensor is essential as it has numerous advantages, such as being inexpensive and fast, and no sophisticated instrumentation or expertise is needed. Along with this biosensors, various scientists have focused on the smartphone-based biosensors, as we all know that more than 65% of the world population uses smartphones, which can help teach and make every person. Many mobile-based applications were also developed based on dengue diagnosis and such mobile applications were also made particularly for influential town or state. This review shows the development of effective biosensing approaches, such as electrochemical, SPR, LFA, colorimetric and smartphone-based biosensors, and mobile applications, that help detect and monitor dengue. As years have passed by with dengue infection, scientists have tried their best to improve diagnostic techniques to detect DENV. And yes, today, dengue diagnosis has improved so much as the scientists designed their practical approaches particularly for dengue-affected areas which helps out controlling and managing the dengue disease. So, scientists are genuinely responsible for where the dengue diagnostic field is today as they work on dengue-affected areas. In the future, dengue biosensors can be quickly commercialized in the market with innovative POC care-approved handheld sensors by developing 3D printer-based paper electrodes integrated with smartphone mobiles for the detection of dengue virus, along with dengue-smart apps that help users to get proper knowledge of dengue on its fingertips. However trials and government involvement are still required to research appropriately.
List of abbreviations
1. DENV | Dengue virus |
2. DSS | Dengue shock syndrome |
3. DHF | Dengue hemorrhagic fever |
4. PCR | Polymerase chain reaction |
5. CME | Chemically modified electrode |
6. LOD | Limit of detection |
7. DIS | Differential impedance sensing |
8. NPs | Nanoparticles |
9. EIS | Electrochemical impedance spectroscopy |
10. SWV | Square wave voltammetry |
11. CRISPR | Clustered regularly interspaced short palindromic repeats |
12. LFA | Lateral flow assay |
13. AFM | Atomic force microscopy |
14. PGE | Pencil graphite electrode-based setup |
15. NS-1 | Nonstructural protein-1 |
16. EWARS | Early warning and adaptive response system |
17. GPS | Global positioning system |
18. POC | Point of care |
19. μTAS | Micro total analysis |
20. 3D-printer | Three-dimensional printer |
21. e-Prescriptions | Electronic prescriptions |
22. e-Health | Electronic health |
23. IoMT | Internet of medical things |
24. AI | Artificial intelligence |
24. 5G technology | Fifth-generation technology |
25. HI | Hemagglutination inhibition |
Ethical approval
Ethical approval is not required as this is a review article.
Data availability
Data will be available on request.
Conflicts of interest
The authors confirm they have no conflicts of interest to declare.
Acknowledgements
This work was associated with ICMR, New Delhi.
References
- P. Weeratunga, C. Rodrigo, S. D. Fernando and S. Rajapakse, Cochrane Database Syst. Rev., 2017, 8, 1–10 Search PubMed.
- I. Dieng, M. D. Cunha, M. M. Diagne, P. M. Sembène, P. M. Zanotto and O. Faye, Viruses, 2021, 13–57 Search PubMed.
- S. P. Wijayanti, S. Sunaryo, S. Suprihatin, M. McFarlane, S. M. Rainey, I. Dietrich, E. Schnettler, R. Biek and A. Kohl, PLoS Neglected Trop. Dis., 2016, 10–11 Search PubMed.
- A. Wilder-Smith, K. E. Renhorn, H. Tissera, S. Abu Bakar, L. Alphey, P. Kittayapong, S. Lindsay, J. Logan, C. Hatz, P. Reiter and J. Rocklöv, Glob. Health Action, 2012, 5–17273 Search PubMed.
- V. K. Pathak and M. Mohan, J. Family Med. Prim. Care, 2019, 8–3125 Search PubMed.
- B. Pozzetto, M. Memmi and O. Garraud, J. Virol., 2015, 4–113 Search PubMed.
- C. Singhal, C. S. Pundir and J. Narang, Biosens. Bioelectron., 2017, 75–82 CrossRef CAS.
- A. Somprasong, W. Choeibuakaew and P. Pinto, Adv. Health Sci. Res., 2022, 43, 222–225 Search PubMed.
- S. B. Halstead, Science, 1988, 476–481 CrossRef CAS.
- O. Parkash and R. H. Shueb, Viruses, 2015, 5410–5427 CrossRef CAS.
- S. S. Hegde and B. R. Bhat, Biosens. Bioelectron.: X, 2022, 10–100100 Search PubMed.
- A. A. Sebayang, H. Fahlena, V. Anam, D. Knopoff, N. Stollenwerk, M. Aguiar and E. Soewono, Biology, 2021, 10(9), 941 CrossRef.
- L. Fernández-García, J. Angulo, H. Ramos, A. Barrera, K. Pino, J. Vera-Otarola and M. López-Lastra, J. Virol., 2020, e01998-20 Search PubMed.
- A. J. Baeumner, N. A. Schlesinger, N. S. Slutzki, J. Romano, E. M. Lee and R. A. Montagna, Anal. Chem., 2002, 1442–1448 CrossRef CAS.
- J. Whitehorn and J. Farrar, Br. Med. Bull., 2010, 161–173 CrossRef.
- V. Tricou, N. N. Minh, T. P. Van, S. J. Lee, J. Farrar, B. Wills, H. T. Tran and C. P. Simmons, PLoS Neglected Trop. Dis., 2010, 4–8 Search PubMed.
- S. S. Whitehead, J. E. Blaney, A. P. Durbin and B. R. Murphy, Nat. Rev. Microbiol., 2007, 518–528 CrossRef CAS.
- A. Wilder-Smith, Curr. Opin. Virol., 2020, 71–78 CrossRef CAS PubMed.
- B. A. Prabowo, P. D. Cabral, P. Freitas and E. Fernandes, Chemosensors, 2021, 9(11), 299 CrossRef CAS.
- O. Parkash, M. A. Abdullah, C. Y. Yean, S. D. Sekaran and R. H. Shueb, Diagnostics, 2020, 11(1), 33 CrossRef.
- L. Chen, H. Wang, T. Guo, C. Xiao, L. Liu, X. Zhang, B. Liu, P. Li, A. Liu, B. Li and B. Li, J. Immunol. Methods, 2018, 456, 23–27 CrossRef CAS.
- M. R. Hasan, S. Singh, P. Sharma, C. S. Pundir, K. Bhalla, M. Z. Abdin and J. Narang, Int. J. Environ. Anal. Chem., 2024, 31, 1–7 CrossRef.
- M. Hussain, Z. Ali, B. I. Liu, J. Dai, X. Liu, J. Zhu and Y. Tang, Biocell, 2022, 46(1), 61 CAS.
- N. A. Omar and Y. X. Fen, Sens. Rev., 2018, 106–116 CrossRef.
- J. Deng and C. S. Toh, Sensors, 2013, 7774–7785 CrossRef CAS PubMed.
- N. Kavitha, M. Elavarasan, R. Ramachandran, S. Uthayakumar, A. Chandramohan and K. Dinakaran, Curr. Res. Green Sustainable Chem., 2022, 5, 100316 CrossRef CAS.
- G. Thergarajan and S. D. Sekaran, Expert Rev. Mol. Diagn., 2023, 643–651 CrossRef CAS.
- S. O. De Paula and B. A. Fonseca, Braz. J. Infect. Dis., 2004, 8, 390–398 CAS.
- F. R. Teles, D. M. Prazeres and J. L. Lima-Filho, Rev. Med. Virol., 2005, 15(5), 287–302 CrossRef CAS.
- N. T. Darwish, Y. B. Alias and S. M. Khor, TrAC, Trends Anal. Chem., 2015, 1(67), 45–55 CrossRef.
- A. Thiha and F. Ibrahim, Sensors, 2015, 15(5), 11431–11441 CrossRef CAS.
- R. W. Peeling, H. Artsob, J. L. Pelegrino, P. Buchy, M. J. Cardosa, S. Devi, D. A. Enria, J. Farrar, D. J. Gubler, M. G. Guzman and S. B. Halstead, Nat. Rev. Microbiol., 2010, 8(12), 30–37 CrossRef PubMed.
- M. Ahmed, N. Mahboob, K. T. Mamun and H. Iqbal, Bangladesh Journal of Medicine, 2019, 30(2), 83–92 CrossRef.
- S. S. Hegde and B. R. Bhat, Biosens. Bioelectron.: X, 2022, 1(10), 100100 Search PubMed.
- S. Vigneshvar, C. C. Sudhakumari, B. Senthilkumaran and H. Prakash, Front. Bioeng. Biotechnol., 2016, 4–11 Search PubMed.
- B. Bohunicky and S. A. Mousa, Nanotechnol., Sci. Appl., 2011, 4, 1–10 CAS.
- M. A. Alam, M. R. Hasan, N. Anzar, S. Suleman and J. Narang, Process Biochem., 2021, 156–168 CrossRef CAS.
- N. Anzar, M. R. Hasan, M. Akram, N. Yadav and J. Narang, Process Biochem., 2020, 126–135 CrossRef CAS.
- D. R. Thévenot, K. Toth, R. A. Durst and G. S. Wilson, Biosens. Bioelectron., 2001, 121–131 CrossRef PubMed.
- A. Ahmadalinezhad and A. Chen, Biosens. Bioelectron., 2011, 4508–4513 Search PubMed.
- O. Y. Siew, E. L. Pang, H. S. Loh and M. T. Tan, Biosens. Bioelectron., 2021, 176–112895 Search PubMed.
- S. Solanki, A. Soni, V. V. Agrawal, M. K. Pandey and G. Sumana, Langmuir, 2021, 8705–8713 CrossRef CAS PubMed.
- Y. Lee, J. Choi, H. K. Han, S. Park, S. Y. Park, C. Park, C. Baek, T. Lee and J. Min, Sens. Actuators, B, 2021, 326, 128677 CrossRef CAS.
- P. Piedimonte, L. Sola, M. Cretich, A. Gori, M. Chiari, E. Marchisio, P. Borga, R. Bertacco, A. Melloni, G. Ferrari and M. Sampietro, Biosens. Bioelectron., 2022, 15, 113996 CrossRef.
- B. B. Junior, M. R. Batistuti, A. S. Pereira, E. M. de Sousa Russo and M. Mulato, Talanta, 2021, 233–122527 Search PubMed.
- M. R. Hasan, P. Sharma, S. Singh, S. Kumar, K. Rani and J. Narang, Sens. Int., 2024, 1(5), 100285 CrossRef.
- N. A. Omar, Y. W. Fen, J. Abdullah, M. H. Zaid, W. M. Daniyal and M. A. Mahdi, Opt. Laser Technol., 2019, 204–208 CrossRef CAS.
- N. A. Omar, Y. W. Fen, J. Abdullah, Y. M. Kamil, W. M. Daniyal, A. R. Sadrolhosseini and M. A. Mahdi, Sci. Rep., 2020, 1–5 CAS.
- N. A. Omar, Y. W. Fen, I. Ramli, A. R. Adrolhosseini, J. Abdullah, N. A. Yusof, Y. M. Kamil and M. A. Mahdi, Polymer, 2021, 5–762 Search PubMed.
- A. Vázquez-Guardado, F. Mehta, B. Jimenez, A. Biswas, K. Ray, A. Baksh, S. Lee, N. Saraf, S. Seal and D. Chanda, Nano Lett., 2021, 7505–7511 CrossRef PubMed.
- H. Z. Mahmood, A. Jilani, S. Farooq, Y. Javed, Y. Jamil, J. Iqbal, S. Ullah and S. Wageh, Plasmon-Based Label-Free Biosensor Using Gold Nanosphere for Dengue Detection, Crystals, 2021, 11–1340 Search PubMed.
- S. K. Gahlaut, A. Pathak, B. D. Gupta and J. P. Singh, Biosens. Bioelectron., 2022, 196–113720 Search PubMed.
- C. Carrillo, S. Werbajh, C. Malnero, F. Stolowicz, L. Larocca, V. Malirat and A. Vojnov, Int. J. Infect. Dis., 2018, 73–171 Search PubMed.
- R. Wang, S. Y. Ongagna-Yhombi, Z. Lu, E. Centeno-Tablante, S. Colt, X. Cao, Y. Ren, W. B. Cárdenas, S. Mehta and D. Erickson, Anal. Chem., 2019, 5415–5423 CrossRef CAS.
- R. Ramírez-Navarro, P. Polesnak, J. Reyes-Leyva, U. Haque, J. C. Vazquez-Chagoyán, M. R. Pedroza-Montero, M. A. Méndez-Rojas and A. Angulo-Molina, Nanoscale Adv., 2020, 3017–3026 RSC.
- A. M. Charisma, E. A. Farida and F. Anwari, Indonesian Journal of Clinical Pathology and Medical Laboratory, 2021, 244–248 CrossRef.
- T. V. Tran, B. V. Nguyen, T. T. Nguyen, T. T. Tran, K. G. Pham, Q. B. Le, B. N. Do, H. N. Pham, C. V. Nguyen, D. P. Dinh and V. T. Ha, PeerJ, 2019, 7–7779 Search PubMed.
- F. M. Yrad, J. M. Castañares and E. C. Alocilja, Diagnostics, 2019, 3–74 Search PubMed.
- Y. Xi, C. Z. Xu, Z. Z. Xie, D. L. Zhu and J. M. Dong, Mol. Cell. Probes, 2019, 46–101413 Search PubMed.
- M. K. Yuzon, J. H. Kim and S. Kim, BioChip J., 2019, 277–287 CrossRef CAS.
- T. Axelrod, E. Eltzov and R. S. Marks, ACS Omega, 2020, 10433–10440 CrossRef CAS PubMed.
- Y. F. Lee, K. Y. Lien, H. Y. Lei and G. B. Lee, Biosens. Bioelectron., 2009, 745–752 CrossRef CAS.
- N. V. Zaytseva, R. A. Montagna and A. J. Baeumner, Anal. Chem., 2005, 7520–7527 CrossRef CAS.
- N. Moser, L. S. Yu, J. Rodriguez Manzano, K. Malpartida-Cardenas, A. Au, P. Arkell, C. Cicatiello, A. Moniri, L. Miglietta, W. H. Wang and S. F. Wang, Front. Bioeng. Biotechnol., 2022, 10, 892853 CrossRef.
- A. Priye, S. W. Bird, Y. K. Light, C. S. Ball, O. A. Negrete and R. J. Meagher, Sci. Rep., 2017, 1 CAS.
- A. Ganguli, A. Ornob, H. Yu, G. L. Damhorst, W. Chen, F. Sun, A. Bhuiya, B. T. Cunningham and R. Bashir, Biomed. Microdevices, 2017, 11–13 Search PubMed.
- A. N. Babu, E. Niehaus, S. Shah, C. Unnithan, P. S. Ramkumar, J. Shah, V. V. Binoy, B. Soman, M. C. Arunan and C. P. Jose, Environ. Monit. Assess., 2019, 1–7 Search PubMed.
-
A. Sajid and M. A. Awan, 2021 16th International Conference on Emerging Technologies (ICET), IEEE, 2021, pp. 1–6 Search PubMed.
-
A. Somprasong, W. Choeibuakaew and P. Pinto, Conference on Interdisciplinary Approach in Sports in conjunction with the 4th Yogyakarta International Seminar on Health, Physical Education, and Sport Science (COIS-YISHPESS 2021), Atlantis Press, 2022, pp. 222–225 Search PubMed.
- S. Damiati and B. Schuster, Sensors, 2020, 20–1721 Search PubMed.
- B. B. Junior, M. R. Batistuti, A. S. Pereira, E. M. de Sousa Russo and M. Mulato, Talanta, 2021, 233–122527 Search PubMed.
- J. H. Kim, C. H. Cho, M. Y. Ryu, J. G. Kim, S. J. Lee, T. J. Park and J. P. Park, PLoS One, 2019, 14–19 Search PubMed.
- H. A. Abdulbari and E. A. Basheer, ChemBioEng Rev., 2017, 92–105 CrossRef.
- A. G. Ferrari, S. J. Rowley-Neale and C. E. Banks, Talanta Open, 2021, 3–100032 Search PubMed.
- A. Neubauer, D. Pum and U. B. Sleytr, Anal. Lett., 1993, 1347–1360 CrossRef CAS.
- H. A. Alhazmi, W. Ahsan, M. M. Taha, M. Albratty, A. Najmi, A. Farasani, A. A. Abdulhaq and I. A. Darwish, J. King Saud Univ., Sci., 2023, 35, 102568 CrossRef.
- O. Parkash, C. Y. Yean and R. H. Shueb, Diagnostics, 2014, 165–180 CrossRef.
- M. R. Hasan, N. Anzar, M. Tyagi, N. Yadav and J. Narang, Sensors International, 2020, 1, 100003 CrossRef.
-
M. R. Hasan, S. Suleman and J. Narang, Sensing Tools and Techniques for COVID-19, 2022, pp. 25–47 Search PubMed.
- A. Bishoyi, M. A. Alam, M. R. Hasan, M. Khanuja, R. Pilloton and J. Narang, Micromachines, 2022, 12–2037 Search PubMed.
- P. Sharma, H. Hassan, M. R. Hasan, T. Fatima, H. Mohan, M. Khanuja, S. Kaushik and J. Narang, Biosens. Bioelectron.: X, 2023, 1–13 Search PubMed.
- S. M. Russell, A. Alba-Patiño, M. Borges and R. de la Rica, ACS Sens., 2018, 1712–1718 CrossRef CAS.
- P. Biswas, G. N. Mukunthan Sulochana, T. N. Banuprasad, P. Goyal, D. Modak, A. K. Ghosh and S. Chakraborty, All-serotype dengue virus detection through multilayered origami-based paper/polymer microfluidics, ACS Sens., 2022, 7(12), 3720–3729 CrossRef CAS.
- M. R. Hasan, S. Singh, P. Sharma, C. Rawat, M. Khanuja, R. Pilloton and J. Narang, Sensors, 2024, 24(3), 801 CrossRef CAS.
- M. R. Hasan, S. Singh, P. Sharma, Z. Azmi, A. S. Dadial and J. Narang, Biomed. Microdevices, 2024, 26(2), 21 CrossRef CAS.
- S. Sharma, R. Jain and A. N. Raja, J. Electrochem. Soc., 2020, 3, 037501 Search PubMed.
- E. Souza, G. Nascimento, N. Santana, D. Ferreira, M. Lima, E. Natividade, D. Martins and J. Lima-Filho, Sensors, 2011, 5616–5629 CrossRef CAS.
- R. Alhans, A. Singh, C. Singhal, J. Narang, S. Wadhwa and A. Mathur, Mater. Sci. Eng., C, 2018, 273–279 CrossRef CAS PubMed.
- N. K. Mehto, P. Sharma, S. Kumar, M. Khanuja, R. Rawal and J. Narang, Process Biochem., 2022, 36–43 CrossRef CAS.
- M. R. Hasan, P. Sharma, S. Shaikh, S. Singh, R. Pilloton and J. Narang, Biosensors, 2022, 13, 1–9 CrossRef.
- M. J. Huang, H. Xie, Q. Wan, L. Zhang, Y. Ning and G. J. Zhang, J. Nanosci. Nanotechnol., 2013, 3810–3817 CrossRef CAS PubMed.
- C. Singhal, S. K. Shukla, A. Jain, C. Pundir, M. Khanuja, J. Narang and N. P. Shetti, ACS Biomater. Sci. Eng., 2020, 5886–5894 CrossRef CAS.
- I. Sampaio, F. D. Quatroni, J. N. Costa and V. Zucolotto, Biosens. Bioelectron., 2022, 216–114630 Search PubMed.
- A. Haleem and M. Javaid, Clin. Epidemiol. Global Health, 2020, 215–223 CrossRef.
- R. Amin, S. Knowlton, A. Hart, B. Yenilmez, F. Ghaderinezhad, S. Katebifar, M. Messina, A. Khademhosseini and S. Tasoglu, Biofabrication, 2016, 8(2), 022001 CrossRef PubMed.
- R. Suvanasuthi, S. Chimnaronk and C. Promptmas, Talanta, 2022, 237, 122962 CrossRef CAS.
- M. R. Hasan, P. Sharma, S. Singh, A. Mishra, Z. Azmi and J. Narang, Biosens. Bioelectron.: X, 2024, 1(16), 100431 Search PubMed.
- P. Escobedo, C. E. Ramos-Lorente, A. Ejaz, M. M. Erenas, A. Martínez-Olmos, M. A. Carvajal, C. García-Núñez, I. de Orbe-Payá, L. F. Capitán-Vallvey and A. J. Palma, Sens. Actuators, B, 2023, 1(376), 133001 CrossRef.
- M. R. Hasan, P. Sharma, Y. Mehta, A. Angel, B. Angel, V. Joshi and J. Narang, Biosens. Bioelectron., 2024, 1(263), 116561 CrossRef PubMed.
- R. Eivazzadeh-Keihan, P. Pashazadeh-Panahi, T. Mahmoudi, K. K. Chenab, B. Baradaran, M. Hashemzaei, F. Radinekiyan, A. Mokhtarzadeh and A. Maleki, Microchim. Acta, 2019, 1–24 CAS.
- M. R. Hasan, P. Sharma, S. Singh and J. Narang, ACS Appl. Bio Mater., 2024, 7(4), 2299–2308 CrossRef CAS.
- M. R. Hasan, P. Sharma, N. Anzar, C. S. Pundir, R. Pilloton, J. Narang and N. P. Shetti, Biomedical Engineering Advances, 2021, 1–100006 Search PubMed.
- E. Vega-Avila and M. K. Pugsley, Proc. West. Pharmacol. Soc., 2011, 10–14 CAS.
- B. A. Rohrman, V. Leautaud, E. Molyneux and R. R. Richards-Kortum, PLoS One, 2012, 45611 CrossRef.
- M. Sajid, A. N. Kawde and M. Daud, J. Saudi Chem. Soc., 2015, 689–705 CrossRef.
-
K. F. Lei, Materials and fabrication techniques for nano-and microfluidic devices, 2014, pp. 1–28 Search PubMed.
- M. Garg, M. G. Christensen, A. Iles, A. L. Sharma, S. Singh and N. Pamme, Biosensors, 2020, 8–91 Search PubMed.
- N. V. Zaytseva, V. N. Goral, R. A. Montagna and A. J. Baeumner, Lab Chip, 2005, 805–811 RSC.
- T. Beduk, D. Beduk, M. R. Hasan, E. Guler Celik, J. Kosel, J. Narang, K. N. Salama and S. Timur, Biosensors, 2022, 8–583 Search PubMed.
- B. Ciui, A. Martin, R. K. Mishra, T. Nakagawa, T. J. Dawkins, M. Lyu, C. Cristea, R. Sandulescu and J. Wang, ACS Sens., 2018, 2375–2384 CrossRef CAS PubMed.
- C. E. Anderson, T. Huynh, D. J. Gasperino, L. F. Alonzo, J. L. Cantera, S. P. Harston, H. V. Hsieh, R. Marzan, S. K. McGuire, J. R. Williford and C. I. Oncina, Anal. Bioanal. Chem., 2022, 414, 2607–2618 CrossRef CAS PubMed.
-
T. Huynh, C. E. Anderson, D. J. Gasperino, S. P. Harston, H. V. Hsieh, R. Marzan, J. R. Williford, C. I. Oncina, V. A. Glukhova, D. M. Cate and K. P. Nichols, 2019 IEEE Global Humanitarian Technology Conference (GHTC), IEEE, 2019, pp. 1–4 Search PubMed.
- K. Yu, L. Tan, L. Lin, X. Cheng, Z. Yi and T. Sato, IEEE Wirel. Commun., 2021, 54–61 Search PubMed.
- Y. Zhu, X. Gu and C. Xu, Heart Failure Rev., 2020, 231–243 CrossRef.
- S. Jain, M. Nehra, R. Kumar, N. Dilbaghi, T. Hu, S. Kumar, A. Kaushik and C. Z. Li, Biosens. Bioelectron., 2021, 179–113074 Search PubMed.
- L. Eisen and S. Lozano-Fuentes, PLoS Neglected Trop. Dis., 2009, 3(4), e411 CrossRef.
-
K. M. Fornace, E. Johnson, M. Moreno, A. Hardy and G. Carrasco-Escobar, in Planetary Health Approaches to Understand and Control Vector-Borne Diseases, ed. K. Fornace, J. Conn, M. A. M. Sallum, L. S. M. Chaves and J. Logan, Series: Ecology and control of vector-borne diseases, Wageningen Academic Publishers, 2023, pp. 319–346 Search PubMed.
|
This journal is © The Royal Society of Chemistry 2025 |
Click here to see how this site uses Cookies. View our privacy policy here.