DOI:
10.1039/D4SC07594C
(Edge Article)
Chem. Sci., 2025,
16, 4815-4820
Room temperature crystal field splitting of curium resolved by circularly polarized luminescence spectroscopy†
Received
9th November 2024
, Accepted 5th February 2025
First published on 6th February 2025
Abstract
Coordination of Cm(III) with a chiral decadentate ligand N,N,N′,N′-tetrakis[(6-carboxypyridin-2-yl)methyl]-1,2-diaminocyclohexane (tpadac) generated complexes with strong luminescence allowing for the unprecedented measurement of well-resolved Cm(III) circularly polarized luminescence spectra. Quantitative resolution of the electronic structure of the [Cm(tpadac)][K] complexes was achieved at room temperature, highlighting the strength of the combination of luminescence and circularly polarized luminescence spectroscopies to unravel the fundamental electronic structure of Cm(III). These results are a clear demonstration that these spectroscopies are powerful yet simple tools for the fundamental understanding of electronic structure, which opens the door to future investigations of other Cm(III) complexes in geometries relevant to nuclear applications, and even other 5f-elements.
Introduction
The current revitalization of the civil nuclear industry coupled with renewed geopolitical tensions underscores the need for a fundamental understanding of the chemistry of the transplutonium elements. In the event of accidental or otherwise uncontrolled environmental release of nuclear material, reclamation of small amounts of disseminated radioactive isotopes and identification of their origin would be critical.1 The reclamation of radioactive metals can be aided by a fundamental understanding of their coordination chemistry to design better chelates and extractants.2 Additionally, identification of the elements present in radioactive materials can be facilitated by the introduction of “intentional forensic tags”. The development of such tags requires precise fundamental knowledge of the electronic structure of the elements involved.3
Curium (Cm) is an important element to study not only for its presence as a by-product in spent nuclear fuel,4 but also for its unique photophysical properties that could be exploited for nuclear forensics applications. Like its isoelectronic lanthanide counterpart, Gd(III), Cm(III) features a half-filled f-shell with seven unpaired electrons. Due to spin–orbit coupling, the energy gap between the ground state and excited states of Cm(III) (∼16
800 cm−1)5 is significantly smaller than that of Gd(III) (30
000 cm−1). This unique property results in strong red-orange luminescence centered around 595 nm, which makes Cm(III) particularly suitable for spectroscopic detection at nanomolar concentrations.6 The photophysical properties of Cm(III) are highly sensitive to the coordination environment and electronic structure of the metal ion. In this context, luminescence from Cm(III) has been exploited to determine the hydration number of the aqua ion.7 Both the ground and excited states of Cm(III) are highly sensitive to the coordination environment around the metal center. The nature of the surrounding atoms can cause these states to exhibit crystal field splitting, which can provide important information related to the electronic structure of this ion. The magnitude of this splitting is highly dependent on the nature of the coordinating ligand and the symmetry of the complex.8 Crystal field splitting of the ground and excited states of Cm(III) is often small and difficult to quantify in solution, especially at room temperature.9 As such, researchers have resorted to performing these analyses in the solid state or at cryogenic temperatures.10 Such measurements can be experimentally challenging, especially when involving rigorous engineering controls for safely handling highly radioactive samples in breakable vessels. Additionally, data obtained from cryogenic measurements may not be representative of the behavior of the element in environments relevant to nuclear applications.
Due to their expected chemical and electronic similarities, 5f-elements and 4f-elements should present similar spectroscopic behavior. Circularly polarized luminescence (CPL) spectroscopy is the measure of the preferential emission of right- or left-handed circularly polarized light. Because of the core-like nature of 4f orbitals, the splitting between energy levels of 4f-elements is larger through spin–orbit coupling than through crystal field splitting. In 4f-elements, each luminescent transition between spin–orbit coupling term levels is associated with a specific transition type, resulting in different relative CPL strengths.11 More importantly, CPL spectroscopy allows for better distinction of the individual components resulting from crystal field splitting, though these transitions' selection rules are not well understood. Since the strength of CPL spectroscopy to resolve fundamental electronic structures has been demonstrated with 4f-elements,12 we were motivated to use CPL spectroscopy as a tool to further understand the electronic structure of 5f-elements, specifically transplutonium elements. Since CPL typically requires relatively strong luminescence signals to provide resolved signals, curium was the 5f-element of choice to use as a proof-of-concept. We show below that by employing an appropriate ligand, well-resolved CPL spectra of a transplutonium element could be observed. More importantly, the quality of the data allowed for the deconvolution of the energy levels providing experimental mapping of the fundamental electronic structure of a Cm(III) coordination complex in aqueous solution at room temperature.
Results and discussion
Synthesis
We have recently shown that the use of N,N,N′,N′-tetrakis[(6-carboxypyridin-2-yl)methyl]-1,2-diaminocyclo-hexane (tpadac) (Fig. 1), a decadentate ligand containing a trans-1,2-diaminocyclohexane backbone and picolinate chelating groups, to generate complexes of trivalent lanthanides that were both highly luminescent in water and CPL active.13 The combination of having a well-characterized model system, strong luminescence, ability to generate CPL, and the potential for a strong ligand field prompted us to examine the coordination chemistry and photophysical properties of this chelator with curium.
 |
| Fig. 1 Synthesis of [Cm(S,S)-tpadac][K], the other enantiomer was synthesized analogously using (R,R)-tpadac. MOPS: 3-(N-morpholino)propanesulfonic acid. | |
To limit exposure to the highly radioactive curium isotope (Cm-248: t1/2 = 348
000 years, decaying by alpha decay and spontaneous fission), the synthetic procedure was adapted to work on a microgram scale and was designed to limit the number of hands-on operations. Briefly, a buffered solution of ligand was added to a solid residue of CmCl3·xH2O and the solution was allowed to equilibrate before being directly transferred to a cuvette for spectroscopic measurements (see ESI for details†). The procedure was validated using terbium as a surrogate and the spectroscopic data obtained were identical to those reported in the literature (see ESI for details†).13
Optical properties
The absorption spectrum of [Cm(S,S)-tpadac][K] is consistent with all [Ln(S,S)-tpadac][K] complexes, with a single absorption feature centered at 270 nm and attributed to the picolinate π–π* transition (Fig. 2).14 Upon excitation at 280 nm, a sharp luminescence feature was observed between 570 and 630 nm, consistent with picolinate-sensitized emission from the 6D7/2/6P7/2 → 8S7/2 transition of Cm(III).10a,15 Visual inspection of the luminescence spectrum clearly reveals the contribution of multiple overlapping features (see chiroptical analyses below). The excitation spectrum measured at the peak maximum (609 nm) is also consistent with what was previously observed with all [Ln(S,S)-tpadac][K] complexes.13 The luminescence lifetime of [Cm(S,S)-tpadac][K] was measured to be 0.723 ms and the decay was fitted to a monoexponential, consistent with the presence of a single emissive species in solution. The quantum yield, which was measured using an integrating sphere, was determined to be 13%. This is lower than that of the analogous terbium complex (47%) and slightly higher than the europium complex (6.5%), which is consistent with the expected positions of the emissive levels of Tb, Cm, and Eu (20
500, 17
100, and 17
300 cm−1, respectively)16 relative to the triplet state of the ligand (22
272 cm−1).17 Additionally, to examine the possibility of luminescence quenching by inner-sphere water molecules, we measured the lifetimes in various H2O/D2O mixtures to determine the number of coordinated water molecules (q) through the fit initially described by Kimura and Choppin.7d Our experiments suggest a q value of −0.2 ± 0.01 (with an equation error estimated at ±0.5 water molecules7d). These results are consistent with no bound water molecules (see ESI†). Overall, all the data collected through optical spectroscopy are consistent with a similar coordination environment around curium when compared with the lanthanides. We thus proceeded with chiroptical measurements.
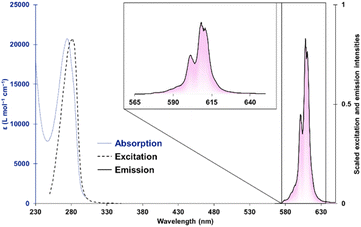 |
| Fig. 2 Absorption (dotted), excitation (dashed), emission (solid) spectra of [Cm(S,S)-tpadac][K] (4.7 μM) in 0.1 M MOPS buffer (pH 7). Insert: zoom in of the emission spectrum. MOPS: 3-(N-morpholino)propanesulfonic acid. | |
Chiroptical properties
Upon excitation at 280 nm, the [Cm(S,S)-tpadac][K] complex displayed circularly polarized luminescence (Fig. 3). Thanks to the relatively strong quantum yield of the complex, the CPL spectrum of [Cm(S,S)-tpadac][K] is well resolved and at least seven individual luminescent components can be clearly observed. This contrasts starkly with previously published curium CPL spectra, which are noisy and featureless.18 A maximum dissymmetry factor was observed at 609 nm at −0.07 although another luminescent contribution at 589 nm displays a close dissymmetry factor at −0.06. These values may be slightly larger than those of previously reported CPL-active curium complexes (|glum|∼0.01).18 However, it has been shown that CPL metrics can vary greatly if the measurements were not performed under identical conditions (different bandpass),12,19 it is thus safer to consider the metrics of [Cm(S,S)-tpadac][K] to be of the same order of magnitude (Table 1). The spectrum of [Cm(R,R)-tpadac][K] was the expected mirror-image. The CPL brightness (BCPL) was calculated to be 165 L mol−1 cm−1 using the simpler equation introduced in 2020,20 we also calculated the AnBCPL (83.5 L mol−1 cm−1) utilizing an equation we developed that accounts for the different sign contribution of the mJ states.21a
 |
| Fig. 3 CPL spectra of [Cm(S,S)-tpadac][K] (orange) and [Cm(R,R)-tpadac][K] (blue) in solution in 0.1 M MOPS buffer (4.7 μM). The total luminescence is traced in the background. Excitation: 280 nm, bandpass: 0.5 nm. MOPS: 3-(N-morpholino)propanesulfonic acid. | |
Table 1 Summarized photophysical data for [Cm(S,S)/(R,R)-tpadac][K]
Φ
|
τ (ms) |
Max glum |
B
CPL (L mol−1 cm−1) |
An
B
CPL
21 (L mol−1 cm−1) |
0.13 |
0.723 |
±0.07 |
165 |
83.5 |
With the availability of well-resolved luminescence and circularly polarized luminescence spectra, we hypothesized the data obtained was sufficient to resolve the electronic structure of the [Cm(S,S)-tpadac][K] complex at room temperature. For Cm(III), the luminescent transition arises from a mixed 6D7/2/6P7/2 excited state to a ground 8S7/2 state.22 In C2-symmetry, both levels can theoretically be split by crystal field into a maximum of four individual mJ states, yielding sixteen possible luminescent transitions.23 Using both the luminescence and CPL spectra (Fig. 4), we were able to estimate the position of those sixteen transitions (see ESI for details†). This allowed the experimental mapping of the individual mJ states of the excited state (split into 4 states at 16
390, 16
510, 16
650, and 17
331 cm−1) and ground state (split into 4 states at 0, 88, 140, and 210 cm−1). The magnitude of the splitting is significantly greater than that of the free ion in a LuPO4 lattice (∼1–20 cm−1)24 and highlights the strong effect of ligand chelation on the splitting of these states. The fitted luminescence spectrum, using a theoretical statistical Boltzmann population of the states, is in reasonable agreement with the experimental data (black bars in Fig. 4 and S8† for Lorentzian fit). By fitting the CPL spectrum (see Fig. S9†), we also determined the sign for each transition between crystal field splitting levels (see Table S1†). This information could prove crucial for a broader study of the selection rules between crystal field splitting levels of 5f-elements. Lower temperature luminescence and CPL measurement could provide better precision, however, those experiments are logistically challenging as described above.
 |
| Fig. 4 (Left) Fundamental electronic structure of [Cm(S,S)-tpadac][K]; (top right) emission spectrum overlayed with predicted transitions as vertical black bars (the intensity is representative of a statistical Boltzmann distribution); (bottom right) CPL spectrum overlayed with predicted transitions as vertical dashed lines. | |
Conclusions
By using a ligand designed to enable strong luminescence, circularly polarized luminescence, and strong crystal field splitting, we have mapped the electronic structure of both the excited and ground states of a molecular complex of Cm(III) at room temperature. This unprecedented achievement was only possible thanks to the combination of luminescence and circularly polarized luminescence studies. Our study demonstrates that the electronic structure of curium and likely other luminescent actinides can be established at room temperature using readily available spectroscopic techniques, thus providing a clear path towards a deeper fundamental understanding of these rare, but critical elements in environmentally relevant conditions. Additionally, the magnitude of the crystal field splitting observed is significant enough to hypothesize that thorough structure/function studies will allow us and others to correlate ligand field, covalency, and electronic structures in a more precise and quantitative manner.
Data availability
The data supporting this article have been included as part of the ESI.†
Author contributions
Conceptualization: JJW, RJA, GU; investigation: JJW, AP, JAA, RL, JNW, GU; data curation: JJW, AP, JNW, GU; writing – original draft: JJW, GU; writing – reviewing & editing: JJW, AP, JAA, JNW, RJA, GU; funding acquisition: GU, RJA.
Conflicts of interest
There are no conflicts to declare.
Acknowledgements
The work described here was supported by the U.S. Department of Energy (DOE), Office of Science, Office of Basic Energy Sciences, Chemical Sciences, Geosciences, and Biosciences Division, Heavy Element Chemistry Program at Lawrence Berkeley National Laboratory under contract DE-AC02-05CH11231. AP was supported by the DOE's National Nuclear Security Administration's Office of Defense Nuclear Nonproliferation Research and Development (NA-22) as part of the NextGen Nonproliferation Leadership Development Program. This work also made use of instrumentation and resources available at the Molecular Foundry, a user facility supported by the U.S. DOE, Office of Science, Office of Basic Energy Sciences under Contract No. DE-AC02-05CH11231.
Notes and references
-
(a) K. Maher, J. R. Bargar and G. E. Brown Jr, Environmental Speciation of Actinides, Inorg. Chem., 2013, 57, 3510–3532, DOI:10.1021/ic301686d;
(b) S. D. Reilly, G. M. Patton, J. Murillo, W. J. Oldham, J. L. Miller, S. P. Lamont, M. E. Sanborm, I. May, A. J. Gaunt and S. K. Hanson, Trace Actinide Signatures of a Bulk Neptunium Sample, Anal. Chem., 2023, 95, 9123–9129, DOI:10.1021/acs.analchem.3c01682;
(c) M. D. Straub, J. Arnold, J. Fessenden and J. L. Kiplinger, Recent Advances in Nuclear Forensic Chemistry, Anal. Chem., 2021, 93, 3–22, DOI:10.1021/acs.analchem.0c03571.
-
(a) L. S. Natrajan, Developments in the photophysics and photochemistry of actinide ions and their coordination compounds, Coord. Chem. Rev., 2012, 256, 1583–1603, DOI:10.1016/j.ccr.2012.03.029;
(b) G. J.-P. Deblonde, M. P. Kelley, J. Su, E. R. Batista, P. Yang, C. H. Booth and R. J. Abergel, Spectroscopic and Computational Characterization of Diethylenetriaminepentaacetic Acid/Transplutonium Chelates: Evidencing Heterogeneity in the Heavy Actinide(III) Series, Angew. Chem., Int. Ed., 2018, 57, 4521–4526, DOI:10.1002/anie.201709183;
(c) P. J. Panak and A. Geist, Complexation and Extraction of Trivalent Actinides and Lanthanides by Triazinylpyridine N-Donor Ligands, Chem. Rev., 2013, 113, 1199–1236, DOI:10.1021/cr3003399;
(d) M. P. Jensen, R. Chiarizia, I. A. Shkorb, J. S. Ulicki, B. D. Spindler, D. J. Murphy, M. Hossain, A. Roca-Sabio, C. Platas-Iglesias, A. de Blas and T. Rodriguez-Blas, Aqueous Complexes for Efficient Size-based Separation of Americium from Curium, Inorg. Chem., 2014, 53, 6003–6012, DOI:10.1021/ic500244p;
(e) M. P. Kelley, J. Su, M. Urban, M. Luckey, E. R. Batista, P. Yang and J. C. Shafer, On the Origin of Covalent Bonding in Heavy Actinides, J. Am. Chem. Soc., 2017, 139, 9901–9908, DOI:10.1021/jacs.7b03251.
- F. Menetrier, D. M. Taylor and A. Comte, The biokinetics and radiotoxicology of curium: a comparison with americium, Appl. Radiat. Isot., 2008, 66, 632–647, DOI:10.1016/j.apradiso.2007.12.002.
-
(a) M. Osaka, S.-I. Koyama, K. Morozumi, T. Namekawa and T. Mitsugashira, Analysis of Curium Isotopes in Mixed Oxide Fuel Irradiated in Fast Reactor, J. Nucl. Sci. Technol., 2012, 38, 912–914, DOI:10.1080/18811248.2001.9715116;
(b) C. Madic, B. Boullis, P. Baron, F. Testard, M. J. Hudson, J.-O. Liljenzin, B. Christiansen, M. Ferrando, A. Facchini, A. Geist, G. Modolo, A. G. Espartero and J. De Mendoza, Futuristic back-end of the nuclear fuel cycle with the partitioning of minor actinides, J. Alloys Compd., 2007, 445, 23–27, DOI:10.1016/j.jallcom.2007.05.051;
(c) K. P. McCann, M. A. Jones, E. A. Kyser, T. E. Smith and N. J. Bridges, Scaling Trivalent Actinide and Lanthanide Recovery by Diglycolamide Resin from Savannah River Site's Mark-18A Targets, Ind. Eng. Chem. Res., 2021, 60, 507–513, DOI:10.1021/acs.iecr.0c03897.
- W. T. Carnall, Energies and Intensities of Some Low-Lying Levels of Trivalent Curium, J. Chem. Phys., 1967, 47, 3081–3082, DOI:10.1063/1.1712341.
-
(a) N. M. Edelstein, R. Klenze, T. Fanghanel and S. Hubert, Optical properties of Cm(III) in crystals and solutions and their application to Cm(III) speciation, Coord. Chem. Rev., 2006, 250, 948–973, DOI:10.1016/j.ccr.2006.02.004;
(b) R. N. Collins, T. Saito, N. Aoyagi, T. E. Payne, T. Kimura and T. D. Waite, Applications of time-resolved laser fluorescence spectroscopy to the environmental biogeochemistry of actinides, J. Environ. Qual., 2011, 40, 731–741, DOI:10.2134/jeq2010.0166.
-
(a) T. Kimura, G. R. Choppin, Y. Kato and Z. Yoshida, Determination of the Hydration Number of Cm(III) in Various Aqueous Solutions, Radiochim. Acta, 1996, 72, 61–64, DOI:10.1524/ract.1996.72.2.61;
(b) P. Lindqvist-Reis, R. Klenze, G. Schubert and T. Fanghänel, Hydration of Cm3+ in Aqueous Solution from 20 to 200 °C. A Time-Resolved Laser Fluorescence Spectroscopy Study, J. Phys. Chem. B, 2005, 109, 3077–3083, DOI:10.1021/jp045516+;
(c) G. Tian, N. M. Edelstein and L. Rao, Spectroscopic Properties and Hydration of the Cm(III) Aqua Ion from 10 to 85 °C, J. Phys. Chem. A, 2011, 115, 1933–1938, DOI:10.1021/jp1116432;
(d) T. Kimura and G. R. Choppin, Luminescence study on determination of the hydration number of Cm(III), J. Alloys Compd., 1994, 213, 313–317, DOI:10.1016/0925-8388(94)90921-0.
-
(a) I. Colliard, J. R. I. Lee, C. A. Colla, H. E. Mason, A. M. Sawvel, M. Zavarin, M. Nyman and G. J.-P. Deblonde, Polyoxometalates as ligands to synthesize, isolate and characterize compounds of rare isotopes on the microgram scale, Nat. Chem., 2022, 14, 1357–1366, DOI:10.1038/s41557-022-01018-8;
(b) M. Sturzbecher-Hoehne, B. Kullgren, E. E. Jarvis, D. D. An and R. J. Abergel, Highly Luminescent and Stable Hydroxypyridinonate Complexes: A Step Towards New Curium Decontamination Strategies, Chem.–Eur. J., 2014, 20, 9962–9968, DOI:10.1002/chem.201402103;
(c) J. V. Beitz, Laser-induced Fluorescence Studies of Cm3+ Complexes in Solution, Radiochim. Acta, 1991, 52, 35–39, DOI:10.1524/ract.1991.5253.1.35;
(d) N. Huittinen, I. Jessat, F. Real, V. Vallet, S. Starke, M. Eibl and N. Jordan, Revisiting the Complexation of Cm(III) with Aqueous Phosphates: What Can We Learn from the Complex Structures Using Luminescence Spectroscopy and Ab Initio Simulations?, Inorg. Chem., 2021, 60, 10656–10673, DOI:10.1021/acs.inorgchem.1c01319;
(e) M. Sturzbecher-Hoehne, C. Goujon, G. J.-P. Deblonde, A. B. Mason and R. J. Abergel, Sensitizing Curium Luminescence through an Antenna Protein To Investigate Biological Actinide Transport Mechanisms, J. Am. Chem. Soc., 2013, 135, 2676–2683, DOI:10.1021/ja310957f;
(f) G. J.-P. Deblonde, J. A. Mattocks, H. Wang, E. M. Gale, A. B. Kersting, M. Zavarin and J. A. Cotruvo Jr, Characterization of Americium and Curium Complexes with the Protein Lanmodulin: A Potential Macromolecular Mechanism for Actinide Mobility in the Environment, J. Am. Chem. Soc., 2021, 143, 15769–15783, DOI:10.1021/jacs.1c07103;
(g) M. A. Denecke, A. Rossberg, P. J. Panak, M. Weigl, B. Schimmelpfennig and A. Geist, Characterization and Comparison of Cm(III) and Eu(III) Complexed with 2,6-Di(5,6-dipropyl-1,2,4-triazin-3-yl)pyridine Using EXAFS, TRFLS, and Quantum-Chemical Methods, Inorg. Chem., 2005, 44, 8418–8425, DOI:10.1021/ic0511726;
(h) A. Skerencak-Frech, M. Trumm, D. R. Frohlich and P. J. Panak, Coordination and Thermodynamics of Trivalent Curium with Malonate at Increased Temperatures: A Spectroscopic and Quantum Chemical Study, Inorg. Chem., 2017, 56, 10172–10180, DOI:10.1021/acs.inorgchem.7b00694;
(i) R. E. Sykora, Z. Assefa, R. G. Haire and T. E. Albrecht-Schmitt, Synthesis, Structure, and Spectroscopic Properties of Am(IO3)3 and the Photoluminescence Behavior of Cm(IO3)3, Inorg. Chem., 2005, 44, 5667–5676, DOI:10.1021/ic050386k.
-
(a)
N. M. Edelstein, J. D. Navratil and W. W. Schultz, Americium and Curium Chemistry and Technology, D Reidel Publishing Co, United States, 1985 CrossRef;
(b)
L. R. Morss, N. M. Edelstein, J. Fuger and J. J. Katz, Chemistry of Actinide and Transactinide Elements, 4th edn, 2010 Search PubMed.
-
(a) S. K. Cary, M. A. Silver, G. Liu, J. C. Wang, J. A. Bogart, J. T. Stritzinger, A. A. Arico, K. Hanson, E. J. Schelter and T. E. Albrecht-Schmitt, Spontaneous Partitioning of Californium from Curium: Curious Cases from the Crystallization of Curium Coordination Complexes, Inorg. Chem., 2015, 54, 11399–11404, DOI:10.1021/acs.inorgchem.5b02052;
(b) P. Lindqvist-Reis, C. Walther, R. Klenze and N. M. Edelstein, Optical Spectra and Crystal-Field Levels of [Cm(H2O)9]3+ Ions with C3h Symmetry in Isotypic Rare-Earth Triflate and Ethyl Sulfate Salts, J. Phys. Chem. C, 2009, 113, 449–458, DOI:10.1021/jp808491k;
(c) A. Ioussov and J. C. Krupa, Luminescence Properties and Stability Constants of Curium(III) Complexes with Lacunary Heteropolyanions PW11O397− and SiW11O398− in Nitric Acid Solutions, Radiochim. Acta, 1997, 78, 97–104, DOI:10.1524/ract.1997.78.special-issue.97;
(d) R. T. Brundage, R. L. Powell, J. V. Beitz and G. K. Liu, Measurement of homogeneous line widths and ground-state splittings of trivalent curium in fluoride glasses, J. Lumin., 1996, 69, 121–129, DOI:10.1016/0022-2313(96)00089-0;
(e) N. Huittinen, A. C. Scheinost, Y. Ji, P. M. Kowalski, Y. Arinicheva, A. Wilden, S. Neumeier and T. A Stumpf, Spectroscopic and Computational Study of Cm3+ Incorporation in Lanthanide Phosphate Rhabdophane (LnPO4·0.67H2O) and Monazite (LnPO4), Inorg. Chem., 2018, 57, 6252–6265, DOI:10.1021/acs.inorgchem.8b00095.
- F. S. Richardson, Selection rules for lanthanide optical activity, Inorg. Chem., 1980, 19, 2806–2812, DOI:10.1021/ic50211a063.
-
(a) A. Sickinger, B. Baguenard, A. Bensalah-Ledoux, Y. Guyot, L. Guy, F. Pointillart, O. Cador, M. Grasser, B. Le Guennic, F. Riobe, O. Maury and S. Guy, Impact of the experimental bandwidth on circularly polarized luminescence measurements of lanthanide complexes: the case of erbium(III), J. Mater. Chem. C, 2024, 12, 4253–4260, 10.1039/D3TC04717B;
(b) A. Sickinger, M. Grasser, B. Baguenard, A. Bensalah-Ledoux, L. Guy, A. T. Bui, Y. Guyot, V. Dorcet, F. Pointillart, O. Cador, S. Guy, O. Maury, B. Le Guennic and F. Riobe, Temperature-dependent NIR-CPL spectra of chiral Yb(III) complexes, Phys. Chem. Chem. Phys., 2024, 26, 15776–15783, 10.1039/D4CP01286K.
- J. A. Adewuyi, N. D. Schley and G. Ung, Synthesis of bright water-soluble circularly polarized luminescence emitters as potential sensors, Inorg. Chem. Front., 2022, 9, 1474–1480, 10.1039/D1QI01398J.
-
(a) N. Chatterton, Y. Bretonniere, J. Pecaut and M. Mazzanti, An Efficient Design for the Rigid Assembly of Four Bidentate Chromophores in Water-Stable Highly Luminescent Lanthanide Complexes, Angew. Chem., Int. Ed., 2005, 44, 7595–7598, DOI:10.1002/anie.200502231;
(b) A. Hu, I. Keresztes, S. N. MacMillan, Y. Yang, E. Ding, W. R. Zipfel, R. A. DiStasio Jr, J. W. Babich and J. J. Wilson, Oxyaapa: A Picolinate-Based Ligand with Five Oxygen Donors that Strongly Chelates Lanthanides, Inorg. Chem., 2020, 59, 5116–5132, DOI:10.1021/acs.inorgchem.0c00372;
(c) V. Jornet-Molla, C. Dreessen and F. M. Romero, Robust Lanthanoid Picolinate-Based Coordination Polymers for Luminescence and Sensing Applications, Inorg. Chem., 2021, 60, 10572–10584, DOI:10.1021/acs.inorgchem.1c01229;
(d) C. Guanci, G. Giovenzana, L. Lattuada, C. Platas-Iglesias and L. J. Charbonniere, AMPED: a new platform for picolinate based luminescent lanthanide chelates, Dalton Trans., 2015, 44, 7654–7661, 10.1039/C5DT00077G.
- S. K. Cary, M. Vasiliu, R. E. Baumbach, J. T. Stritzing, T. D. Green, K. Diefenbach, J. N. Cross, K. L. Knappenberger, G. Liu, M. A. Silver, A. E. DePrince, M. J. Polinski, S. M. Van Cleve, J. H. House, N. Kikugawa, A. Gallager, A. A. Arico, D. A. Dixon and T. E. Albrecht-Schmitt, Emergence of californium as the second transitional element in the actinide series, Nat. Commun., 2015, 6, 6827, DOI:10.1038/ncomms7827.
- C.-G. Ma, M. G. Brik, D.-X. Liu, B. Feng, Y. Tian and A. Suchocki, Energy level schemes of fN electronic configurations for the di-, tri-, and tetravalent lanthanides and actinides in a free state, J. Lumin., 2016, 170, 369–374, DOI:10.1016/j.jlumin.2015.07.053.
- A. G. Cosby, G. Quevedo and E. A. Boros, High-Throughput Method To Measure Relative Quantum Yield of Lanthanide Complexes for Bioimaging, Inorg. Chem., 2019, 58, 10611–10615, DOI:10.1021/acs.inorgchem.9b01786.
-
(a) G.-L. Law, C. M. Andolina, J. Xu, V. Luu, P. X. Rutkowski, G. Muller, D. K. Shuh, J. K. Gibson and K. N. Raymond, Circularly Polarized Luminescence of Curium: A New Characterization of the 5f Actinide Complexes, J. Am. Chem. Soc., 2012, 134, 15545–15549, DOI:10.1021/ja306354n;
(b) A. Peterson, J. A. Adewuyi, J. J. Woods, J. N. Wacker, W. W. Lukens, R. J. Abergel and G. Ung, Consolidated Curium Reprocessing Procedure Inspires Molecular Design for Sensitized Curium Circularly Polarized Luminescence, Inorg. Chem., 2024, 63, 19752–19785 CrossRef CAS PubMed.
- D. Schnable and G. Ung, Augmentation of NIR Circularly Polarized Luminescence Activity in Shibasaki-Type Lanthanide Complexes Supported by the Spirane Sphenol, Inorg. Chem., 2024, 63, 7378–7385, DOI:10.1021/acs.inorgchem.4c00417.
-
(a) Y. Nagata and T. Mori, Irreverent Nature of Dissymmetry Factor and Quantum Yield in Circularly Polarized Luminescence of Small Organic Molecules, Front. Chem., 2020, 8, 1–6, DOI:10.3389/fchem.2020.00448;
(b) L. Arrico, L. di Bari and F. Zinna, Quantifying the Overall Efficiency of Circularly Polarized Emitters, Chem.–Eur. J., 2021, 27, 2920–2934, DOI:10.1002/chem.202002791.
-
(a) B.-A. N. Willis, D. Schnable, N. D. Schley and G. Ung, Spinolate Lanthanide Complexes for High Circularly Polarized Luminescence Metrics in the Visible and Near-Infrared, J. Am. Chem. Soc., 2022, 144, 22421–22425, DOI:10.1021/jacs.2c10364;
(b) O. G. Willis, F. Zinna and L. Di Bari, NIR-Circularly Polarized Luminescence from Chiral Complexes of Lanthanides and d-Metals, Angew. Chem., Int. Ed., 2023, 62, e202302358, DOI:10.1002/anie.202302358.
- W. T. Carnall and K. Rajnak, Electronic energy level and intensity correlations in the spectra of the trivalent actinide aquo ions. II. Cm3+, J. Chem. Phys., 1975, 63, 3510–3514, DOI:10.1063/1.431789.
-
A. de Bettencourt-Dias, Introduction to Lanthanide Ion Luminescence, Luminescence of Lanthanide Ions in Coordination Compounds and Nanomaterials, John Wiley & Sons, 2014, DOI:10.1002/9781118682760.ch01.
- J. Sytsma, K. M. Murdoch, N. M. Edelstein, L. A. Boatner and M. M. Abraham, Spectroscopic studies and crystal-field analysis of Cm3+ and Gd3+ in LuPO4, Phys. Rev. B:Condens. Matter Mater. Phys., 1995, 52, 12668–12676, DOI:10.1103/PhysRevB.52.12668.
|
This journal is © The Royal Society of Chemistry 2025 |
Click here to see how this site uses Cookies. View our privacy policy here.