DOI:
10.1039/D4RA07531E
(Review Article)
RSC Adv., 2025,
15, 9375-9397
Three-dimensional bio-derived materials for biomedical applications: challenges and opportunities
Received
21st October 2024
, Accepted 28th February 2025
First published on 28th March 2025
Abstract
Three-dimensional (3D) bio-derived materials are emerging as a promising approach to enhance wound healing therapies. These innovative materials can be tailored to meet the specific needs of various wound types and patients, facilitating the controlled release of therapeutic agents such as growth factors and antibiotics, which promote cell growth and tissue regeneration. Despite their potential, significant challenges remain in achieving optimal biocompatibility, ensuring structural integrity, and maintaining precise release mechanisms. Additionally, issues such as scalability, cost-effectiveness, and regulatory compliance pose substantial barriers to widespread use. However, recent advances in materials science and interdisciplinary research offer new opportunities to overcome these challenges. This review provides a comprehensive analysis of the current state of 3D bio-derived materials in biomedical applications, highlighting the types of materials available, their advantages and limitations, and the progress made in their design and development. It also outlines new directions for future research aimed at bridging the gap between scientific discoveries and their practical applications in injury healing strategies. The findings of this review underscore the significant potential of 3D bio-derived materials in revolutionizing wound healing and advancing personalized therapeutic approaches.
1. Introduction
A wound refers to damage to biological tissues such as skin, mucous membranes, and organs, and can arise from various injuries.1 However, mitigating infections and further harm requires meticulous cleaning and dressing.2 In the year 1964, the National Academy of Sciences and the National Research Council introduced the Surgical Wound Classification (SWC) system to assess bacterial load in surgical fields. The Centers for Disease Control and Prevention (CDC) later refined this system, defining four classifications of wounds: Class 1, Class 2, Class 3, and Class 4. Class 1 wounds, such as those resulting from inguinal hernia repair or thyroidectomy, are considered clean wounds. They remain uninfected, reveal no signs of infection, and are usually closed. These wounds do not affect the respiratory, gastrointestinal, reproductive, or urinary tracts, though closed drainage may be required. Class 2 wounds are classified as clean-contaminated and involve minimal contamination. These wounds often involve controlled access to the respiratory, gastrointestinal, reproductive, or urinary tracts.3 Class 3 wounds, which include incisions connected to acute or non-purulent inflammation, result from breaches in sterile procedures or leaks from the gastrointestinal tract. Class 4 wounds, which are regarded as unclean or infected, typically arise from visible infections, severe purulence, or insufficient management of traumatic wounds. Tissue necrosis may be present in these wounds, frequently due to surgery or germs from organ perforations.4 Skin, being highly adaptive to environmental threats, exhibits sophisticated reparative processes, facilitating rapid healing.1 Despite its intrinsic healing abilities, compromised injury responses, often associated with advanced age or uncontrolled diabetes, can lead to chronic wounds.5
Chronic wounds require careful wound care to speed up the healing process. These wounds are frequently linked to venous illness, infection, diabetes, and metabolic abnormalities in the elderly. Wounds must be kept clean, moist, and shielded from infection and re-injury. Wounds may become chronic if the healing process is interfered with or does not proceed through its typical stages.6,7 The skin is a crucial barrier, shielding us from the physical environment, chemicals, and bacteria. When a wound breaches this barrier, the body initiates a complex healing process called wound healing.8,9 This process involves a coordinated effort by various cells and signaling molecules to repair and restore damaged tissue.9,10
1.1. Bio-derived materials role in different wound healing phases
Hemostasis, inflammation, proliferation, and remodeling are the four overlapping phases that make up the clearly defined stages that make up wound healing. Tables 1–4 provides a detailed analysis of the various biomaterial types that are utilized to support the four different phases in wound healing.
Table 1 Bio-derived materials used for hemostasis phases in wound healing
Name of the compound used in bio-derived materials |
Physical, chemical, and biological properties |
Applications |
Name of the commercially available products |
Medical device type |
Collagen/gelatin |
Adhesion, activation, and accumulation of platelets in wound sites, along with the start of coagulation cascades |
Hemorrhage is regulated by gelatin, a denatured form of collagen. The hemostatic effect of gelatin is ascribed to the biopolymer's swelling upon encountering blood, in addition to platelet initiation and aggregation, both of which augment blood coagulation |
Collagen: Avitene, Instat, Tachosil, Hilitene, and Gelatin: Hemopatch FloSeal and Gelfoam |
Sponge, hydrogel, films, nanofibrous mat11,12 |
Chitosan |
Biocompatible, biodegradable, and antimicrobial |
Platelet adhesion and blood coagulation |
Celox, TraumaStat, and HemCon |
Sponge, hydrogel, films, nanofibrous mat13–15 |
Cellulose |
Biodegradability, absorbability, and antibacterial |
Stimulate fibroblast proliferation |
Surgicel, Traumastem, Resorbs Cell |
Gauze, clay, sponge, hydrogel, films, nanofibrous mat16,17 |
Fibrin |
Biodegradability, absorbability |
Coagulation cascade and blood clot formation |
Tissel, Evicel, and Artiss, EVARREST, TachoSil |
Gauze, sponge, nanofibrous mat18–20 |
Hyaluronic acid |
Cytocompatibility, burst strength, and hemostatic potential in a rat hemorrhaging model |
Adhesion of lymphocytes to the endothelium, signal transduction, and gene expression |
Hyalosaf, Hyalomatrix, HylaSponge, Hylase Wound Gel, Laserskin, Connettivina, Bionect, Hyalofill |
Hydrogels, films/membranes, transdermal/matrix patches, bandages, subject gels, microspheres, nanoparticles, hydrocolloids, and nanofibrous mat21,22 |
Dextran |
Fast absorption, significant blood loss, low cytotoxicity, and robust tissue attachment |
Promotes quick wound closure, the start and aggregation of cells, and the aggregation of coagulation components at the wound site, all of which speed up the coagulation process |
Dex, Duoderm |
Sponge, nanofibrous mats and hydrogels23–25 |
Alginate |
Easy gelation using divalent cations, cheap cost, minimal toxicity, and biocompatibility |
The calcium ions in the alginate and the sodium ions in the blood undergo an ion exchange reaction when they come into contact. Blood coagulation activity is increased due to the activation of platelets. It possesses remarkable antimicrobial qualities. It removes released fluids and stops liquid from building up in an undesirable way at the injury site |
Sorbalgon and Sorbalgon Ag, Tegaderm Alginate, Kaltostat, CarboFlex, Biatain, Alginate, and Comfeel® Plus ulcer |
Hydrogels, fibers, granules, and tissue adhesives.26–28 |
Starch |
High water absorption, degradability, availability, affordability, and outstanding biocompatibility |
Uncontrolled and non-compressible severe hemorrhages are receiving quick hemostasis from them. By absorbing blood and helping platelets, red blood cells, and coagulating components to accumulate at the injury site and accelerate the blood coagulation cascade, starch's high specific surface area and porous structure contribute to the induction of hemostasis |
Quick clean |
Films, sponge29,30 |
Table 2 Bio-derived materials used for inflammation phases in wound healing
Name of the compound used in bio-derived materials |
Physical, chemical, and biological properties |
Properties |
Applications |
Medical device type |
Heparin |
Cell adhesion and proliferation, biodegradability, mechanical resistance, and biocompatibility |
Natural polymer – mucopolysaccharide or glycosaminoglycan |
Reduce inflammation, anticoagulant |
Polymeric, metal, and core/shell with biological material composites, hydrogels, and nanofibers31,32 |
Poly(lactic-co-glycolic acid) (PLGA) |
Superior mechanical qualities, appropriate biodegradability, well-cell compatibility, and improved cytocompatibility |
Glycolic and lactic acid monomers in a linear copolymer |
PLGA's superior processability, scaffolds with different pore diameters can be created. These scaffolds can be used in combination to regenerate damaged tissues and heal broken bones |
3D scaffolds, granules, fillers33,34 |
Polyglycolic acid and polylactic acid (PLA and PGA) |
Low availability, restricted molecular weight, and biodegradable |
Aliphatic polyester |
Agriculture feedstock serves as the basis for PLA raw materials, and the global agriculture sector will benefit from the rising demand for PLA resins |
Film, thermoforming, foaming, electrospinning fibers, internal sutures, scaffolds, and implant devices35,36 |
Polyglycolic acid (PGA) |
Degradation, highly crystalline structure, biodegradability, and environmentally safe properties |
Simplest linear aliphatic polyester |
Excellent knot strength that can be tied, and better handling features that enable it to be easily positioned where the surgeon desires |
Suture, stainless steel implants for bone fracture37 |
Chitosan |
Biocompatible, biodegradable, and antimicrobial |
Natural – polycationic polysaccharide |
Platelet adhesion |
Sponge, hydrogel, films, nanofibrous mat13–15 |
Collagen/gelatin |
Platelet adhesion, activation, and accumulation at the site of wounds, as well as the initiation of coagulation cascades |
Natural-polypeptide |
Hemorrhage is regulated by gelatin, a denatured form of collagen. The hemostatic effect of gelatin is ascribed to the biopolymer's swelling upon coming into contact with blood, in addition to platelet initiation and aggregation, both of which augment blood coagulation |
Sponge, hydrogel, films, nanofibrous mat11,12 |
Alginate |
Easy gelation using divalent cations, cheap cost, minimal toxicity, and biocompatibility |
Polysaccharides with a negative charge |
An ion exchange mechanism occurs when the sodium ions in the blood and the calcium ions in the alginate come into touch. Platelet activation has remarkable antimicrobial characteristics and increases blood coagulation activity. Holds and absorbs fluids that are produced. Liquids from accumulating at the injured site in an undesired manner |
Fibers, hydrogels, granules, and tissue adhesives26–28 |
Hyaluronic acid |
In a rat hemorrhage model, cytocompatibility, burst strength, and hemostatic potential |
Natural linear polysaccharide |
Gene expression, lymphocyte adhesion to the endothelium, and signal transduction |
Film/membrane, hydrogels, topical gels, hydrocolloids, foams, transdermal/matrix patches, microspheres, and nanoparticles.21,22 |
Table 3 Bio-derived materials used for proliferation phases in wound healing
Name of the compound used in bio-derived materials |
Physical, chemical, and biological properties |
Properties |
Applications |
Medical device type |
Polyethylene glycol (PEG) |
Low immunogenicity and biocompatibility |
Petroleum-derived polyether hydrophilic polymer |
Sealant and/or adhesive for controlling bleeding |
Hydrogels, films, nanofibrous mat, sponges38,39 |
Poly(lactic-co-glycolic acid) (PLGA) |
Superior mechanical qualities, appropriate biodegradability, well-cell compatibility, and improved cytocompatibility |
Glycolic and lactic acid monomers in a linear copolymer |
PLGA's superior processability, scaffolds with different pore diameters can be created. Combining these scaffolds can help with bone regeneration and repair and tissue restoration |
3D scaffolds, granules, fillers33,34 |
Polycaprolactone (PCL) |
Biocompatibility, non-immunogenicity, hydrophilicity, and flexibility |
Hydrophobic synthetic polymer |
A porous structure with a high surface-to-volume ratio promotes improved wound exudate absorption, cellular migration, proliferation, and adhesion |
Microspheres, hydrogel40,41 |
Gellan |
Non-toxic and biodegradable, inadequate mechanical strength biodegradable and pliable |
Linear negatively charged exopolysaccharide |
Protein carriers, biological signaling, directed bone-regeneration material, wound-healing cell adhesion, gene transfection, and biocides to prevent microbial infections |
Microspheres and poly-spheres42,43 |
Chitosan |
Antimicrobial, biocompatible, and biodegradable |
Natural – polycationic polysaccharide |
Blood coagulation |
Sponge, hydrogel, films, nanofibrous mat14–17 |
Collagen/gelatin |
Platelet adhesion, activation, and accumulation at the site of wounds, as well as the initiation of coagulation cascades |
Natural-polypeptide |
Collagen that has been denatured to create gelatin controls bleeding. Platelet initiation and aggregation, which promote blood coagulation, and the biopolymer's swelling upon blood contact are responsible for gelatin's hemostatic action |
Sponge, hydrogel, films, nanofibrous mat11,12 |
Silk fibroin |
Distinct mechanical characteristics, biocompatibility, adjustable rate of biodegradation, high nutrient permeability, and low inflammatory response |
Natural fibrous protein |
Attachment, proliferation, and migration of fibroblasts through the matrix |
Vascular graft, bone cartilage, corneal, films, mats, gels, sponges, beads, nanofibers, and porous foams44,45 |
Table 4 Bio-derived materials used for remodeling phase in wound healing
Name of the compound used in bio-derived materials |
Physical, chemical, and biological properties |
Properties |
Applications |
Medical device type |
Polyethylene glycol (PEG) |
Biocompatibility, and low immunogenicity |
Petroleum-derived polyether hydrophilic polymer |
Sealant and/or adhesive for controlling bleeding |
Hydrogels, films, nanofibrous mat, sponges38,39 |
Poly(lactic-co-glycolic acid) (PLGA) |
Well-cell compatibility, suitable biodegradability, excellent mechanical properties, and better cytocompatibility |
Glycolic and lactic acid monomers in a linear copolymer |
PLGA's superior processability, scaffolds with different pore diameters can be created. Combining these scaffolds can help with bone regeneration repair and tissue restoration |
3D scaffolds, granules, fillers33,34 |
Polycaprolactone (PCL) |
Biocompatibility, non-immunogenicity, hydrophilicity, and flexibility |
Hydrophobic synthetic polymer |
A porous structure with a high surface-to-volume ratio promotes improved wound exudate absorption, cellular migration, proliferation, and adhesion |
Microspheres, hydrogel40,41 |
Keratin |
Non-toxic and biodegradable, inadequate structural support and mechanical strength. Biodegradable and cellular patterns |
Natural cysteine-rich proteins |
The key element of the stratum corneum of the skin is crucial for stabilizing epithelial cells. Serve as structural scaffolding where the skin meets the epidermis |
Sponge, films, nanofibrous mat46,47 |
Collagen/gelatin |
Adhesion, activation, and accumulation of platelets in wound sites, along with the start of coagulation cascades |
Natural-polypeptide |
Hemorrhage is regulated by gelatin, a denatured form of collagen. The hemostatic effect of gelatin is ascribed to the biopolymer's swelling upon encountering blood, in addition to platelet initiation and aggregation, both of which augment blood coagulation |
Sponge, hydrogel, films, nanofibrous mat11,12 |
Silk fibroin |
Special mechanical qualities, low inflammatory reaction, biocompatibility, adjustable biodegradation rate, and high nutrient permeability |
Natural fibrous protein |
Non-toxic and biodegradable, inadequate structural support and mechanical strength. Biodegradable and cellular patterns |
Porous foams, films, mats, gels, sponges, beads, nanofibers, corneal grafts, and bone cartilage44,45 |
Polyvinyl alcohol (PVA) |
Antimicrobial, cell viability, and absence of negative consequences on human health |
Macromolecular organic polymer |
Wound repair capability |
Artificial joints, nanofibrous mats, contact lenses, stunts, and sponges48 |
Alginate |
The qualities of biocompatibility, bio-absorbability, and gel-forming ability |
Natural polysaccharide |
Its ability to absorb a lot of water draws fluids out of wounds, which inhibits the growth of bacteria |
Hydrogels, nanofibrous mat49 |
Casein |
Biocompatible, biodegradable, self-assembling properties |
Natural protein |
Enhanced epithelial proliferation and restitution |
Nanofibrous mat, films50 |
Curcumin |
Anti-inflammatory and antioxidant |
Natural polyphenolic |
To accelerate collagen deposition and granulation tissue development, promote fibroblast migration and proliferation. The last phases of wound healing significantly accelerate the process of wound re-epithelialization |
Films, hydrogels, nanofibrous mats, sponges, nanomaterials51,52 |
Honey |
Antimicrobial, antiviral, inflammatory-reducing, antibacterial, and antioxidant qualities |
Natural carbohydrate-rich syrup |
The process of physiological wound healing (debridement and granulation) is expedited. It lessens pain, debrides necrotic tissue, shields the site from infection, and encourages the growth of granulation tissue |
Films, hydrogels, nanofibrous mats, sponges, and nanomaterials53–55 |
In the initial stage of hemostasis, the primary focus is on stopping bleeding. Platelets aggregate to form a clot, effectively sealing the wound.8 The injury triggers the leakage of blood and lymphatic fluid, leading to the formation of the first reparative coagulum.56 Coagulation mechanisms are activated by intrinsic and extrinsic pathways.57 The intrinsic pathway is stimulated by platelets, while the extrinsic pathway is initiated by damaged tissue.3 Platelets adhere to injured endothelial cells and release platelet adenosine (ADP), which promotes further platelet aggregation and helps close the wound. Shortly after vasoconstriction, the blood vessels dilate, allowing more platelets and other blood cells to enter the wound site.58
Inflammation, the second stage of wound healing, starts when damaged blood vessels leak fluid, which is a combination of protein, salt, and water. This results in localized swelling. Both the immune system and hemorrhage control are strengthened by inflammation. The fluid buildup at the wound site draws cells capable of repair and healing, which go to the location to start the healing process.58 In the inflammatory phase, the wound site is cleared of bacteria, pathogens, and damaged cells.59 Because of the action of white blood cells, growth hormones, nutrients, and enzymes, this phase is marked by swelling, heat, discomfort, and redness.59 Prolonged or severe inflammation can be damaging, even though it is a natural and vital element of the healing process.60
The proliferative phase of wound healing involves the reconstruction of the wound with new tissue based on collagen and extracellular matrix.59 During this phase, the wound contracts, and new tissue is formed.60 A new network of blood vessels develops to ensure that the granulation tissue remains healthy and receives adequate oxygen and nutrients. Myofibroblasts help close the wound by holding the wound edges and exerting tension, similar to the contraction in smooth muscle cells.61 Granulation tissue, which is pink or red with an irregular texture in the early stages, should not bleed easily if healthy. Dark granulation tissue could indicate inadequate perfusion, ischemia, or damage. At the end of the proliferative phase, epithelial cells reappear in the wound.62 Epithelialization occurs most rapidly when wounds remain moist and hydrated.63 When occlusive or semi-occlusive dressings are applied within 48 hours following an injury, tissue hydration is maintained and epithelialization is encouraged.64
During the maturation phase, also known as remodeling, the wound healing process reaches its final stage. This phase is characterized by converting type III to type I collagen, which is critical for restoring tissue integrity and strength.65 Apoptosis, or programmed cell death, occurs to eliminate excess cells that were necessary during the previous phases but are no longer needed.66 Collagen deposited early in the proliferative phase, which is often disorganized and thick, undergoes significant restructuring and reorganization to improve tissue function and elasticity.67 Collagen fibers seem closer together and form a cross-link when they align along stress lines and reabsorb water.68 This cross-linking fortifies the skin surrounding the wound and lessens the thickness of the scar.59 Different Phases of Wound Healing treated using 3D bio-derived materials are illustrated in Fig. 1.
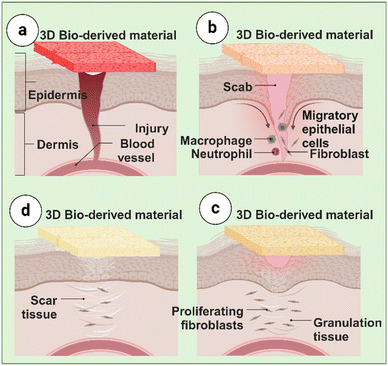 |
| Fig. 1 Different phases in wound healing – [a] bleeding and hemostasis, [b] inflammation, [c] proliferation, and [d] remodeling. Created in BioRender. Dhilip Kumar S. (2025) https://BioRender.com/q39h755. | |
2. Core principles of 3D bio-derived materials: design, properties, and applications
Biomaterials are essential to wound healing because they offer state-of-the-art methods for enhancing tissue regeneration, repair, and complete wound closure. Biomaterials function as scaffolding or matrices that resemble extracellular matrix (ECM) and offer essential guidance and support for cell migration, proliferation, and differentiation. Through increased endogenous cell recruitment, promotion of angiogenesis, collagen deposition, and epithelialization, they provide an environment conducive to tissue regeneration.69
Biomaterials can control the inflammatory response, pH, oxygen tension, and moisture content to affect the wound microenvironment. They can regulate the release of bioactive molecules (such as growth factors and cytokines) to promote healing and lower oxidative stress, inflammation, and infection. Therapeutics like growth factors, antibiotics, analgesics, and stem cells can be delivered locally and continuously with the help of biomaterials. Biomaterial carriers facilitate targeted administration, enhanced efficacy, less systemic side effects, and delayed release kinetics, hence improving treatment outcomes by encasing and safeguarding bioactive compounds.70
The vascularization of the wound bed—which is necessary for the delivery of nutrients, oxygenation, elimination of waste, and cell survival—is greatly aided by biomaterials. To promote angiogenesis and vasculogenesis and hasten wound healing and tissue regeneration, they can either replicate the nature of the extracellular matrix (ECM) or contain pro-angiogenic substances.71
Biomaterials can be used to treat a variety of wound kinds, sizes, and complexity because of their versatility in composition and design. Specific wound characteristics, such as chronicity, infection, exudate levels, and tissue loss, can be addressed to tailor treatment and enhance patient outcomes.57
Biomaterials can be utilized in performance with other therapeutic modalities like phototherapy, growth factor therapy, cell therapy, and physical stimuli like ultrasound and electrical stimulation to improve treatment outcomes more successfully. Combination techniques work better than monotherapies because they combine the several modalities' complementary mechanisms of action.72 Biomaterials show a considerable improvement in wound healing and tissue regeneration because they mimic the natural properties and composition of tissues to promote cell growth and proliferation.73 Among other things, they act as a scaffold to assist the growth of new tissue and guide cells to the injured area. These biocompatible materials, which offer transient structural support during tissue development, reduce the risk of rejection or inflammation in the body. Furthermore, biomaterials can be tailored to address specific wound conditions and individual patient needs.74
Overall, biomaterials have a variety of roles in wound healing, including as promoting vascularization, promoting tissue regeneration, modifying the wound microenvironment, delivering therapy specifically to target wounds, adapting to different types of wounds, and integrating with other therapeutic modalities. They are essential instruments in creating cutting-edge wound care treatments that enhance patient outcomes due to their adaptability and therapeutic potential.
2.1. Three-dimensional (3D) scaffold requirements
Numerous articles on scaffolds with different macro- and microstructures and biomaterial compositions have been published in the literature. In addition to sterility and economic feasibility, scaffold design considers mechanical factors (stiffness, elastic modulus, etc.), physicochemical factors (surface chemistry, porosity, biodegradability, etc.), and biological factors (cell adhesion, vascularization, biocompatibility, etc.). This review discusses the types, therapeutic uses, mechanisms of action, safety, and biocompatibility of 3D biomaterials for wound healing and skin tissue regeneration. The analysis highlights the emerging role of scaffolds in wound healing, tissue engineering, and regenerative medicine. It also highlights how the bioactivity and function of scaffolds can be manipulated by adjusting factors such as shape, size, strength, porosity, and degradation rate. The scaffold design has been developed to mimic the anatomical structure and biomechanics of the target tissue, allowing it to withstand external loads while maintaining the mechanical properties of the skin in the surrounding tissue. Tissue-specific mechanical properties, particularly stiffness, have been shown to influence mesenchymal stem cell differentiation.75
Scaffold designs sponges, meshes, and foams are essential for managing biodegradation, which is a critical aspect of tissue engineering. Degradation of biomaterials can happen across the material or just on the surface. In contrast to bulk deterioration, which destroys the interior structure, surface degradation maintains the bulk structure. The rate of deterioration needs to be adjusted to match the growth of the tissue. This can be done through physical, chemical, biological, or a combination of processes, all impacting the 3D scaffold's biocompatibility. For instance, the implant material is biologically altered by enzymatic digestion and cell-mediated breakdown, whereas incorporating numerous biodegradable components results in hydrolysis. Permanent (non-degradable) or semi-permanent scaffolds can be utilized in situations when total degradation is not required, such as articular cartilage restoration. When biocompatible scaffolds are inserted into the body, they shouldn't trigger any immunological, poisonous, or foreign body reactions. In addition, the scaffold's surface characteristics ought to be engineered to promote cell attachment, uniform dispersion, growth, and intercellular communication. Sustaining a porous or fibrous structure guarantees a high surface-to-volume ratio, hence fostering tissue growth and cell adhesion. When compared to smooth materials, nanostructured surfaces have a high surface energy, which enhances hydrophilicity and, consequently, protein and cell adhesion. The smaller grain size in metal and ceramic scaffolds enhances both mechanical strength and bone cell adhesion and proliferation.76
Consequently, a major factor in regulating cell behavior is the scaffold's topography and mechanical characteristics. Cells must be coaxed into taking on a certain in vivo morphology before they can be sown on 3D scaffolds. The creation of interconnected neurovascular networks between the mature tissue and its surroundings is also necessary for the regeneration process. While guaranteeing that nutrients, oxygen, and other soluble components are adequately given to the embedding cells and metabolic wastes are efficiently eliminated, the scaffold design must, on the one hand, promote the remodeling of blood vessels as the tissue matures. Conversely, to maintain homeostasis, nerve fibers—which are spatially intimately associated with cells that produce neuropeptide receptors—must develop in tandem with the new tissue. Generally speaking, the peripheral nerves are densely distributed across various tissues.77 Microsurgical integration of endogenous neurovascular bundles after scaffold implantation improves scaffold performance, considering the continued problem of regulating the development of different tissue types.78
The endogenous healing process can be aided and accelerated by many treatments, including stem cells, either alone or with natural or artificial scaffolds, especially in cases of significant or irreversible damage. Stem cells are widely used in therapy because they can differentiate when triggered in response to an illness and naturally maintain tissue homeostasis in healthy situations. These stem cells may originate from the lung, the umbilical cord, bone marrow, adipose tissue, muscular tissue, etc. harm. The tissue from which stem cells originated can be restored by tissue-specific stem cells. After an injury, several biological processes take place to heal the injured tissue, including the migration of stem cells and the production of chemicals and growth hormones. Several bioactive substances, including growth factors, peptides, genes, aptamers (single-chain oligonucleotides), antibodies, medications, and extracellular matrix (ECM) components, can mimic, improve, and regulate these activities. Biomaterials are bonded to the framework chemically or physically by hydrophobic interactions, hydrogen bonding, and electrostatic forces. The finely crafted scaffolds imitate natural signals and repair processes in this way, establishing an environment that is favorable to stem cell adhesion, proliferation, and differentiation and promoting tissue regeneration.77
3. Types of 3D bio-derived materials
Currently under investigation for use in wound healing applications are a variety of 3D biomaterials with special properties that are critical to the healing process. Variety is essential in satisfying the different needs of wounds, as seen by the variety of 3D biomaterials available for wound healing. Generally speaking, these biomaterials fall into two groups: synthetic and natural. Fig. 2 illustrates the overall biofabrication pathways for 3D tissue models, utilizing different cell sources to target organ-specific disease modeling and drug screening.
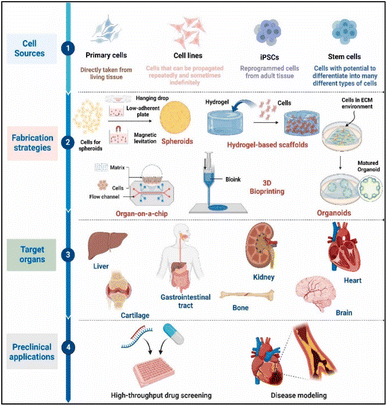 |
| Fig. 2 3D tissue models biofabrication. Reprinted from ref. 79 copyright 2024, Elsevier. | |
3.1. Natural biomaterials
In the realm of biomedical sciences, natural biomaterials are categorized based on their source and can be further divided into three groups: polysaccharides (cellulose, chitin/chitosan, alginate, agarose), proteins (collagen, gelatin, silk, fibrin, etc.), and glycosaminoglycans (hyaluronic acid, chondroitin sulfate, dermatan sulfate, heparan sulfate, keratan sulfate, etc.). Complex biomaterials derived from organs or cells have recently come into public view. Despite their varied origins, these biomaterials share the qualities of biocompatibility, biodegradability, and reconstructibility, which has piqued the curiosity of the scientific community. Applications for these biomaterials exist in tissue engineering and medicine. Consequently, we summarize and discuss the applications of the most widely used natural biomaterials in this section.80 Preparation of multifunctional nanocomposite hydrogel for wound healing applications are shown in Fig. 3.
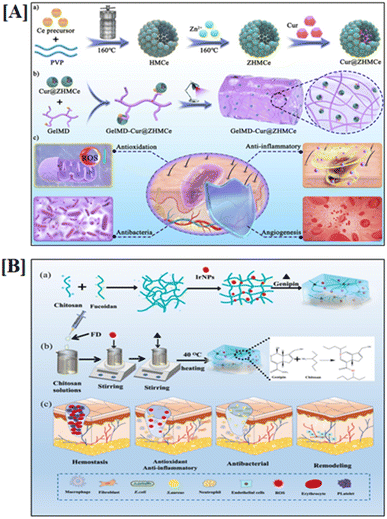 |
| Fig. 3 Preparation of multifunctional nanocomposite for wound healing applications. [A] Cur@ZHMCe-based nanomaterial, and [B] chitosan and fucoidan-based biomaterial. Reprinted from ref. 81 and 82 copyright 2024, Elsevier. | |
3.1.1. Protein-based biomaterials.
3.1.1.1. Collagen. Collagen is essential in biomaterials for wound healing because it closely mimics the natural scaffolding found in the body. It promotes cell adhesion, growth, and angiogenesis, accelerating the healing. However, due care must be taken regarding its origin (animal or human) and the potential for immune reactions. Collagen, the primary structural protein found in most animal tissues, is essential for preserving the extracellular matrix's (ECM) structural and biological integrity giving cells and tissues the support they need. Fibroblasts are the main biological source of collagen, which is released by them and regulates important processes such as cell shape, adhesion, migration, and differentiation. Collagen is found mostly in fibrous tissues like skin, tendons, and ligaments tissues that need strength and elasticity-like bone, cartilage, blood vessels, corneas, intestines, intervertebral discs, and dental dentin. Collagen makes up roughly 25–35% of the total protein content in the human body.83Collagen's potential applications are substantially expanded by its produced forms, which include films, fibers, sponges, beads, meshes, and interconnected steps. These forms also showcase collagen's adaptability.84 Numerous studies have shown the value of collagen as a scaffold for bone and cartilage.85–87 It can also be used for other applications, such as drug delivery systems, vascular grafts, bioprosthetic heart valves, ophthalmology, and nerve regeneration.84
Furthermore, 3D scaffolds for soft tissue engineering have cleverly integrated collagen matrices-containing microcapsules.85 In the field of liver tissue bioengineering, collagen-coated silicone scaffolds are now a crucial tool for maintaining stable three-dimensional hepatocyte cultures.86 Notably, using micro-engineered collagen scaffolds, Wang Y. et al. recently succeeded in reproducing the crypt architecture of human intestinal epithelium. These illustrations demonstrate collagen's enormous potential as a biomaterial.87
3.1.1.2. Gelatin. Gelatin is a denatured form of collagen that is hydrolyzed to create a biopolymer that is biocompatible, biodegradable, and fully absorbable. Its unique biological characteristics, exceptional water solubility, and affordable price have made it a convenient biomaterial with numerous benefits over its parent protein. Nanoparticles, microparticles, 3D scaffolds, electrospun nanofibers, and in situ gels are the formulations most frequently utilized in biological applications. The tissues of pigs, fish, and cows are used in these preparations.88Because of its versatility as a biomaterial, gelatin has been widely explored for biomedical applications. In stem cell research, it has been demonstrated that changes to the gelatin formulation can regulate the fate of stem cells, particularly in injectable cell-based treatments. Furthermore, gelatin-based delivery strategies have proven effective in delivering genes and siRNA by inducing gene silencing or promoting the expression of therapeutic proteins, respectively. With significant advancements made thus far and further discoveries anticipated, the likelihood of the rapid development and clinical application of products based on gelatin is increasing.89
3.1.1.3. Silk. A class of fibrous proteins known as silks, primarily composed of fibroin and sericin, are synthesized by the glandular epithelium of several insects, including silkworms, scorpions, spiders, mites, and other insects. Silk fibers have been used as sutures for decades, but they have now opened up new research avenues with promising results when it comes to experimenting with other formulations including gels, sponges, and films.90Silk has tremendous potential as a biomaterial, as evidenced by several studies conducted in the domains of orthopedics and cartilage tissue engineering. Despite their many applications—particularly in cartilage engineering silk fibers have attracted a lot of attention.91
Silk sericin, a glycoprotein derived from the silkworm Bombyx mori of the Bombycidae family,92 has garnered significant attention in recent years as a versatile biopolymer. Its intrinsic biological properties, including antimicrobial, antioxidant, antitumor, anti-aging, and moisture-retention capabilities, make it highly valuable across diverse industries such as food, cosmetics, textiles, and medicine. Beyond these attributes, sericin exhibits exceptional biocompatibility and biodegradability, promotes cell adhesion and proliferation, inhibits tyrosinase activity, and possesses anti-inflammatory properties, making it a promising candidate for wound healing and tissue regeneration.93
The three-dimensional (3D) biomaterial applications of sericin have further expanded its potential in regenerative medicine. When incorporated into 3D biomaterial systems such as scaffolds, hydrogels, and nanofiber matrices sericin enhances cellular interactions by providing a biomimetic microenvironment conducive to tissue repair. The nanoscale modification of sericin, achieved through nano structuring techniques, significantly increases its surface-area-to-volume ratio, thereby improving bioavailability, cellular adhesion, and polymer penetration. This nanosizing strategy, coupled with 3D biomaterial design, facilitates enhanced interaction with biological targets and enables a controlled, sustained release of bioactive compounds, which can be tailored to align with the dynamic phases of wound healing. By integrating nanotechnology with 3D biomaterial systems, sericin-based biomaterials offer a multifunctional platform for advanced wound healing applications, supporting accelerated tissue regeneration while ensuring optimal therapeutic efficacy.94
3.1.1.4. Fibrin. Fibrin, a non-globular protein important for blood clotting, is created when thrombin breaks down fibrinogen. Extensive research has taken advantage of fibrin's ability to repair wounds and act as a hemostatic plug, suggesting that fibrin may have uses in tissue engineering and medicine.91Three-dimensional fibrin gels have been used as a scaffold to facilitate cell migration and proliferation, among other uses. Ye Q. et al. have demonstrated the effectiveness of fibrin gels as a scaffold for cardiac tissue engineering, with well-regulated breakdown, good creation of tissue, and excellent cell seeding effects.95
Moreover, a unique biomaterial called as fibrin glue or fibrin sealant has been created by combining extremely high quantities of fibrinogen, thrombin, calcium, and Factor XIII. These days, this material is used to help with hemostasis in patients undergoing various surgeries. More specifically, autologous fibrin glue is a promising scaffold in regenerative maxillofacial surgery, according to Azizollah Khodakaram-Tafti et al.96
3.1.2. Polysaccharides-based biomaterials.
3.1.2.1. Cellulose. The cell walls of certain algae and green plants contain cellulose, the most prevalent naturally occurring polymer on Earth, which is created by bacteria.91 The physiological and pharmacological uses of cellulose are restricted by its challenging refining process, which makes it a less appealing substitute for natural biomaterials even though cellulose is abundant and renewable natural resources are readily available.97 Current research efforts are concentrated on optimizing the typically time-consuming procedures of depolymerizing cellulose and synthesizing its derivatives to fulfil the potential of cellulose as a biomaterial. The discovery of significant cellulose derivatives, such as carboxymethyl cellulose, cellulose nitrate, cellulose acetate, and cellulose xanthate, has been facilitated by developments in tissue engineering and medicine.91 For instance, several kinds of cellulose have been employed in cartilage98 and cellulose tissue engineering. Cardiac scaffolds have been made using cellulose acetate and regenerated cellulose based biomaterials.98,99 An intriguing and new use is the use of scaffolds composed of heparinized bacterial cellulose to encourage angiogenesis in tissue regeneration.82
3.1.2.2. Chitin/chitosan. Chitin is predominantly found in the exoskeletons of numerous insects and arthropods, and it is the second most prevalent natural polysaccharide after cellulose. Some chitin derivatives in food, medicinal, and environmental applications, include chitosan, glycol-chitin, and carboxymethyl chitin.100The science of tissue engineering has focused on chitosan (CS)-based polymers and their uses in recent years. Chitosan's intrinsic antibacterial qualities, porosity, and adaptability to different geometries that promote cell development and osteoconduction are the reasons for this interest.101 Moreover, chitosan has been shown to promote angiogenesis and accelerate wound-healing reactions by promoting the migration of inflammatory cells to the wound site and increasing collagen matrix deposition in open-skin wounds.102–104
3.1.2.3. Alginate. Brown seaweed, the source of alginate, produces hydrogels that resemble natural wound fluids and provide a moist environment that encourages cell migration. Its functional qualities are enhanced by its easy accessibility and biocompatibility, which facilitate its seamless integration with other materials. Alginate is a naturally occurring anionic polymer with remarkable biological merits, such as low toxicity, biocompatibility, and affordability. Its adaptability also extends to the creation of three-dimensional scaffolding materials, including hydrogels, foams, fibers, sponges, microspheres, and microcapsules.105 Because of its unique physicochemical characteristics, alginate is ideal for a variety of applications, especially in drug and cell delivery systems. Anionic alginates interact with multivalent inorganic cations through ionotropic gelation to form a hydrogel with hydrophilic polymer networks and three-dimensional cross-linked structures that may absorb huge volumes of water or biological fluids. Alginate is therefore one of the most important biomaterials in the production of hydrogels. Alginate hydrogels and the extracellular matrix (ECM) of biological tissues share structural similarities, which makes them highly advantageous for use in enhanced drug delivery systems and wound healing.106
3.1.2.4. Agarose. Agarose, the primary component of agar, is a naturally occurring polymer that is obtained from seaweed and red algae. It also has many of the positive attributes of alginate, including as biocompatibility and ease of manufacturing. Agarose and alginate were among the first materials to be utilized as hydrogels for cartilage tissue creation.107Biodegradability, biocompatibility, support for functional tissue growth, and adequate biomechanical properties to maximize tissue regeneration are some of the important requirements that biomaterials intended for tissue repair must meet.108,109 These materials are often used as hydrogels or porous scaffolds in cartilage tissue engineering. Although algae-derived agarose lacks the natural linkers that would allow it to interact directly with mammalian cells, making it somewhat inert, it nevertheless has several advantages.109 Agarose hydrogels provide a favorable environment for minimally invasive delivery, with the potential to form an injectable hydrogel/cell matrix, which is useful for applications involving tissue engineering.110
Agarose hydrogels have been thoroughly studied for their potential as scaffolds to facilitate cartilage regeneration and encourage chondrocyte growth.109,110 Numerous studies have demonstrated the superiority of agarose as an in vitro chondrocyte phenotypic maintainer, indicating its potential as a cartilage tissue engineering material. Despite these advantages, agarose hydrogels have disadvantages like weak biomechanical properties and challenging handling that have prevented widespread deployment. Furthermore, regenerative medicine is still very interested in the possibility of using agarose to promote the chondrogenic differentiation of adult stem cells, although this possibility has not yet been thoroughly investigated.111,112
3.1.3. Glycosaminoglycans. Hyaluronic acid (HA), chondroitin sulfate (CS), dermatan sulfate, heparan sulfate, and keratan sulfate are among the materials that comprise the glycosaminoglycans (GAGs) class of linear, unbranched polysaccharides. Repetitive disaccharide units set GAGs apart. Because of their crucial roles in the construction of the extracellular matrix (ECM) and cellular processes, HA and CS have emerged as the GAGs that are the subject of the most investigation in the fields of tissue engineering and regenerative medicine.91Proteins (such as collagen, gelatin, silk, and fibrin), polysaccharides (such as cellulose, chitin/chitosan, alginate, and agarose), or oligosaccharides (such as hyaluronic acid, chondroitin sulfate, dermatan sulfate, heparan sulfate, and keratan sulfate) are examples of natural biomaterials used in biomedical applications. More complex biomaterials have lately been developed, including matrices made from cells or organs that closely mimic the extracellular matrix seen in nature. Although these biomaterials vary widely, they share fundamental characteristics, like tissue remodeling support, biocompatibility, and biodegradability. These properties have spurred growing scientific interest in using these materials to further medical and tissue engineering technology.113
3.1.3.1. Hyaluronic acid (HA). Hyaluronic acid is special because of its ability to attract and retain moisture, which produces the perfect environment for cell growth. It also contains anti-inflammatory properties and is essential for tissue regeneration. This complex, which is extensively present in many connective tissues and body fluids, including synovial fluid and the vitreous humor of the eye, is composed of repeating disaccharide units of D-glucuronic acid and N-acetyl-D-glucosamine. Due to its exceptional biocompatibility and inherent chemical properties, including its solubility and reactive functional groups, it is an ideal material for tissue regeneration and medicine.91Biodegradable cartilage scaffolds using HA in tissue engineering, and HA–collagen hybrid scaffolds provide incredibly robust and freely permeable 3-D matrices that promote the formation of mammary stromal tissue in vitro.114,115 A hydrogel was found to have a neuroprotective effect on rats' spinal cords after they had a lacerating lesion to the spinal cord in another study by Kushchayev S. V. et al. (2016).116
3.1.3.2. Chondroitin sulfate. Chondroitin sulfate (CS) is the second most often utilized glycosaminoglycan in biomaterial applications. It is derived from repeating disaccharide units of D-glucuronic acid and N-acetyl galactosamine.117 A variety of extraction and purification methods can be used to get CS from cartilage sources such as shark, chicken, pig, cow, and skate. In biological contexts, CS plays a critical role in bone and cartilage metabolism and regulation. Moreover, it exhibits anti-inflammatory properties and facilitates accelerated bone mineralization.118
3.2. Extracellular matrix-derived biomaterials
3.2.1. Cell-derived matrices – decellularized extracellular matrix (dECM). The cells in these innovative materials were isolated from genuine tissues. It preserves essential components like as growth factors and adhesion molecules, which are essential for guiding the healing process and encouraging a speedier closure and better tissue quality. Recent developments in cell biology, regenerative medicine, and tissue engineering have been fueled by the development of decellularized extracellular matrix (dECM), which overcomes the limitations of traditional naturally obtained biomaterials. A cell-derived matrix (CDM) is an acellular complex comprising several fibrillar proteins that exist naturally, matrix macromolecules, and associated growth factors. Often, these matrices only offer a partial recapitulation of the composition and organization of natural ECM microenvironments. As ECM-derived materials, CDMs provide biological and mechanical support, promoting the synthesis of paracrine factors and tissue-specific differentiation in addition to cellular attachment, migration, and proliferation.85
3.3. Synthetic biomaterials
Polyesters' intrinsic biocompatibility and biodegradability make them a flexible class of polymers widely used in regenerative medicine applications. The reason for their biodegradability is that ester bonds can hydrolyze in aqueous solutions. Out of all the polyesters that are accessible, poly(lactic acid), poly(glycolic acid), and polycaprolactone (PCL) have become the most used types.
3.3.1. Poly(lactic acid) (PLA). The intrinsic chirality of its attached methyl groups, PLA displays a variety of isoforms. In regenerative medicine (RM) applications, the racemic DL-isoform (PDLLA) and the L-isoform (PLLA) are frequently used. PLA has a high strength (about 50–70 MPa) and tensile modulus (∼3 GPa), which makes it a popular fixative for bone implants.119 The molecular weight of PLA affects how quickly it degrades; polymers with a lower molecular weight degrade more quickly. Ester bond hydrolysis is the main mechanism of degradation, which results in a gradual reduction of molecular weight. However, in RM applications, the acidic breakdown products produced during degradation can present problems and even cause tissue damage. Furthermore, seeded cells in conventional tissue engineering applications using PLA scaffolds may be adversely affected by acidic by-products.85
3.3.2. Poly(glycolic) acid (PGA). Another polyester that has been extensively utilized for numerous RM applications is PGA. PGA is more hydrophilic than PLA, which makes it more prone to deterioration under watery conditions. Despite this, PGA has a tensile modulus that is roughly 7 GPa higher than PLA's. However, bulk deterioration could cause its mechanical characteristics to deteriorate with time.120
3.3.3. PCL (polycaprolactone). A semi-crystalline polyester that is widely used in various regenerative medicine (RM) applications is PCL. Due to its adaptability, copolymers with tunable properties can be easily copolymerized with a wide range of different polymers. Copolymer blends are particularly useful in drug delivery applications where control of the dissolution and subsequent release of the drug is important. Additionally, PCL and polyethylene oxide (PEO) have been copolymerized to create polymers with hydrophobic and hydrophilic microdomains.121
3.3.4. Polyurethanes. Synthetic polymers are incredibly versatile; they can be made to display characteristics like strength, flexibility, and controlled medication release, making them appropriate for a wide range of biomaterials. Additionally, by using nonaromatic di-isocyanates, toxicity issues related to the breakdown of aromatic diisocyanate-based polyurethanes are mitigated. PUs is employed in a variety of medical contexts, such as blood arteries (vascular grafts), breast implants, wound healing materials, and face reconstruction, because of their elastomeric nature and compatibility with blood.122 Fig. 4 shows the in vitro and in vivo biocompatibility of 3D biomaterials.
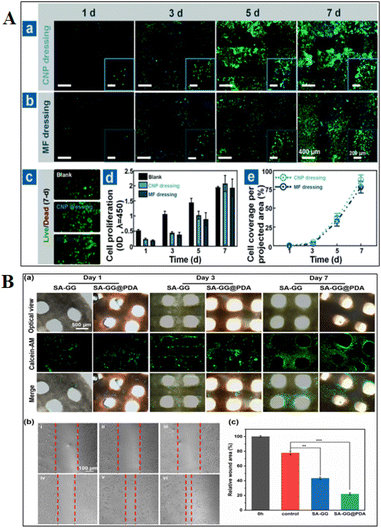 |
| Fig. 4 [A] and [B] In vitro biocompatibility of 3D biomaterials. Reprinted from ref. 123 and 124. | |
3.3.5. Composites. Combining synthetic and natural materials improves their special benefits. For example, the best scaffold is produced when the mechanical stability of a synthetic polymer is combined with the biological signals of collagen. A “composite” is the macroscopic combination of two or more materials with dissimilar compositions, morphologies, and general physical characteristics. Composites can frequently be precisely engineered to have characteristics that meet certain mechanical, chemical, or physical needs. As a result, throughout the previous forty years, composites have been used increasingly frequently in various types of industries, including aerospace, automotive, marine, and more. Their considerable influence is especially evident in several medical specialties, such as orthopedics, cranial bone repair, temporomandibular joint prostheses, cardiology, dental, oral, and maxillofacial surgery, and tissue engineering. Recent research and testing on a wide range of composite biomaterials for medical applications has resulted in the commercialization of some due to their benefits over conventional materials. Human tissues are intrinsically composite, consisting of constituents whose quantity, distribution, shape, and qualities together determine the tissue's ultimate behavior. Examples of these tissues include bones, tendons, skin, ligaments, and teeth. These biological tissues can be partially mimicked by man-made composites, which match their mechanical characteristics and enable damaged tissue to resume its mechanical activities.125
3.3.6. Thin films. Films are a specific type of bandage that may draw fluids and debris from the wound bed and absorb them when placed on the wound surface. Compared to other materials, thin films provide many advantages, including easier gas exchange, flexibility to allow injured tissue to move, and simple wound inspection (especially with transparent films). Moreover, films with advantageous swelling characteristics promote cell migration by creating a moist environment at the damage site. However, it is important to that films are more effective on superficial wounds due to their restricted ability to absorb large amounts of exudates.78
3.3.7. Gauze. An ideal hemostatic dressing should not cause adverse side effects, and it should stop severe bleeding quickly (within about 2 minutes) to avoid ischemia in large wounds. Many hemostatic solutions, such as powders, hydrogels, and sealants, still suffer from drawbacks after decades of research and development, including poor performance, short shelf life, unfavorable side effects, high cost, difficulty in use, or limited availability. Although they are still used, especially in underdeveloped countries such as India, traditional techniques such as manual compression with cotton gauze cannot stimulate or induce platelet activation or clot formation. Ideally, these gauze pads would contain an appropriate hemostatic agent to initiate the coagulation cascade once blood is absorbed from the wound.80 A particular type of dressing called a film can draw fluid and debris from the wound bed and absorb them when it is put on the wound surface. Compared to other materials, thin films offer a few advantages, including simple wound examination, simple gas exchange, and flexibility to allow injured tissue to move (especially with transparent films). Additionally, films that exhibit favorable swelling qualities encourage cell migration by bringing moisture to the site of the damage. It is crucial to remember that films work best on superficial wounds because of their restricted capacity to absorb a lot of exudates.126
3.3.8. Foams. Solid porous matrices with soft silicone that are primarily made of polyurethane.127 Patients won't experience any discomfort when using these sterile foams on wounds.128 For wound dressings, polyurethane has several benefits, including high absorbency, air permeability, softness, flexibility, and cost-effectiveness.129 Furthermore, the silicone membrane helps exudate pass through to the absorbent foam, keeps dressings in place, and shields the area from injury while being removed.127 On the other hand, irregularities in the thickness, density, tensile strength, and elongation of the freeze-dried foam may cause pain and skin maceration in the surrounding area of the incision. An additional possible drawback is the possibility of newly generated tissue penetrating the dressing during the effect of infrequent alterations, which can cause incisional damage upon dressing removal.127,128
3.3.9. Sponges. Due to their flexibility, porous materials, are known for the complex pore networks that make up their three-dimensional structure. These essential properties, such as porosity, increased surface area, and low density, are used to achieve various purposes, including ion exchange, energy storage, purification, and catalysis. They are ideal for adsorption, separation, and storage due to their ability to interact with foreign molecules through internal and surface channels. There has been interest in investigating the potential of three-dimensional porous materials as host systems for biomedical purposes.130These scaffolds act as guides to lead the formation of new tissue and substrates that support the maintenance of specialized functions without obstructing proliferation and encourage cell adhesion. They not only provide an environment that is favorable to cell growth, but they also imitate the natural biological environment. Biomedical science has advanced to the point that scaffolds are being employed as wound dressings.80
Sponge made by freeze-drying techniques has remarkable qualities, including shape flexibility, a significant swelling capacity, the ability to load anti-inflammatory drugs, and sustained release capabilities. Moreover, these sponges promote cell adhesion, proliferation, and extracellular matrix production, especially in chondrocyte-like cells, highlighting their enormous potential in biological domains.126
Sponge materials are essential to biomedical exploration because of their exceptional properties, which include large porosity, elastic deformation, capillary action, and the ability to create a 3D reaction environment that is favorable. Because their intricate porosity structure allows them to use their large surface area and internal networks for effective encapsulation and transport, they offer promising platforms for drug administration, tissue engineering, anti-infection therapies, and wound healing. Improved preparation techniques have been created to regulate the morphology of these sponge materials and increase the variety of medications they may hold, enhancing workflows and guaranteeing manufacturing uniformity.131
3.3.10. Hydrogels. The extracellular matrix (ECM) and hydrogels, which are networks of polymer chains swelled by water, are similar in that they allow the incorporation of bioactive materials such as growth factors and antimicrobial agents and create a moist environment ideal for wound healing.131 Hydrogels are characterized by their intricate three-dimensional structures created by hydrophilic polymers. They are also known for their excellent oxygen permeability and effective absorption of wound exudate. This distinctive architecture promotes cell migration and tissue regeneration by maintaining moisture at the wound interface and by offering mechanical support akin to that of tissue.132 Hydrogels are adaptable carriers for therapeutic items, including medications, cells, and nanoparticles because they may be strategically engineered to respond to certain stimuli, target drug delivery, and provide intelligent monitoring.133 Hydrogels that react to stimuli function as smart materials that release active ingredients when needed. Furthermore, hydrogels' incorporation of monitoring features enables them to continuously evaluate the wound microenvironment, providing important data for treatment planning.134
3.3.11. Electrospinning nanofibrous mats. Electrospun nanofibrous scaffolds accurately replicate the complex architecture of the extracellular matrix (ECM), providing a large surface area required for cell adhesion and growth. Additionally, the scaffolds make it easier to incorporate bioactive chemicals to improve wound healing. Since it requires applying a high-voltage electric field to spin ultrafine nanofibers from molten polymers or solutions, the word “electrospinning” refers to this intricate process. Using a series of exact processes, a charged droplet of liquid polymer is exposed to a high voltage and experiences electrostatic repulsion. The droplet elongates until it reaches a critical point known as a Taylor cone, which causes the ejection of a liquid stream. This repulsive force counteracts the surface tension.135
3.3.12. Bioactive glasses. Bioactive glasses (BG) have been an integral part of bone replacement and regeneration for decades.136 Their efficacy stems from their capacity to integrate and develop a robust link with the bone following implantation, instead of being covered with fibrous tissue or leaving scars.137 Furthermore, they facilitate bone regeneration, releasing ions that induce the regulation of specific genes and gradually degrade, allowing the regeneration process to proceed smoothly.138 In recent years, new BG compositions have been developed and used commercially, such as strontium-releasing BG for treating osteoporosis134 and fluoride-releasing BG for oral care. However, Bioglass 45S5
135 and BonAlive S53P4
137 are still popular BG compositions. The constant development of new scaffolds, composites, and BG compositions. These developments often rely on physiologically active ions to initiate processes that can be exploited to alter gene expression or function as direct therapeutic agents. A thorough comprehension of BG's mechanism of action is necessary for innovative treatments based on it.139
3.3.13. 3D printed scaffolds. The utilization of three-dimensional printing technology has shown great promise in accurately constructing scaffolds, yielding incredibly detailed biomimetic three-dimensional structures. The processes used to create 3D scaffolds involve layer-by-layer construction. These processes include stereolithography, direct 3D printing, fused deposition modeling, and selective laser sintering. Using these techniques, scaffolds ranging from millimeters to nanometers have been constructed with success. Terms like solid-state fabrication, additive manufacturing, and 3D printing have all become interchangeable in the last ten years.140The ability to create flexible scaffolds with intricate characteristics that resemble the extracellular matrix (ECM) and promote homogeneous cellular dispersion is only one of the many benefits of 3D printing. The printing technology still limits the availability of biomaterials with the necessary stability and desired qualities for 3D printed scaffolds. Additionally, the complexity of the designs increases the amount of time needed to construct scaffolds, particularly when manual labor is used rather than automated processes.141
The current limitations in tissue engineering could be greatly reduced with the help of continued study and advancements in 3D printing technology. Hybrid materials and the combination of printing techniques make it possible to create scaffolds that closely mimic the extracellular matrix (ECM). Unlike conventional techniques, 3D printing offers the precision and flexibility required to produce scaffolds that faithfully capture the intricate architecture of the ECM. Eventually, these scaffolds enhance tissue formation and regeneration by creating an environment that is conducive to cellular processes such as adhesion, proliferation, spatial distribution, and differentiation.142 Fig. 3A and B, respectively, show how to make a multifunctional nanocomposite hydrogel and explain how it aids in wound healing and the preparation procedure and crosslinking mechanism of biomaterials.
3.3.14. Nanotubes and nanorods. An important advancement in nanotechnology, nanotubes and nanorods are characterized by their unique one-dimensional forms and possess extraordinary mechanical, electrical, and optical properties. Due to their enormous potential, these nanomaterials, which vary from metal oxide nanorods to carbon-based nanotubes, have garnered a lot of attention in a few industries, including electronics, energy storage, drug delivery, and catalysis. They are necessary for the development of novel nanoscale materials and technologies due to their large surface area, exceptional strength-to-weight ratio, and programmable characteristics.143The exceptional mechanical strength, low density, flexibility, and high surface area of carbon nanotubes (CNTs) have led to extensive research into their use as reinforcement fillers in polymer matrices. As a result, better functional characteristics have been achieved in nanocomposites.144 Carbon nanotubes (CNTs) have great promise for biomedical applications, particularly in orthopedics, because carbon is a basic constituent in biomolecules and is biocompatible. Because of its capacity to improve the adhesion and proliferation of important cell types like myoblasts, neurons, and osteoblasts, CNT-based composites are becoming more and more popular for bone regeneration. Single-walled carbon nanotubes (SWCNTs) and multi-walled carbon nanotubes (MWCNTs) are the two types of carbon nanotubes (CNTs). The focus of recent research has been on CNT/polymer composites for use in biomedicine. MWCNTs produced by chemical vapor deposition (CVD) are now economically feasible due to their low cost.145
Alternatively, because of their anisotropic form, which gives them unique plasmonic features and improved colloidal stability, nanorods are an excellent choice for drug administration, cancer detection and therapy, biomedical imaging, and biosensing.146–148 Gold nanorods, or AuNRs, have demonstrated a great lot of potential. According to a study by Xiao et al., AuNRs combined with doxorubicin and a targeted ligand are effective (2012),147 creating a multipurpose nanoplatform for targeted imaging and medication delivery in cancer cells. Because of their rod-shaped structure and targeting ligands, which enhance cellular absorption.149,150
Nanotubes and nanorods continue to exhibit great promise in the rapidly changing field of biomedical research, especially in tissue engineering and regenerative medicine. These nanostructures can be made from a variety of materials, including metals, metal oxides, polymers, and carbon-based materials like carbon nanotubes. They typically have cylindrical or rod-like geometries with diameters between a few and many tens of nanometers. Because so many different materials were utilized in their development, they have a wide spectrum of mechanical, electrical, and chemical qualities that make them perfect for use in biomedical applications. The primary objectives of biomaterials research, particularly concerning bone implants, are to attain mechanical strength and biocompatibility that are sufficient to endure the stresses exerted on them by the human body. In orthopedic applications, common materials include metals, polymer composites, and alloys, all of which are integral to achieving the necessary functional outcomes in biomedical devices.151
The high aspect ratio and anisotropic structure of nanotubes and nanorods make them naturally one-dimensional (1D) nanomaterials. Nonetheless, their incorporation into three-dimensional (3D) biomaterial systems, like hydrogels, scaffolds, or composite matrices, improves mechanical characteristics, bioactivity, and structural integrity, which promotes cellular adhesion, proliferation, and differentiation. For example, it has been demonstrated that adding carbon nanotubes to 3D-printed scaffolds increases their mechanical strength and facilitates bone tissue engineering applications.152
In specific cases, self-assembled or aggregated nanotube and nanorod networks can exhibit 3D structural characteristics, making them functionally relevant for tissue engineering and regenerative medicine applications. Research has demonstrated that surface-modified carbon nanotubes can promote osteoblast adhesion and differentiation, highlighting their potential in bone tissue engineering.153
While they are not intrinsically 3D biomaterials, their incorporation into macroscopic biomaterial architectures enables them to contribute significantly to 3D biomedical applications. Advancements in nanotechnology and fabrication techniques have facilitated the development of complex 3D nanostructures, such as peptide hydrogels and graphene-based materials, which are utilized in tissue engineering, cancer therapy, and regenerative medicine.154
4. Advancements of bio-derived materials in wound healing
Wound healing can be greatly impacted by some variables, including infection, malnourishment, and underlying medical conditions.155 To address these issues, several treatment alternatives have been developed, including dressings,156 debridement,157 antibiotics,158 growth factor therapy, negative pressure wound therapy, and hyperbaric oxygen therapy.159,160
Resolving wounds is still a major global health concern that affects millions of people each year in all age groups and demographics. These difficulties cover a broad spectrum of wounds, both acute and chronic, such as burns, pressure injuries, diabetic ulcers, and surgical wounds. Healthcare systems bear a significant financial burden in managing and caring for these wounds, which necessitate substantial resources including medical supplies, expert care, prescription drugs, and rehabilitation services.161
Wound healing difficulties have a significant negative influence on patients' quality of life in addition to their financial implications. They may result in excruciating pain, restricted movement, functional deficits, and psychological discomfort. Long-term consequences from chronic wounds might include disruptions to social connections and everyday activities. In addition to making these issues worse, delayed healing raises the possibility of consequences like infections, tissue necrosis, osteomyelitis (bone infections), amputations, and, in the worst situations, even death. Managing complicated wounds presents several challenges for healthcare professionals. These consist of a wide range of patient reactions to therapy, challenges in reaching the best possible healing results, and a significant chance of wound recurrence. Patients' care is made more difficult by the uneven effectiveness of wound healing interventions, which puts them at risk for long-term issues.76 A significant challenge in wound care is the disparity in access to quality treatment. Receiving quality treatment can be difficult for vulnerable groups, such as members of racial and ethnic minorities, the elderly, low-income people, and those who live in remote areas. These disparities are driven by socioeconomic status, healthcare infrastructure, health literacy, cultural beliefs, and geographical limitations. Advancements in wound healing strategies aim to address these challenges through innovative approaches and targeted interventions. This includes reducing healthcare inequities by improving access to high-quality care, implementing early intervention techniques, and developing comprehensive management plans. Emerging technologies in wound care, such as bioengineered materials, 3D biomaterials, and advanced therapeutics, hold promise for optimizing healing outcomes. By integrating these advancements, we can significantly reduce the overall socioeconomic burden of wound care, improve patient outcomes, and enhance the efficiency of healthcare systems.121
4.1. Importance of effective wound healing
Healing from wounds is important for everyone, but it's critical for the elderly and individuals with long-term conditions like diabetes and peripheral vascular disease. These individuals are more likely to get wounds, and they frequently have difficulties getting them healed.159 The wound needs to be kept clean, covered, and moist to encourage healing and avoid infection for healing to proceed successfully.162 Maintaining sufficient blood flow and minimizing pressure on the incision aid in the prevention of additional tissue damage.163 Swelling, inflammation, and slowed recovery are all possible outcomes of infections. In more serious situations, infections can travel to the bloodstream and result in potentially fatal sepsis.164 Restoring functions to the injured area depends on effective wound healing. For example, proper healing will support the muscle's recovery if a wound injures a muscle.84 The study performed using bio-derived materials for in vitro and in vivo wound healing applications are shown in Fig. 4 and 5, respectively.
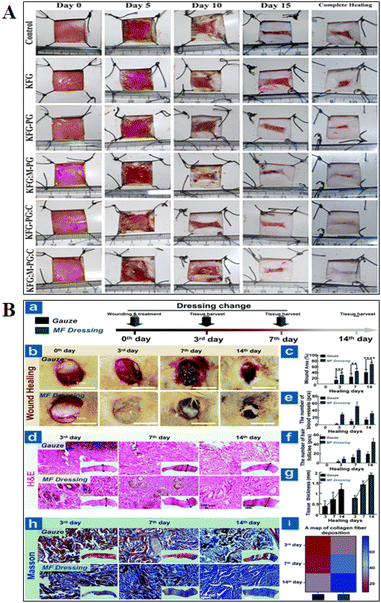 |
| Fig. 5 [A] and [B] In vivo biocompatibility of 3D biomaterials. Reprinted from ref. 47 and 123. | |
5. Three-dimensional bio-derived materials wound healing advantages, disadvantages and challenges
In many biological applications, three-dimensional (3D) biomaterials have unique advantages, disadvantages, and challenges. Understanding both the benefits and drawbacks of these technologies is essential to maximizing their usage in tissue engineering, regenerative medicine, and other medical fields.
5.1. Advantages of 3D bio-derived materials
Cells can adhere, multiply, and regenerate tissue because 3D biomaterials provide an extracellular matrix (ECM)-like structure. These materials allow for more specialized therapy and better results because they may be tailored to each patient's specific demands.122 Certain 3D biomaterials can precisely control the flow of drugs, growth hormones, or other bioactive compounds to speed up the healing process.165 The adoption of biodegradable 3D biomaterials reduces patient discomfort and risk of complications by eliminating the need for surgery. Bioprinting and 3D printing enable more precise construction of intricate structures.166
5.2. Disadvantages of 3D bio-derived materials
Three-dimensional (3D) biomaterials have great potential for wound healing, but some drawbacks may reduce their efficacy. These materials can be difficult to obtain due to the expensive and technically demanding sophisticated manufacturing processes that go into their creation. Biocompatibility problems can also cause negative reactions or inflammation, and mismatched degradation rates can provide insufficient support and create scar tissue. Certain biomaterials do not have enough mechanical strength, and their intricate structures may make it challenging for cells to transport nutrients and oxygen, both of which are necessary for survival. Furthermore, if improperly maintained, the porous structure of 3D biomaterials raises the risk of infection. Regulatory obstacles, patient variability, and the high expense of new production techniques further impede their therapeutic utilization. The goal of achieving optimal integration with host tissues remains a significant challenge that will influence the overall efficacy of these novel materials in promoting healing.167–169
5.3. Challenges of 3D bio-derived materials
The predominance of problems with inflammatory reactions, infection management, safety and regulatory concerns, clinical translation, trauma, and pathophysiological sequelae has made skin wounds a major challenge in modern medicine.34 Hemostasis, inflammation, proliferation, and extracellular matrix remodeling are all highly controlled molecular mechanisms during normal wound healing.170 Three-dimensional (3D) biomaterials provide very effective and customized therapies.171 However, before widespread clinical acceptance is accomplished, a few challenges need to be resolved. To overcome these obstacles, scientists and medical professionals are putting a lot of effort into developing breakthroughs in materials science, bioengineering, and medical technology. These challenges should become easier to overcome as our knowledge of 3D biomaterials and their uses expands, bringing us one step closer to realizing the potential of these materials for tissue regeneration and wound healing.
One of the hardest problems is developing biomaterials that are both biocompatible and biodegradable. Ensuring biodegradability to avoid the necessity for surgical removal once the wound has completely healed and biocompatibility to avoid negative reactions in the body.172 The achievement of exact 3D printing of biomaterials, which necessitates a high degree of accuracy and precision, is another barrier. To maximize the therapeutic impact of the biomaterial, it must be precisely tailored to meet the unique wound requirements of each patient. To guarantee that these materials are available to patients and healthcare professionals, as well as to encourage wider usage and benefits throughout the healthcare spectrum, it is imperative to design biomaterials that are both affordable and easily producible.173
Ensuring 3D biomaterials are completely biocompatible and do not trigger unfavorable immune reactions is a big task. It can be difficult to achieve mechanical properties that closely resemble those of the target tissue. It is still difficult to promote blood vessel growth in 3D biomaterials to guarantee adequate nutrient and oxygen delivery. Retaining structural integrity over time is important, particularly in load-bearing applications.174 Some 3D biomaterial fabrication methods are costly, and increasing production volume might be difficult. It can take a lot of effort and money to make sure that 3D biomaterials are thoroughly studied for safety and efficacy and that they adhere to legal requirements. It might be challenging to integrate seamlessly into host tissues since immunological responses and tissue rejection can happen. The design and creation of 3D biomaterials with the appropriate structures and properties can be challenging and time-consuming. The pace of biodegradation and tissue regrowth can be difficult to balance since biomaterials might degrade too quickly or too slowly. The use of human cells and tissues in bioprinting and tissue engineering may raise ethical and legal concerns.175
For effective clinical and commercial applications, the fabrication of 3D biomaterials poses several complex obstacles. Since material behavior and process variability can affect the product, achieving high precision and reproducibility is one of the manufacturing problems. Furthermore, the limits of existing technology make it challenging to fabricate intricate designs that replicate real tissues. Additionally, many biomaterials need post-processing procedures like surface changes or crosslinking, which raise production costs and complexity.176
Since the procurement of biomaterials frequently depends on non-renewable or animal-derived sources, which raises ethical and environmental concerns, sustainability is another crucial issue. Effective management of industrial waste is also necessary to reduce environmental impact, and energy-intensive manufacturing techniques like 3D printing provide more sustainability issues.177
Additional challenges arise when production is scaled up from laboratory research to industrial manufacturing. Due to equipment, raw material needs, and stringent quality control procedures, large-scale production is frequently costly. For medical applications, batch-to-batch consistency is essential, yet it is still a major challenge. Additionally, because many of the fabrication procedures used today are sluggish and inefficient, manufacturing speed needs to be increased to meet commercial demands.178
Lastly, two major issues in the creation of 3D biomaterials are still biocompatibility and regulatory barriers. For biomaterials to be successful in medical applications, they must not cause immunological rejection or unfavorable biological reactions. Another difficulty is long-term stability, since the materials need to retain their biological and mechanical characteristics for prolonged periods of time inside the body. Additionally, the lengthy and extremely strict regulatory approval process for medical biomaterials delays commercialization and raises expenses. Multidisciplinary cooperation, developments in material science, and enhancements in manufacturing technology are necessary to meet these problems.179
Despite these challenges, many of them are being addressed by continuing research and developments in materials science and biomedical engineering, increasing the usefulness of 3D biomaterials in the medical industry. It is anticipated that 3D biomaterials will help tissue repair and regeneration increasingly more effectively and versatilely as knowledge and technology grow.180 Advanced techniques including 3D printing, electrospinning, and bioprinting are needed to develop 3D biomaterials with complex architectures and qualities that match genuine tissues and organs while maintaining scalability and repeatability.181 It is imperative to guarantee the compatibility of 3D biomaterials with host tissues, taking into account the non-toxic components and additives utilized during their manufacturing.182 The challenge is to stabilize 3D biomaterials while promoting tissue regeneration by carefully releasing bioactive molecules (such cytokines, growth factors, or other bioactive agents). It can be challenging to discover mechanical qualities in biomaterials that are compatible with the characteristics of the target tissue across a range of tissue types and wound locations, such as those resulting from balancing strength and flexibility. The process of forming functional blood vessels inside the tissue, which is required to give oxygen and nutrients to tissues that are renovated, is challenging to do in three-dimensional biomaterials that promote angiogenesis. Promoting tissue regeneration while reducing the immune responses and inflammation caused by 3D biomaterials is crucial. Controlled degradation rates that align with the tissue's healing schedule are critical for 3D biomaterials to maintain stability over time, particularly in the case of long-term implants or chronic wound healing. It can be difficult and expensive to tailor 3D biomaterials to each patient's specific demands, especially when it comes to tailored treatment.
6. Opportunities and innovations
Applications for three-dimensional (3D) biomaterials in wound healing are becoming more and more common. These biomaterials can function as a scaffold for cell proliferation and rebuild tissue (ECM) by emulating the natural extracellular matrix. The creation of extremely intricate and patient-specific biomaterials for tissue remodeling has been made possible by developments in 3D printing and tissue engineering. Additive manufacturing, or 3D printing, is a quickly developing technology that has the power to dramatically change the biomaterials sector. The development of 3D printing is intended to displace conventional machining techniques. Layer by layer, intricate 3D structures may be constructed with 3D printing, precisely controlling the material's composition and cutting down on material waste.183 Scaffolds, patient-specific implants, and more equipment for tissue engineering and regenerative medicine might all be made using this 3D technology. Tissue pictures from frequently used medical imaging techniques, such as computed tomography (CT) and magnetic resonance imaging (MRI), can be utilized to create building blocks. This cannot be accomplished with traditional production methods.184 Dental molds, craniofacial implants, prosthetic parts, implants, and crowns are a few of the more modern medical applications of 3D printing. Other applications include organ printing, surgical models, scaffolds for tissue regeneration, including skin and bone, and tissue models for drug discovery.185–187 Fig. 6 illustrates the strengths and weaknesses of various biofabrication technologies used in constructing different types of 3D tissue models.
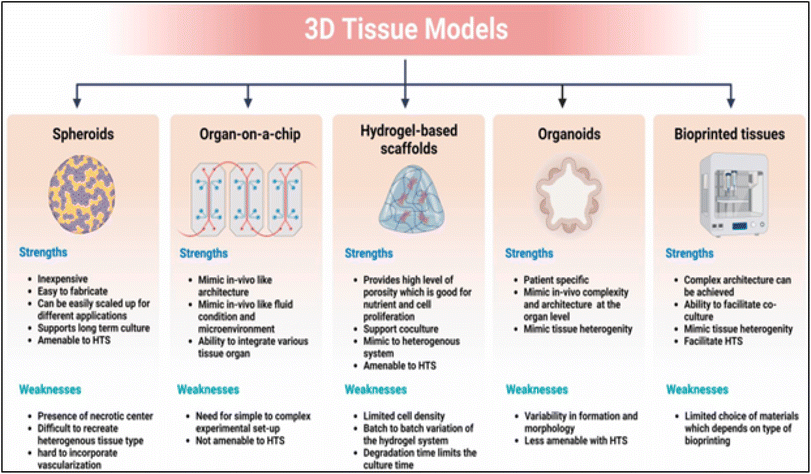 |
| Fig. 6 Biofabrication technology's strength and weakness for building 3D tissue models. Reprinted from ref. 79, copyright 2023, Elsevier. | |
The state-of-the-art in regenerative medicine and healthcare research and development is focused on customized wound healing and combination therapies based on novel biomaterials and technology. Customizing therapy regimens for each patient according to their genetic profile, medical background, and unique wound characteristics is known as personalized wound healing. The identification of genetic markers impacting wound healing is made possible by advancements in genetics and bioinformatics, which empower healthcare providers to provide individualized therapies.188
Modern biomaterials now come with sophisticated smart dressings that can track the advancement of wound healing in real time. By integrating sensors or nanotechnology elements, these dressings can identify signs like infection, inflammation, or pH variations, facilitating prompt management.189 Combination treatments and multimodal strategies are becoming more and more common for wound healing. Tissue regeneration can be improved by combining growth hormones, stem cells, or other biological agents with biomaterials. Stem cells within a three-dimensional biomaterial scaffold, for instance, can aid in tissue regeneration and enhance wound healing.190
Nanotechnology plays a major role in the therapy of personalized wounds. The direct administration of medications or growth factors to the wound site via nanoparticles enables targeted therapy. Through modification of biomaterials' surface characteristics, nanoparticles can enhance their biocompatibility and functionality.191 Our expanding knowledge of the role of the immune system in wound healing has made new approaches to tailored therapy feasible. Immunotherapies and biomaterials that modify the immune response can lessen issues and encourage recovery. Personalized wound care makes use of data-driven strategies. To guide medical decisions and enhance results, forecasting analytics can be developed by integrating patient data, electronic health records, and molecular profiling.192 To create viable tissues and organs for transplantation or in vitro testing, advances in tissue engineering and organoids present new possibilities for customized wound healing. The goal of these methods is to create functional organs and tissues for in vitro testing or transplantation.193 Patients with a range of medical issues can receive effective and affordable healthcare through telemedicine. Personalized wound care can be enhanced by telemedicine and remote monitoring technology, which give medical professionals the ability to watch over, manage, and direct patients. Patients living in remote areas or dealing with persistent wounds should pay special attention to telemedicine.194 Patient education regarding wound care and self-management is crucial for personalized healing. Using wearable technology, interactive tools, and mobile applications, patients can take an active role in their recovery. Personalized wound healing and combination therapies, which rely on novel biomaterials and technologies, present a stimulating prospect to enhance health results and revolutionize the regenerative medicine domain. These developments could hasten healing, lessen complications, and enhance the standard of care for patients with various wounds.188
7. Conclusion and future research
Wound healing therapy has been revolutionized by 3D biomaterials. The intricate structure and function of their imitated natural tissues make them extremely successful in treating burns, surgical incisions, diabetic foot ulcers, and chronic wounds. 3D biomaterials provide a customized, patient-specific approach that accelerates healing, lowers complications, and improves overall care quality, as demonstrated by case studies and applications. Using these biomaterials, which may be precisely shaped to meet the needs of the wound, growth factors, antibiotics, and other therapeutic compounds can be supplied directly to the wound site for targeted treatment.
3D biomaterials create an environment that is wet and supportive of cell development and regeneration while acting as a barrier against infection. Patient care and monitoring are further enhanced by integrating technology like 3D printing, smart dressings, telemedicine, and data analytics. Numerous wounds, such as surgical wounds, burns, chronic wounds, and diabetic foot ulcers, have been treated using them. Future studies should concentrate on improving the customization of 3D biomaterials to match the distinct requirements and features of every patient, investigating the synergistic effects of combining 3D biomaterials with other therapies like immunomodulation, hyperbaric oxygen, or stem cells, and creating intelligent 3D biomaterials that can actively track the progress of wound healing and modify treatment as necessary.
To ensure the safe and effective use of 3D biomaterials in wound healing, standardized regulatory regulations must be developed. Progress in research and development opens up new opportunities for improved patient outcomes, simpler procedures, and improved quality of life for people with burns, surgical wounds, chronic wounds, and diabetic foot ulcers. These innovative biomaterials show how science and medicine can coexist, and they could completely transform the wound-dressing market.
Modern wound care employs 3D biomaterials in novel ways, such as dressings that have biological activity built in and may be used for extended periods of time, as well as the capacity to retain and release bioactive substances—like medications—in a controlled and sustained manner. Advanced wound dressings are being developed using a variety of materials, including natural inert and bioactive polymers, hydrogels, tissue-engineered skin substitutes, alginate dressings, and polymers such as cellulose, gelatin, polyurethane, polyethylene oxide, polyvinyl alcohol, hyaluronic acid, chitosan, and poly(L-lactide-co-ε-caprolactone). Moreover, 3D-printed nanoparticles are used to transport cellular components, anticoagulants, antimicrobials, and anti-inflammatory drugs.
The effectiveness of various 3D-printed nanomaterials in promoting wound healing processes has been confirmed by both in vitro and in vivo studies. Tissue regeneration, drug loading and release efficiency, moisture absorption capacity, biodegradability, resolution and viability, and synergistic anti-infective responses are only a few factors considered in these assessments. Integrating bioinformatics, computational modeling, and advanced imaging techniques could optimize bio-derived materials design for enhanced wound healing outcomes. Future 3D biomaterial success in clinical wound healing applications hinges on coordinated and multidisciplinary efforts across nanomedicine, materials science, and related fields, with scalability, consistency, traceability, and adherence to relevant regulatory standards.
Data availability
No primary research results, software or code have been included and no new data were generated or analysed as part of this review.
Author contributions
S. Singaravelu: methodology, data curation, formal analysis, investigation, writing – original draft, visualization, software. H. Abrahamse: co-supervision, writing – review & editing, funding acquisition, resources. S. S. Dhilip Kumar: conceptualization, project administration, supervision, visualization, software, writing – review & editing. All authors read and approved the final manuscript.
Conflicts of interest
There are no conflicts to declare.
Acknowledgements
This work is based on the research supported by the South African Research Chairs Initiative of the Department of Science and Technology and National Research Foundation of South Africa (Grant No. 98337), as well as grants received from the University of Johannesburg (URC), the National Research Foundation (NRF), and the CSIR (Council for Scientific and Industrial Research) – NLC (National Laser Centre) Laser Rental Pool Programme.
References
- S. A. Eming, P. Martin and M. Tomic-Canic, Sci. Transl. Med., 2014, 6, 265sr266 CrossRef.
- R. G. Wilkins and M. Unverdorben, Adv. Skin Wound Care, 2013, 26, 160–163 CrossRef.
- V. Yin, J. P. Cobb, S. C. Wightman, S. M. Atay, T. Harano and A. W. Kim, World J. Surg., 2023, 47, 2392–2400 CrossRef PubMed.
- C. Kamel, L. McGahan, M. Mierzwinski-Urban and J. Embil, Canadian Evidence and Guidelines, 2013 Search PubMed.
- J. Holl, C. Kowalewski, Z. Zimek, P. Fiedor, A. Kaminski, T. Oldak, M. Moniuszko and A. Eljaszewicz, Cells, 2021, 10, 655 CrossRef CAS.
- B. M. Kyaw, K. Jaerbrink, L. Martinengo, J. Car, K. Harding and A. Schmidtchen, Acta Derm.-Venereol., 2018, 98, 157–158 CrossRef.
- C. K. Sen, G. M. Gordillo, S. Roy, R. Kirsner, L. Lambert, T. K. Hunt, F. Gottrup, G. C. Gurtner and M. T. Longaker, Wound Repair Regener., 2009, 17, 763–771 CrossRef PubMed.
- F. Gottrup, P. Holstein, B. Jørgensen, M. Lohmann and T. Karlsmar, Arch. Surg., 2001, 136, 765–772 CrossRef CAS PubMed.
- M. B. Dreifke, A. A. Jayasuriya and A. C. Jayasuriya, Mater. Sci. Eng., C, 2015, 48, 651–662 CrossRef CAS.
- D. Wainwright, Burns, 1995, 21, 243–248 CAS.
- T. Manon-Jensen, N. Kjeld and M. Karsdal, J. Thromb. Haemostasis, 2016, 14, 438–448 CAS.
- D. A. Hickman, C. L. Pawlowski, U. D. Sekhon, J. Marks and A. S. Gupta, Adv. Mater., 2018, 30, 1700859 Search PubMed.
- A. Simpson, A. Shukla and A. C. Brown, Annu. Rev. Biomed. Eng., 2022, 24, 111–135 CAS.
- Z. Hu, D.-Y. Zhang, S.-T. Lu, P.-W. Li and S.-D. Li, Mar. Drugs, 2018, 16, 273 CrossRef PubMed.
- Q. He, K. Gong, Q. Ao, T. Ma, Y. Yan, Y. Gong and X. Zhang, J. Biomater. Appl., 2013, 27, 1032–1045 Search PubMed.
- Y. Zhong, H. Hu, N. Min, Y. Wei, X. Li and X. Li, Ann. Transl. Med., 2021, 9(7), 577 CAS.
- H. Yuan, L. Chen and F. F. Hong, ACS Appl. Mater. Interfaces, 2019, 12, 3382–3392 Search PubMed.
- S. Kattula, J. R. Byrnes and A. S. Wolberg, Arterioscler., Thromb., Vasc. Biol., 2017, 37, e13–e21 CAS.
- W. Danker III, A. DeAnglis, N. Ferko, D. Garcia and A. Hogan, Ann. Med. Surg., 2021, 61, 161–168 Search PubMed.
- Z. Li, A. Milionis, Y. Zheng, M. Yee, L. Codispoti, F. Tan, D. Poulikakos and C. H. Yap, Nat. Commun., 2019, 10, 5562 Search PubMed.
- E. Ramadan, T. Borg, G. Abdelghani and N. Saleh, Future J. Pharm. Sci., 2018, 4, 166–174 Search PubMed.
- C. Longinotti, Burns Trauma, 2014, 2, 2321–3868 Search PubMed.
- J. Sigurjonsson, D. Hedman, P. Bansch and U. Schött, Perioper. Med., 2018, 7, 1–11 CrossRef PubMed.
- C. Liu, X. Liu, C. Liu, N. Wang, H. Chen, W. Yao, G. Sun, Q. Song and W. Qiao, Biomaterials, 2019, 205, 23–37 CrossRef CAS PubMed.
- X. Qiu, J. Zhang, L. Cao, Q. Jiao, J. Zhou, L. Yang, H. Zhang and Y. Wei, ACS Appl. Mater. Interfaces, 2021, 13, 7060–7069 CrossRef CAS PubMed.
- K. Y. Lee and D. J. Mooney, Chem. Rev., 2001, 101, 1869–1880 CrossRef CAS PubMed.
- D. Li, J. Chen, X. Wang, M. Zhang, C. Li and J. Zhou, Front. Bioeng. Biotechnol., 2020, 8, 926 CrossRef PubMed.
- A. Froelich, E. Jakubowska, M. Wojtyłko, B. Jadach, M. Gackowski, P. Gadziński, O. Napierała, Y. Ravliv and T. Osmałek, Pharmaceutics, 2023, 15, 1142 CrossRef CAS PubMed.
- R. Cui, F. Chen, Y. Zhao, W. Huang and C. Liu, J. Mater. Chem. B, 2020, 8, 8282–8293 RSC.
- Y. Wang, G. Liu, L. Wu, H. Qu, D. Song, H. Huang, C. Wu and M. Xu, Int. J. Biol. Macromol., 2020, 158, 1319–1329 CrossRef CAS PubMed.
- E. N. Zare, D. Khorsandi, A. Zarepour, H. Yilmaz, T. Agarwal, S. Hooshmand, R. Mohammadinejad, F. Ozdemir, O. Sahin and S. Adiguzel, Bioact. Mater., 2024, 31, 87–118 Search PubMed.
- S. Aslani, M. Kabiri, S. HosseinZadeh, H. Hanaee-Ahvaz, E. S. Taherzadeh and M. Soleimani, Microvasc. Res., 2020, 131, 104027 CAS.
- A. Gupta and V. Kumar, Eur. Polym. J., 2007, 43, 4053–4074 CrossRef CAS.
- D. Zhao, T. Zhu, J. Li, L. Cui, Z. Zhang, X. Zhuang and J. Ding, Bioact. Mater., 2021, 6, 346–360 CAS.
- L.-T. Lim, R. Auras and M. Rubino, Prog. Polym. Sci., 2008, 33, 820–852 CrossRef CAS.
- J. R. Dorgan, H. J. Lehermeier, L. I. Palade and J. Cicero, Macromol. Symp., 2001, 175, 55–66 CrossRef CAS.
- E. Göktürk and H. Erdal, Sakarya University Journal of Science, 2017, 21, 1237–1244 Search PubMed.
- K. M. Lewis, D. Spazierer, P. Slezak, B. Baumgartner, J. Regenbogen and H. Gulle, J. Biomater. Appl., 2014, 29, 780–788 CrossRef CAS PubMed.
- K. M. Lewis, C. E. Kuntze and H. Gulle, Med. Devices: Evidence Res., 2015, 1–10 CAS.
- F. Hajiali, S. Tajbakhsh and A. Shojaei, Polym. Rev., 2018, 58, 164–207 CrossRef CAS.
- N. Raina, R. Pahwa, J. K. Khosla, P. N. Gupta and M. Gupta, Polym. Bull., 2021, 1–23 Search PubMed.
- A. Schiavi, R. Cuccaro and A. Troia, J. Mech. Behav. Biomed. Mater., 2016, 53, 119–130 CrossRef CAS PubMed.
- K. M. Zia, S. Tabasum, M. F. Khan, N. Akram, N. Akhter, A. Noreen and M. Zuber, Int. J. Biol. Macromol., 2018, 109, 1068–1087 CrossRef CAS.
- D. N. Rockwood, R. C. Preda, T. Yücel, X. Wang, M. L. Lovett and D. L. Kaplan, Nat. Protoc., 2011, 6, 1612–1631 CAS.
- S. Türkkan, D. Atila, A. Akdağ and A. Tezcaner, J. Biomed. Mater. Res., Part B, 2018, 106, 2625–2635 CrossRef PubMed.
- M. Rajabi, A. Ali, M. McConnell and J. Cabral, Mater. Sci. Eng., C, 2020, 110, 110612 CrossRef CAS PubMed.
- S. Singaravelu, G. Ramanathan and U. T. Sivagnanam, Mater. Sci. Eng., C, 2017, 76, 37–49 CrossRef CAS PubMed.
- H. R. Bakhsheshi-Rad, A. F. Ismail, M. Aziz, M. Akbari, Z. Hadisi, M. Omidi and X. Chen, Int. J. Biol. Macromol., 2020, 149, 513–521 CAS.
- R. De Silva, M. Mantilaka, K. Goh, S. Ratnayake, G. Amaratunga and K. N. de Silva, Int. J. Biomater., 2017, 2017, 1391298 CAS.
- S. Biranje, P. Madiwale and R. Adivarekar, J. Porous Mater., 2019, 26, 29–40 Search PubMed.
- D. Akbik, M. Ghadiri, W. Chrzanowski and R. Rohanizadeh, Life Sci., 2014, 116, 1–7 CrossRef CAS.
- C. Mohanty and S. K. Sahoo, Drug Discovery Today, 2017, 22, 1582–1592 CrossRef CAS PubMed.
- R. Yaghoobi and A. Kazerouni, Jundishapur J. Nat. Pharm. Prod., 2013, 8, 100 CrossRef.
- F. Khan, Z. U. Abadin and N. Rauf, Int. J. Clin. Pract., 2007, 61, 1705–1707 CrossRef CAS PubMed.
- V. P. Shenoy, M. Ballal, P. Shivananda and I. Bairy, J. Global Infect. Dis., 2012, 4, 102–105 CrossRef.
- P. G. Shakespeare, Clin. Dermatol., 2005, 23, 413–418 CrossRef PubMed.
- L. Chen, H. Deng, H. Cui, J. Fang, Z. Zuo, J. Deng, Y. Li, X. Wang and L. Zhao, Oncotarget, 2018, 9, 7204 CrossRef.
- S. Chhabra, N. Chhabra, A. Kaur and N. Gupta, J. Maxillofac. Oral Surg., 2017, 16, 403–423 CrossRef PubMed.
- S. S. Mathew-Steiner, S. Roy and C. K. Sen, Bioengineering, 2021, 8, 63 CrossRef CAS PubMed.
- S. Enoch and P. Price, World Wide Wounds, 2004, 1–12 Search PubMed.
- I. A. Darby, B. Laverdet, F. Bonté and A. Desmoulière, Clin., Cosmet. Invest. Dermatol., 2014, 301–311 Search PubMed.
- X.-T. Trinh, N.-V. Long, L. T. Van Anh, P. T. Nga, N. N. Giang, P. N. Chien, S.-Y. Nam and C.-Y. Heo, Int. J. Mol. Sci., 2022, 23, 9573 CrossRef CAS PubMed.
- I. Pastar, O. Stojadinovic, N. C. Yin, H. Ramirez, A. G. Nusbaum, A. Sawaya, S. B. Patel, L. Khalid, R. R. Isseroff and M. Tomic-Canic, Adv. Wound Care, 2014, 3, 445–464 CrossRef PubMed.
- A. Sood, M. S. Granick and N. L. Tomaselli, Adv. Wound Care, 2014, 3, 511–529 CrossRef.
- K. P. Wilhelm, D. Wilhelm and S. Bielfeldt, Sking Res. Technol., 2017, 23, 3–12 CrossRef.
- S. Riwaldt, T. J. Corydon, D. Pantalone, J. Sahana, P. Wise, M. Wehland, M. Krüger, D. Melnik, S. Kopp and M. Infanger, Front. Bioeng. Biotechnol., 2021, 9, 679650 CrossRef.
- H. N. Wilkinson and M. J. Hardman, Open Biol., 2020, 10, 200223 CrossRef CAS PubMed.
- H. P. Ehrlich and T. K. Hunt, Adv. Wound Care, 2012, 1, 3–9 CrossRef.
- A.-G. Niculescu and A. M. Grumezescu, Polymers, 2022, 14, 421 CrossRef CAS PubMed.
- A. Nourian Dehkordi, F. Mirahmadi Babaheydari, M. Chehelgerdi and S. Raeisi Dehkordi, Stem Cell Res. Ther., 2019, 10, 1–20 CrossRef PubMed.
- A. A. Chaudhari, K. Vig, D. R. Baganizi, R. Sahu, S. Dixit, V. Dennis, S. R. Singh and S. R. Pillai, Int. J. Mol. Sci., 2016, 17, 1974 CrossRef PubMed.
- R. Yu, H. Zhang and B. Guo, Nano-Micro Lett., 2022, 14, 1–46 CrossRef CAS PubMed.
- M. Norouzi, S. M. Boroujeni, N. Omidvarkordshouli and M. Soleimani, Adv. Healthcare Mater., 2015, 4, 1114–1133 CrossRef CAS PubMed.
- R. Z. Murray, Z. E. West, A. J. Cowin and B. L. Farrugia, Burns Trauma, 2019, 7 DOI:10.1186/s41038-018-0139-7.
- S. Vach Agocsova, M. Culenova, I. Birova, L. Omanikova, B. Moncmanova, L. Danisovic, S. Ziaran, D. Bakos and P. Alexy, Materials, 2023, 16, 4267 CrossRef CAS PubMed.
- E. Saiz, E. A. Zimmermann, J. S. Lee, U. G. Wegst and A. P. Tomsia, Dent. Mater., 2013, 29, 103–115 CrossRef CAS PubMed.
- M. P. Nikolova and M. S. Chavali, Bioact. Mater., 2019, 4, 271–292 Search PubMed.
- S. P. Ndlovu, K. Ngece, S. Alven and B. A. Aderibigbe, Polymers, 2021, 13, 2959 CrossRef CAS PubMed.
- R. Xie, V. Pal, Y. Yu, X. Lu, M. Gao, S. Liang, M. Huang, W. Peng and I. T. Ozbolat, Biomaterials, 2023, 122408 Search PubMed.
- J. S. Boateng, K. H. Matthews, H. N. Stevens and G. M. Eccleston, J. Pharm. Sci., 2008, 97, 2892–2923 CrossRef CAS.
- C. Zhao, J. Yang, W. Chen, C. Lu, Z. Zeng, T. Jiang and W. Liu, Int. J. Pharm., 2024, 663, 124575 CrossRef CAS.
- B. Wang, X. Lv, S. Chen, Z. Li, J. Yao, X. Peng, C. Feng, Y. Xu and H. Wang, Carbohydr. Polym., 2018, 181, 948–956 CrossRef CAS PubMed.
- J. M. Pachence, J. Biomed. Mater. Res., 1996, 33, 35–40 CrossRef CAS PubMed.
- N. F. Huang, T. S. Zaitseva and M. V. Paukshto, Bioengineering, 2023, 10, 90 CrossRef PubMed.
- K. P. Robb, A. Shridhar and L. E. Flynn, ACS Biomater. Sci. Eng., 2017, 4, 3627–3643 CrossRef PubMed.
- Z. Heydari, M. Najimi, H. Mirzaei, A. Shpichka, M. Ruoss, Z. Farzaneh, L. Montazeri, A. Piryaei, P. Timashev and R. Gramignoli, Cells, 2020, 9, 304 CrossRef CAS PubMed.
- Y. Wang, D. B. Gunasekara, M. I. Reed, M. DiSalvo, S. J. Bultman, C. E. Sims, S. T. Magness and N. L. Allbritton, Biomaterials, 2017, 128, 44–55 CrossRef CAS PubMed.
- M. C Echave, L. S Burgo, J. L Pedraz and G. Orive, Curr. Pharm. Des., 2017, 23, 3567–3584 CrossRef PubMed.
- M. H. Amer, F. R. Rose, K. M. Shakesheff and L. J. White, Stem Cell Res. Ther., 2018, 9, 1–15 CrossRef PubMed.
- C. Vepari and D. L. Kaplan, Prog. Polym. Sci., 2007, 32, 991–1007 CrossRef CAS PubMed.
- M. Brovold, J. I. Almeida, I. Pla-Palacín, P. Sainz-Arnal, N. Sánchez-Romero, J. J. Rivas, H. Almeida, P. R. Dachary, T. Serrano-Aulló and S. Soker, Novel Biomaterials for Regenerative Medicine, 2018, pp. 421–449 Search PubMed.
- S. Takeda, in Encyclopedia of Insects, Elsevier, 2009, pp. 117–119 Search PubMed.
- Y. Li, Y. Wei, G. Zhang and Y. Zhang, Polymers, 2023, 15, 2941 CrossRef CAS PubMed.
- Z. H. Mok, Pharm. Sci. Adv., 2024, 2, 100031 CrossRef.
- Q. Ye, G. Zünd, P. Benedikt, S. Jockenhoevel, S. P. Hoerstrup, S. Sakyama, J. A. Hubbell and M. Turina, Eur. J. Cardio. Thorac. Surg., 2000, 17, 587–591 CrossRef CAS PubMed.
- A. Khodakaram-Tafti, D. Mehrabani and H. Shaterzadeh-Yazdi, Dent. Res. J., 2017, 14, 79 CrossRef.
- M. Y. Eo, H. Fan, Y. J. Cho, S. M. Kim and S. K. Lee, Biomater. Res., 2016, 20, 16 CrossRef PubMed.
- E. Entcheva, H. Bien, L. Yin, C.-Y. Chung, M. Farrell and Y. Kostov, Biomaterials, 2004, 25, 5753–5762 CrossRef CAS PubMed.
- A. Svensson, E. Nicklasson, T. Harrah, B. Panilaitis, D. L. Kaplan, M. Brittberg and P. Gatenholm, Biomaterials, 2005, 26, 419–431 CrossRef CAS PubMed.
- B. K. Park and M.-M. Kim, Int. J. Mol. Sci., 2010, 11, 5152–5164 CrossRef CAS PubMed.
- J. Venkatesan and S.-K. Kim, Mar. Drugs, 2010, 8, 2252–2266 CrossRef CAS PubMed.
- D.-K. Kweon, S.-B. Song and Y.-Y. Park, Biomaterials, 2003, 24, 1595–1601 CrossRef CAS PubMed.
- H. Ueno, H. Yamada, I. Tanaka, N. Kaba, M. Matsuura, M. Okumura, T. Kadosawa and T. Fujinaga, Biomaterials, 1999, 20, 1407–1414 CrossRef CAS PubMed.
- Y. Yu, R. Chen, Y. Sun, Y. Pan, W. Tang, S. Zhang, L. Cao, Y. Yuan, J. Wang and C. Liu, Acta Biomater., 2018, 71, 510–521 CAS.
- S. Thomas, J. Wound Care, 2000, 9, 56–60 CAS.
- T. Kumar Giri, D. Thakur, A. Alexander, H. Badwaik and D. Krishna Tripathi, Curr. Drug Delivery, 2012, 9, 539–555 CrossRef PubMed.
- A. Petrosyan, F. Montali, A. Peloso, A. Citro, L. N. Byers, C. La Pointe, M. Suleiman, A. Marchetti, E. P. Mcneill and A. L. Speer, Front. Bioeng. Biotechnol., 2022, 10, 1015628 CrossRef PubMed.
- H. Bendz and W. Friess, Eur. J. Pharm. Biopharm., 2003, 1, 147–148 CrossRef.
- M. A. Salati, J. Khazai, A. M. Tahmuri, A. Samadi, A. Taghizadeh, M. Taghizadeh, P. Zarrintaj, J. D. Ramsey, S. Habibzadeh and F. Seidi, Polymers, 2020, 12, 1150 CrossRef CAS PubMed.
- R. L. Mauck, M. A. Soltz, C. C. Wang, D. D. Wong, P.-H. G. Chao, W. B. Valhmu, C. T. Hung and G. A. Ateshian, J. Biomech. Eng., 2000, 122, 252–260 CrossRef CAS PubMed.
- G. R. Erickson, J. M. Gimble, D. M. Franklin, H. E. Rice, H. Awad and F. Guilak, Biochem. Biophys. Res. Commun., 2002, 290, 763–769 CrossRef CAS PubMed.
- D. R. Diduch, L. C. Jordan, C. M. Mierisch and G. Balian, Arthroscopy, 2000, 16, 571–577 CrossRef CAS PubMed.
- M. Chelu and A. M. Musuc, Processes, 2023, 11, 2696 CrossRef CAS.
- H. S. Yoo, E. A. Lee, J. J. Yoon and T. G. Park, Biomaterials, 2005, 26, 1925–1933 CrossRef CAS PubMed.
- N. Davidenko, J. Campbell, E. Thian, C. Watson and R. Cameron, Acta Biomater., 2010, 6, 3957–3968 CAS.
- S. V. Kushchayev, M. B. Giers, D. H. Eng, N. L. Martirosyan, J. M. Eschbacher, M. M. Mortazavi, N. Theodore, A. Panitch and M. C. Preul, J. Neurosurg., 2016, 25, 114–124 Search PubMed.
- C.-T. Lee, P.-H. Kung and Y.-D. Lee, Carbohydr. Polym., 2005, 61, 348–354 CrossRef CAS.
- J.-P. Bali, H. Cousse and E. Neuzil, Semin. Arthritis Rheum., 2001, 31, 58–68 CrossRef CAS PubMed.
- A. Södergård and M. Stolt, Prog. Polym. Sci., 2002, 27, 1123–1163 CrossRef.
- P. K. Samantaray, A. Little, D. M. Haddleton, T. McNally, B. Tan, Z. Sun, W. Huang, Y. Ji and C. Wan, Green Chem., 2020, 22, 4055–4081 RSC.
- D. H. Rehkopf, F. F. Furstenberg, H. Elser, C. Jackson, N. Levy, J. W. Rowe and A. S. R. Network, Milbank Q., 2022, 100, 102–133 CrossRef PubMed.
- R. J. Zdrahala and I. J. Zdrahala, J. Biomater. Appl., 1999, 14, 67–90 CrossRef CAS PubMed.
- Q. Huang, T. Wu, L. Wang, J. Zhu, Y. Guo, X. Yu, L. Fan, J. H. Xin and H. Yu, Biomater. Sci., 2022, 10, 2568–2576 RSC.
- L. Xu, Y. Chen, P. Zhang, J. Tang, Y. Xue, H. Luo, R. Dai, J. Jin and J. Liu, Biomater. Sci., 2022, 10, 5648–5661 RSC.
- E. Salernitano and C. Migliaresi, J. Appl. Biomater. Biomech., 2003, 1, 3–18 CAS.
- S. S. Silva, N. M. Oliveira, M. B. Oliveira, D. P. S. da Costa, D. Naskar, J. F. Mano, S. C. Kundu and R. L. Reis, Acta Biomater., 2016, 32, 178–189 CrossRef CAS PubMed.
- C. D. Weller, V. Team and G. Sussman, Front. Pharmacol., 2020, 11, 155 CrossRef CAS PubMed.
- B. A. Aderibigbe and B. Buyana, Pharmaceutics, 2018, 10, 42 CrossRef PubMed.
- N. Namviriyachote, P. Muangman, K. Chinaroonchai, C. Chuntrasakul and G. C. Ritthidej, Int. J. Biol. Macromol., 2020, 143, 510–520 CrossRef CAS PubMed.
- Y. Guan, F. Cheng and Z. Pan, Polymers, 2019, 11, 806 Search PubMed.
- Y. Guo, R. Ma, M. Zhang, Y. Cao, Z. Zhang and W. Yang, Vaccines, 2023, 11, 1440 Search PubMed.
- A. Vedadghavami, F. Minooei, M. H. Mohammadi, S. Khetani, A. R. Kolahchi, S. Mashayekhan and A. Sanati-Nezhad, Acta Biomater., 2017, 62, 42–63 CrossRef CAS PubMed.
- Y. Wu, Y. Wang, L. Long, C. Hu, Q. Kong and Y. Wang, J. Controlled Release, 2022, 341, 147–165 CrossRef CAS PubMed.
- Z. Arabpour, F. Abedi, M. Salehi, S. M. Baharnoori, M. Soleimani and A. R. Djalilian, Int. J. Mol. Sci., 2024, 25, 1982 CrossRef CAS PubMed.
- W. Zhong, in Advances in Smart Medical Textiles, Elsevier, 2016, pp. 57–70 Search PubMed.
- H. R. Fernandes, A. Gaddam, A. Rebelo, D. Brazete, G. E. Stan and J. M. Ferreira, Materials, 2018, 11, 2530 CrossRef CAS PubMed.
- L. L. Hench, R. J. Splinter, W. Allen and T. Greenlee, J. Biomed. Mater. Res., 1971, 5, 117–141 CrossRef.
- D. Wheeler, K. Stokes, R. Hoellrich, D. Chamberland and S. McLoughlin, J. Biomed. Mater. Res., 1998, 41, 527–533 CrossRef CAS PubMed.
- E. Jablonská, D. Horkavcová, D. Rohanová and D. S. Brauer, J. Mater. Chem. B, 2020, 8, 10941–10953 RSC.
- S. M. Peltola, F. P. Melchels, D. W. Grijpma and M. Kellomäki, Ann. Med., 2008, 40, 268–280 CrossRef CAS PubMed.
- K. C. Hribar, P. Soman, J. Warner, P. Chung and S. Chen, Lab Chip, 2014, 14, 268–275 RSC.
- K. Leong, C. Cheah and C. Chua, Biomaterials, 2003, 24, 2363–2378 CrossRef CAS PubMed.
- F. S. A. Khan, N. Mubarak, M. Khalid, R. Walvekar, E. Abdullah, A. Ahmad, R. R. Karri and H. Pakalapati, Sci. Rep., 2021, 11, 843 CrossRef CAS PubMed.
- S. Bao and S. C. Tjong, Mater. Sci. Eng., A, 2008, 485, 508–516 CrossRef.
- S. K. Misra, T. I. Ansari, S. P. Valappil, D. Mohn, S. E. Philip, W. J. Stark, I. Roy, J. C. Knowles, V. Salih and A. R. Boccaccini, Biomaterials, 2010, 31, 2806–2815 CrossRef CAS PubMed.
- X. Huang, I. H. El-Sayed, W. Qian and M. A. El-Sayed, J. Am. Chem. Soc., 2006, 128, 2115–2120 CrossRef CAS PubMed.
- Y. Xiao, H. Hong, V. Z. Matson, A. Javadi, W. Xu, Y. Yang, Y. Zhang, J. W. Engle, R. J. Nickles and W. Cai, Theranostics, 2012, 2, 757 CrossRef CAS PubMed.
- C. Wang, Z. Ma, T. Wang and Z. Su, Adv. Funct. Mater., 2006, 16, 1673–1678 CrossRef CAS.
- Arnida, A. Malugin and H. Ghandehari, J. Appl. Toxicol., 2010, 30, 212–217 CrossRef CAS PubMed.
- F. Chen, G. Wang, J. I. Griffin, B. Brenneman, N. K. Banda, V. M. Holers, D. S. Backos, L. Wu, S. M. Moghimi and D. Simberg, Nat. Nanotechnol., 2017, 12, 387–393 CrossRef CAS PubMed.
- M. Vedhanayagam, R. Mohan, B. U. Nair and K. J. Sreeram, Biomed. Mater., 2015, 10, 065010 CrossRef PubMed.
- X. Liu, M. N. George, S. Park, A. L. Miller II, B. Gaihre, L. Li, B. E. Waletzki, A. Terzic, M. J. Yaszemski and L. Lu, Acta Biomater., 2020, 111, 129–140 CrossRef CAS PubMed.
- K. K. Rao, T. S. Vani, B. A. Lakshmi, D. Hemalatha and K. M. Rao, Surface Modified Carbon Nanotubes Volume 2: Industrial Applications, 2022, pp. 1–17 Search PubMed.
- A. Gholami, S. A. Hashemi, K. Yousefi, S. M. Mousavi, W.-H. Chiang, S. Ramakrishna, S. Mazraedoost, A. Alizadeh, N. Omidifar and G. Behbudi, J. Nanomater., 2020, 2020, 1852946 Search PubMed.
- P. Monika, M. N. Chandraprabha, A. Rangarajan, P. V. Waiker and K. N. Chidambara Murthy, Front. Nutr., 2022, 8, 791899 CrossRef PubMed.
- E. Eriksson, P. Y. Liu, G. S. Schultz, M. M. Martins-Green, R. Tanaka, D. Weir, L. J. Gould, D. G. Armstrong, G. W. Gibbons and R. Wolcott, Wound Repair Regener., 2022, 30, 156–171 CrossRef PubMed.
- M. Rodrigues, N. Kosaric, C. A. Bonham and G. C. Gurtner, Physiol. Rev., 2019, 99, 665–706 CrossRef CAS PubMed.
- C. M. Sena, C. F. Bento, P. Pereira, F. Marques and R. Seiça, New Strategies to Advance Pre/Diabetes Care: Integrative Approach by PPPM, 2013, pp. 29–87 Search PubMed.
- G. Han and R. Ceilley, Adv. Ther., 2017, 34, 599–610 CrossRef PubMed.
- B. Sheokand, M. Vats, A. Kumar, C. M. Srivastava, I. Bahadur and S. R. Pathak, J. Polym. Sci., 2023, 61, 1389–1414 CAS.
- R. G. Frykberg and J. Banks, Adv. Wound Care, 2015, 4, 560–582 Search PubMed.
- M. Flanagan, Wounds, 2005, 1, 74 Search PubMed.
- D. T. Ubbink, F. E. Brölmann, P. M. Go and H. Vermeulen, Adv. Wound Care, 2015, 4, 286–294 CrossRef PubMed.
- R. S. Hotchkiss, L. L. Moldawer, S. M. Opal, K. Reinhart, I. R. Turnbull and J.-L. Vincent, Nat. Rev. Dis. Primers, 2016, 2, 1–21 Search PubMed.
- Z. Li, H. Wang, B. Yang, Y. Sun and R. Huo, Mater. Sci. Eng., C, 2015, 57, 181–188 CAS.
- R. O. Hynes and A. Naba, Cold Spring Harbor Perspect. Biol., 2012, 4, a004903 Search PubMed.
- N. J. Castro, C. Meinert, P. Levett and D. W. Hutmacher, Curr. Opin. Biomed. Eng., 2017, 2, 67–75 CrossRef.
- S. Kanwar and S. Vijayavenkataraman, Bioprinting, 2021, 24, e00167 CrossRef.
- T. Li, J. Chang, Y. Zhu and C. Wu, Adv. Healthcare Mater., 2020, 9, 2000208 CrossRef CAS PubMed.
- H. Chopra, S. Kumar, S. Z. Safi, I. Singh and T. B. Emran, Int. J. Surg., 2022, 104, 106793 CrossRef PubMed.
- G. Beltrami, G. Ristori, A. M. Nucci, A. Galeotti, A. Tamburini, G. Scoccianti, D. Campanacci, M. Innocenti and R. Capanna, J. Clin. Med., 2021, 10, 1056 CrossRef PubMed.
- S. Khunmanee, Y. Jeong and H. Park, J. Tissue Eng., 2017, 8, 2041731417726464 CrossRef PubMed.
- B. C. Pereira, A. Isreb, R. T. Forbes, F. Dores, R. Habashy, J.-B. Petit, M. A. Alhnan and E. F. Oga, Eur. J. Pharm. Biopharm., 2019, 135, 94–103 CrossRef CAS PubMed.
- J. Carlsson, C.-G. Stålnacke, H. Acker, M. Haji-Karim, S. Nilsson and B. Larsson, Int. J. Radiat. Oncol., Biol., Phys., 1979, 5, 2011–2020 CrossRef CAS PubMed.
- J. Choi, E. J. Lee, W. B. Jang and S.-M. Kwon, J. Funct. Biomater., 2023, 14, 497 CrossRef CAS PubMed.
- N. Celikkin, D. Presutti, F. Maiullari, E. Fornetti, T. Agarwal, A. Paradiso, M. Volpi, W. Święszkowski, C. Bearzi and A. Barbetta, Front. Bioeng. Biotechnol., 2021, 9, 732130 CrossRef PubMed.
- P. Wiśniewska, M. R. Saeb and S. A. Bencherif, Front. Biomater. Sci., 2023, 2, 1260402 CrossRef PubMed.
- J. S. Miller, PLoS Biol., 2014, 12, e1001882 Search PubMed.
- H. Kanďárová and P. Pôbiš, Front. Toxicol., 2024, 5, 1337468 Search PubMed.
- S. Bhatti and J. Singh, Int. J. Interact. Des. Manuf., 2023, 1–22 Search PubMed.
- S. F. Badylak, D. O. Freytes and T. W. Gilbert, Acta Biomater., 2009, 5, 1–13 CAS.
- W. L. Ng, J. M. Lee, M. Zhou, Y.-W. Chen, K.-X. A. Lee, W. Y. Yeong and Y.-F. Shen, Biofabrication, 2020, 12, 022001 CAS.
- A. C. Sommer and E. Z. Blumenthal, Graefe's Arch. Clin. Exp. Ophthalmol., 2019, 257, 1815–1822 Search PubMed.
- S. V. Murphy and A. Atala, Nat. Biotechnol., 2014, 32, 773–785 CAS.
- J. Y. Wong, Aerosp. Med. Hum. Perform., 2015, 86, 830–834 Search PubMed.
- A. Pietrabissa, S. Marconi, A. Peri, L. Pugliese, E. Cavazzi, A. Vinci, M. Botti and F. Auricchio, Surg. Endosc., 2016, 30, 366–371 Search PubMed.
- A. V. Do, B. Khorsand, S. M. Geary and A. K. Salem, Adv. Healthcare Mater., 2015, 4, 1742–1762 CAS.
- J. Sobek, K. Bartscherer, A. Jacob, J. D. Hoheisel and P. Angenendt, Comb. Chem. High Throughput Screening, 2006, 9, 365–380 CAS.
- A. Pusta, M. Tertiş, C. Cristea and S. Mirel, Biosensors, 2021, 12, 1 Search PubMed.
- P. R. Baraniak and T. C. McDevitt, Regener. Med., 2010, 5, 121–143 CrossRef PubMed.
- M. Kitamura, K. Nakashima, Y. Kowashi, T. Fujii, H. Shimauchi, T. Sasano, T. Furuuchi, M. Fukuda, T. Noguchi and T. Shibutani, PLoS One, 2008, 3, e2611 CrossRef PubMed.
- T. J. Keane, I. T. Swinehart and S. F. Badylak, Methods, 2015, 84, 25–34 CrossRef CAS PubMed.
- W. Sun, B. Starly, A. C. Daly, J. A. Burdick, J. Groll, G. Skeldon, W. Shu, Y. Sakai, M. Shinohara and M. Nishikawa, Biofabrication, 2020, 12, 022002 CrossRef CAS PubMed.
- C. D. Q. Flumignan, A. P. d. Rocha, A. C. P. N. Pinto, K. M. M. Milby, M. R. Batista, Á. N. Atallah and H. Saconato, Sao Paulo Med. J., 2019, 137, 184–192 Search PubMed.
|
This journal is © The Royal Society of Chemistry 2025 |
Click here to see how this site uses Cookies. View our privacy policy here.