DOI:
10.1039/D4RA05721J
(Paper)
RSC Adv., 2025,
15, 8541-8552
Construction of CeCux-BTC/CN S-type heterojunctions and photocatalytic CO2 reduction to CO and CH4
Received
7th August 2024
, Accepted 25th September 2024
First published on 19th March 2025
Abstract
S-type heterojunction photocatalysts (CeCux-BTC/CN) of cerium-copper bimetallic organic framework (CeCux-BTC) and graphitic carbon nitride (g-C3N4) were constructed by a simple solvothermal method using cerium nitrate, copper nitrate, and urea as the raw materials, and 1,3,5-benzene-tricarboxylic acid as the ligand for the photocatalytic CO2 reduction to CO and CH4. The results show that the built-in electric field constructed by Fermi energy level flattening transfers the electrons in an S-type manner, which not only preserves the strong reducing properties of the electrons in the material but also provides the maximum redox capacity and enables the composite samples to obtain higher visible-light trapping capacity and improve the separation efficiency of the carriers while refining the crystal particles. With the addition of only 1 mL of H2O as the proton supply source, CeCu0.05-BTC/CN exhibits the optimal photocatalytic performance. The CO and CH4 yields were 64.44 and 0.5575 μmol g−1, which were 7.56 and 2.42 times higher than those of g-C3N4, respectively, and the catalytic performances were basically stable after cycling tests.
1 Introduction
Since the industrial revolution, the greenhouse effect has become more pronounced as carbon dioxide emissions have continued to increase, and the frequency of extreme weather events has led to increasingly severe global environmental problems.1,2 The emission of fossil fuels (coal, oil, natural gas, etc.) is the main source of the increase of carbon dioxide in the atmosphere.3 Therefore, it is imperative to reduce the consumption of fossil fuels and the content of carbon dioxide in the atmosphere. Carbon dioxide reduction using solar-driven semiconductor photocatalysts is an efficient and promising technology for achieving long-term solar-fuel conversion.4,5 Graphite carbon nitride (g-C3N4) is a 2D layered semiconductor,6 which is often regarded as a very promising semiconductor material due to its suitable band gap energy, good chemical-thermal stability, and low cost.7–9 However, the small specific surface area, the presence of rapid recombination of photogenerated electrons and holes, and the limited absorption and photoresponsivity to visible light limit the practical use of this material for photocatalytic carbon dioxide reduction applications. At present, several aspects of catalyst modification are common in the market, such as chemical doping,10 the construction of heterojunction,11 noble metal loading,12 and the regulation of its morphology.13 These include metal oxides, sulfides, carbon-nitrides, metal–organic skeletons (MOFs), etc.14,15
During research in the semiconductor field, MOFs have attracted the attention of a wide range of researchers due to their diverse and controllable crystal structure, ultra-high specific surface area,16,17 and tunable pores.18 Cerium (Ce) is an excellent catalytic additive as a rare earth element with unfilled electron 4f orbitals, capable of reversible changes between Ce4+ and Ce3+, and surface oxygen vacancy defects that can be spontaneously generated and eliminated. Ye et al.19 obtained oxygen vacancy-rich and N-doped MOF-derived electrocatalysts by pyrolysis of melamine-containing CuCe-BTC with high selectivity and good electrocatalytic activity compared to a reversible hydrogen electrode with a NH3 yield of 44.5 μg h−1 mgcat.−1 at 0.5 V. Zhang et al.20 prepared CuO/CeO2 catalysts synthesized from MOFs using in situ synthesis, mechanical mixing, and impregnation methods and found that the CuCeO–ETH catalysts had higher than 98% selectivity for CO oxidation and a wide temperature activity window. Zhang et al.21 achieved efficient CO2–CH4 electroconversion with a methane local current density of 138.6 mA cm−2 by pyrolysis of a metal–organic framework (MOF) precursor for the preparation of cerium carbide-copper oxide complexes (Cu/CeO2@C). Therefore, the heterostructured photocatalytic system constructed from the two may better improve the photocatalyst activity and CO2 reduction efficiency.
Currently, although some Cu-BTC, Ce-BTC and related materials of CeCu-BTC from different preparation methods have been synthesized, little research has been reported on bimetallic organic frameworks (CeCu-BTC) and graphite carbon nitride (g-C3N4) composite heterostructures for photocatalytic carbon dioxide reduction. In the present study, composite heterostructures of bimetallic organic frameworks (CeCux-BTC) and graphite carbon nitride (g-C3N4) were synthesized by a simple solvothermal method using cerium nitrate and urea as raw materials and 1,3,5-benzotricarboxylic acid as a ligand. The results show that Ce-BTC microrods surrounded by Cu-doped microspheres can form beneficial MOFs materials to synergistically improve the photocatalytic CO2 reduction activity, and this finding also paves the way for further improvement of the photocatalytic CO2 reduction performance and a small amount of low-cost composite modification of g-C3N4.
2 Experimental section
2.1 Materials
Cerium nitrate hexahydrate (Ce(NO3)3·6H2O), copper nitrate trihydrate (Cu(NO3)2·3H2O), N,N-dimethylformamide (C3H7NO), 1,3,5-benzene-tricarboxylic acid (C9H6O6), all analytically pure AR, Aladdin Reagents Ltd urea (CH4N2O), anhydrous ethanol (C2H6O), both analytically pure AR, Sinopharm Group Chemical Reagent Co. Ltd acetone (C3H6O), analytically pure AR, Tianjin Jindong Tianzheng Fine Chemical Reagent Factory.
2.2 Preparation of g-C3N4, Ce-BTC and Cu-BTC
g-C3N4 and Ce-BTC were prepared by simple thermal polymerization and solvothermal methods, respectively. The temperature was raised to 550 °C at a rate of 5 °C min−1 and held for 4 hours. After grinding, a light yellow g-C3N4 powder was obtained.
3 mmol (0.63 g) of 1,3,5-benzene tricarboxylic acid (H3BTC) and 10 mmol (2.17 g) of Ce(NO3)3 6H2O were dissolved in 50 mL (1
:
1) of a mixture of dimethylformamide (DMF) and ethanol, and the mixture was ultrasonicated for 30 min and then sealed in an autoclave at 100 mL, held at 130 °C for 24 h, naturally cooled to room temperature, washed sufficiently, and then dried under vacuum at 80 °C for 12 h. The Ce-BTC powder material was ground. Cu-BTC was prepared by replacing Ce(NO3)3 6H2O with 0.13 g of Cu(NO3)2 3H2O as described above.
2.3 Preparation of CeCux-BTC/CN composite photocatalysts
CeCux-BTC/CN composite photocatalysts were prepared by a simple solvent-thermal method. The CeCux-BTC/CN composite photocatalysts were prepared similarly to Ce-BTC, except that Cu(NO3)2 3H2O and g-C3N4 were added to the reaction solution. As an example, CeCu0.05-BTC/CN was weighed, and 0.63 g of 1,3,5-benzotricarboxylic acid (H3BTC), 2.17 g of Ce(NO3)3 6H2O, and 0.13 g of Cu(NO3)2 3H2O were dissolved in a 50 mL (1
:
1) mixture of dimethylformamide (DMF) and ethanol. And 500 mg of g-C3N4 was taken in a certain amount of anhydrous ethanol. After ultrasonic dispersion for 30 min, the two were mixed and strongly stirred and sealed in a 100 mL polytetrafluoroethylene stainless steel autoclave at 130 °C for 24 h, naturally cooled to room temperature and centrifuged, washed several times with anhydrous ethanol and acetone, and then vacuum-dried for 12 h at 80 °C and milled to obtain the CeCu0.05-BTC/CN powder material. Wherein, the obtained CeCux-BTC/g-C3N4 catalysts were named as CeCu0.05-BTC/CN, CeCu0.1-BTC/CN, CeCu0.15-BTC/CN, CeCu0.2-BTC/CN, respectively. CeCu-BTC was prepared with x = 0.05 as an example, and in all the synthesised CeCux-BTC/CN, x denotes a Cu content of 5%.
2.4 Characterization
The crystal structure was analyzed using an X-ray diffraction analyzer with a scanning range of 5 to 70°, Bruker diffractometer type D8-ADVANCE, Germany. GAIA3(XMU/XMH) Ga-ion double beam scanning electron microscope (FIB-SEM) and high-resolution field emission scanning electron microscope Hitachi SU8010 to observe the surface micro-morphology of Czechoslovakia and Japan. Transmission electron microscopy (TEM) images were taken with a FEI-Talos F200S instrument with an accelerating voltage of 200 kV, energy spectrum: X-Twin, origin Netherlands. FT-IR spectrometer to analyze the molecular structure and chemical components of the samples, NICOLET iS50FT-IR type. X-ray electron spectroscopy to analyze the elemental composition and chemical state of the samples, ESCALAB 205Xi type, all of the above are from Thermo Fisher Scientific, USA. UV-Vis Diffuse Reflectance Spectroscopy, test wavelength range 200–800 nm, Model U-3900, Hitachi High-Technologies Corporation, Japan. PL Fluorescence Spectroscopy, testing wavelength of 285 nm, Model F-4600, Hitachi High-Technologies, Japan. Beijing Perfectlight High Performance Xenon Light Source simulating daylight (PLS-SXE300E/UV), power 300 W, Beijing Perfectlight Technology Co. Fully automated system gas chromatography for photocatalytic carbon dioxide reduction testing, Model GC79720, Beijing CEC Jinyuan Technology Co. Electrochemical workstation for electrochemical performance testing of samples, Model 1260A+1287A, Sully Strong, UK. EPR electron paramagnetic resonance spectrometer using the Bruker model E500, Germany.
2.5 Photocatalytic CO2 reduction
The photocatalytic CO2 reduction experiments were carried out in a sealed 250 mL quartz glass reactor with a 28# standard ball mill and flange O-ring seal, which was connected to an automatic on-line photocatalytic analysis system using a PLS-SXE300 xenon lamp with a wavelength of 320–780 nm as the light source. Specifically, 50 mg of photocatalyst was put into the reaction bottle to build a platform, and 1 mL of deionized water was used as the reaction solution. Before illumination, vacuum the reaction system 2–3 times until the pressure gauge shows −91.7 kPa, and then charge pure CO2 gas with high-purity argon as the carrier gas. Use the circulating water chiller to maintain the temperature in the bottle and carry out the photocatalytic reaction under stable temperature conditions. The trace gas in the reaction bottle was extracted by automatic sampling every 60 min, and the yield of CO and CH4 was obtained by a GC7920 gas chromatograph (equipped with FID, TCD, ECD, and FPD detectors).
2.6 Electrochemical testing
The electrochemical tests were carried out using a three-electrode system. Glymercury electrode was used as a reference electrode, a platinum electrode as a counter electrode, a catalyst membrane electrode as a working electrode, and a 0.2 mol L−1 Na2SO4 aqueous solution as an electrolyte. Catalyst membrane electrode: 10 mg of photocatalyst was weighed and mixed with 0.2 mL of ethanol, 0.7375 mL of water, and 0.0625 mL of 5% Nafion solution and sonicated for at least 30 min, Next, using a micropipette, 10 μL of the combined solution was applied to a 4 cm2 conductive glass surface, creating a center area with a volume of 1 cm2, which was then left to dry naturally. The transient photocurrent test potential in the photocurrent test was 0.1 V, and the test time was 540 s with a light–dark cycle of 60 s. Electrochemical impedance frequency range 100 kHz to 0.01 Hz.
2.7 Electron paramagnetic resonance (EPR)
5,5-Dimethyl-1-pyrroline-N-oxide (DMPO) was used as a spin trapping agent and methanol as a hole-sacrificing agent at room temperature and atmospheric pressure. Mix the solution to be tested with 100 mm of capturing agent in a ratio of 1
:
1 to obtain 1 mL of the mixed solution. After full mixing, suck 30 μL with a capillary glass tube and put it into the EPR-specific test tube. Record the spectral signal in dark and light conditions for 15 minutes.
3 Results and discussion
3.1 Characterization of physical phases and micromorphology
Fig. 1(a–c) shows the XRD spectra of the samples, the bright diffraction peaks of the samples indicate that all the samples are well crystallized. There are two distinct diffraction peaks at 13.05° and 27.34° for g-C3N4, corresponding to the (100) and (002) crystal planes of the graphite-like phase, respectively, which are attributed to the in-plane stacking and interlayer stacking of the typical triazine ring in g-C3N4 (ref. 22) (JCPDS 87-1526), The stronger diffraction peak crystal spacing is typically 0.326 nm. The diffraction peaks of Ce-BTC were 8.5°, 10.6°, and 18.1°, respectively, and the XRD data were consistent with the previous report23 (CCDC ID: 773084), in which the peaks of CeCu-BTC and Ce-BTC remained consistent. It is noteworthy that a part of CeO2 was generated in the samples under hydrothermal conditions, with diffraction peaks of 28.55°, 33.09°, and 47.49°, corresponding to the (111), (200), and (220) crystal planes of CeO2, respectively. The Cu-BTC diffraction peaks were 6.7°, 9.5°, 11.6°, 13.5°, and 19.3°, respectively. Some of the synthesised samples contain very small amounts of copper oxide impurities, and the diffraction peaks at 35.5° and 38.9° correspond to the (111) and (200) crystal planes of CuO, respectively.19,20 Due to the difference between the ion radii of Ce3+ (1.02 A) and Cu2+ (0.73 A), the doping of Cu causes the XRD pattern to move in a higher 2θ direction, indicating that the nodes of the Ce3+ skeleton are partially and isomorphically replaced by Cu2+ ions to form CeCu-BTC. With the increasing amount of Cu doping in the heterojunction, the corresponding (101) diffraction peak intensity gradually decreases, the full width of the half-peak increases, and the grain size gradually decreases, indicating that the crystal growth process of Ce-BTC is hindered by the high content of Cu,24 and the refined grain size also helps to increase the specific surface area of the composite photocatalyst, which in turn improves its photocatalytic performance.
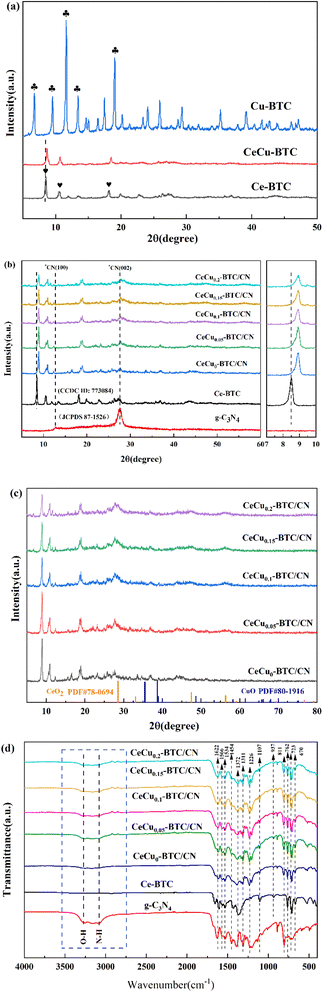 |
| Fig. 1 XRD patterns of Ce-BTC, Cu-BTC and CeCu-BTC (a), g-C3N4, Ce-BTC and CeCux-BTC/CN (b), XRD spectra of CeO2 and CuO (c), FT-IR spectra of g-C3N4, Ce-BTC and CeCux-BTC/CN (d). | |
Fig. 1(d) shows the FT-IR spectra of the samples, and all CeCux-BTC/CN samples show almost the same absorption bands, and the broad absorption bands at 3500–2820 cm−1 can be attributed to the bonded or physically adsorbed hydroxyl (–OH) stretching vibrations of the bonded or physically adsorbed water molecules and the N–H bond stretching vibrations.25 In the characteristic frequency region, the absorption bands at 1625–1534 cm−1 and 1454–1372 cm−1 belong to the asymmetric stretching vibration (vas) and symmetric stretching vibration (vs) of the carboxyl group (O–C
O) in homobenzoic acid,26,27 respectively. In the fingerprint region, the absorption bands at 1300–1000 cm−1 belong to C–O stretching vibration, the absorption band at 1107 cm−1 corresponds to C–H in-plane bending vibration, and the absorption peak at 810 cm−1 corresponds to the out-of-plane bending vibration of the heptane-triazole ring in g-C3N4. It is also found that the absorption intensity of the peak at this location gradually increases with the increase of Cu content in the heterojunction. The absorption bands at 767 and 721 cm−1 correspond to C–C ring out-of-plane bending vibrations, which confirm the aromatic ring structure of the BTC ligand.28 The absorption band between 700 and 450 cm−1 can be attributed to the Ce–O stretching vibration of Ce-BTC samples.
By comparison, it can be seen that the organic ligand BTC has formed a bridge structure with rare earth ions and Cu ions. For the offset phenomenon of some absorption peaks, it also shows that the compound of BTC and g-C3N4 has a significant impact on the chemical environment of the g-C3N4 molecule, which fully shows that the two have successfully constructed a heterojunction, which is consistent with the XRD analysis results.
Fig. 2 is the SEM image of the sample. Fig. 2(a) shows that g-C3N4 presents a clearly visible irregular two-dimensional folded layered structure, and the adjacent thin sections may show a good pore structure.29 Ce-BTC in Fig. 2(b) shows a typical rod- or bar-like structure with different sizes and lengths, mostly around 15–20 μm, presenting a smooth and textured surface, and the crystal edges can be clearly seen. Fig. 2(c) shows the tight and ordered stacking of Ce-BTC and g-C3N4 cladding after the sonicated g-C3N4 structure has been dispersed. Some surface aggregates of copper oxide with microspherical structure and micron flower-like g-C3N4 are shown in Fig. 2(d). In addition, when the Cu content was further increased, such as in CeCu0.1-BTC/CN samples, more Cu-BTC particles appeared (Fig. 2(h)), but the high content of Cu caused the crystal growth of Ce-BTC to be hindered, which might lead to a corresponding decrease in the particle size of Cu-BTC (Fig. 2e–g).30 To sum up, Cu-BTC and g-C3N4 are evenly dispersed in the structure of Ce-BTC to form a relatively complex structure. It is inferred that heterostructures may be formed and play a certain role in promoting the separation of charges in photoexcitation.31
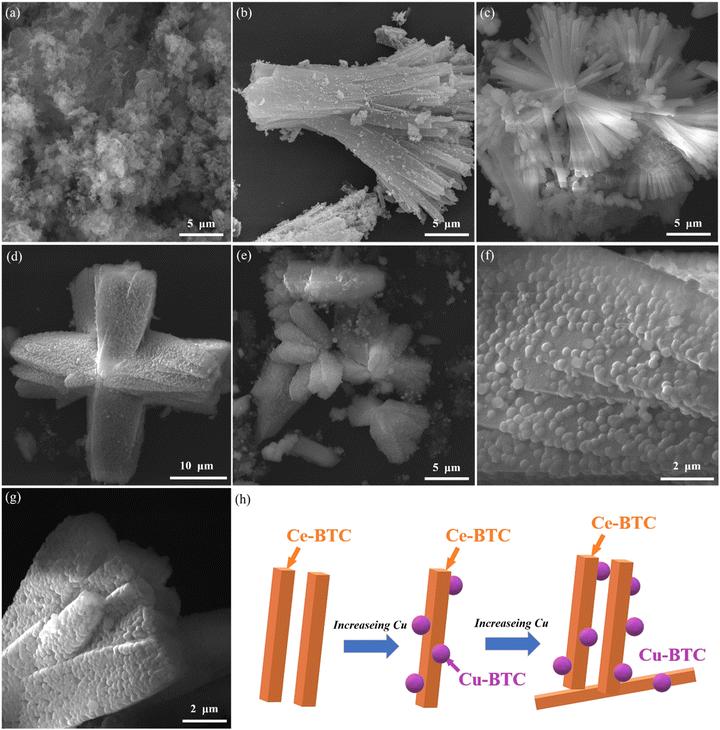 |
| Fig. 2 SEM images of g-C3N4 (a), Ce-BTC (b), CeCu0-BTC/CN (c), CeCu0.05-BTC/CN (d), CeCu0.1-BTC/CN (e), CeCu0.05-BTC/CN sample enlarged view (f), CeCu0.1-BTC/CN sample enlarged view (g), schematic diagram of sample crystal growth (h). | |
Fig. 3 shows the TEM image of the sample, and Fig. 3(a) shows that some coalesced spherical Cu-BTC and rod-shaped Ce-BTC are uniformly distributed on the smooth carbon nitride surface and are in close contact on the surface of g-C3N4, Further, it is clearly proved that Cu in Ce-BTC is successfully doped and coexists with g-C3N4, forming a more complex structure, which proves that the sample is successfully compounded. As reported in previous studies, g-C3N4, with its functional amino groups and large specific surface area, is also a good carrier for loading nanocatalysts, which is more favorable for the construction of heterojunction32,33 structure. Thus, in summary, it was confirmed that the CeCux-BTC/CN composite heterojunction was successfully constructed, and the composition of this heterojunction may be conducive to the rapid transfer of photoexcited electrons at the interface,34 which may improve charge migration and photocatalytic efficiency. Fig. 3(b and c) clearly show lattice spacings of 0.32, 0.31, 0.19, and 0.21 nm corresponding to the (002) crystallographic plane of g-C3N4, the (111) and (220) crystallographic planes of CeO2, and the (200) crystallographic plane of CuO grains, with well-matched lattice planes and good agreement with the XRD data. Fig. 3(d) shows its dark field image. By collecting scattered (diffracted) electronic signals for imaging, it can be seen that the larger and thicker the sample, the stronger the scattering and the brighter the sample area in the dark field. Fig. 3(e–h) clearly shows the elemental distribution of the samples, with brighter points in the elemental mapping region indicating higher concentrations of the matched elements. It is worth noting that the distribution of C, N, O, Ce, and Cu elements is relatively uniform. The total distribution of each element is shown in Fig. 3(i), which indicates the good coexistence of Ce-BTC, Cu-BTC, and g-C3N4, and it also indicates that the structure of the prepared samples is well dispersed and thermally stable, and it also proves once again that the samples are successful in constructing heterojunctions.
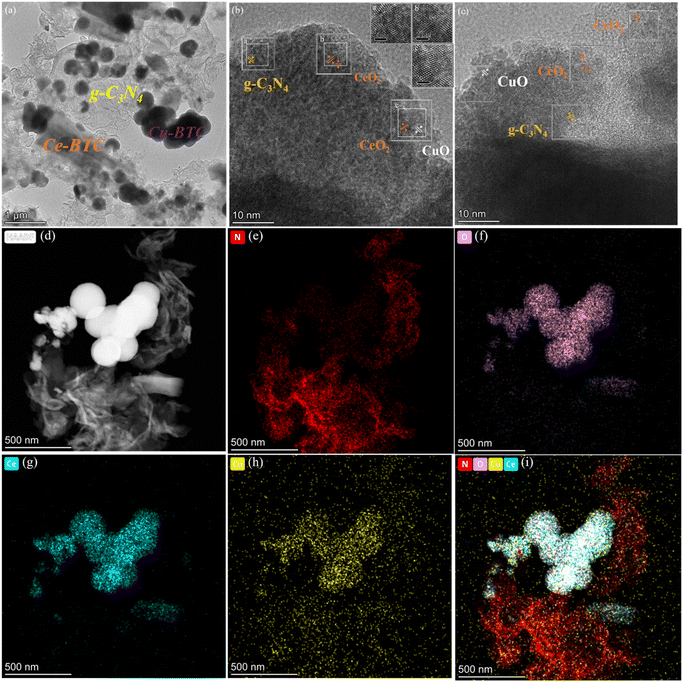 |
| Fig. 3 TEM (HR-TEM) (a–c) of CeCu0.05-BTC/CN, dark field image of CeCu0.05-BTC/CN (d), EDS mapping image: N (e), O (f), Ce (g), Cu (h), general diagram of element distribution (i). | |
Fig. 4 shows the surface element and chemical valence composition of g-C3N4, CeCu-BTC, and CeCu0.05-BTC/CN. Pollution carbon (284.8 eV) was selected as the internal standard for all elements in the figure, and the spectral correction has been completed. Fig. 4(a) shows the full spectrum of the sample. It is found that there are mainly energy spectrum peaks related to C 1s, N 1s, and O 1s elements in g-C3N4. CeCu-BTC and CeCu0.05-BTC/CN mainly have energy spectrum peaks related to C 1s, N 1s, O 1s, Ce 3d, and Cu 2p elements without other impurities, which corresponds to XRD data. The element content in CeCu0.05-BTC/CN also indicates that g-C3N4 and CeCux-BTC are successfully combined.
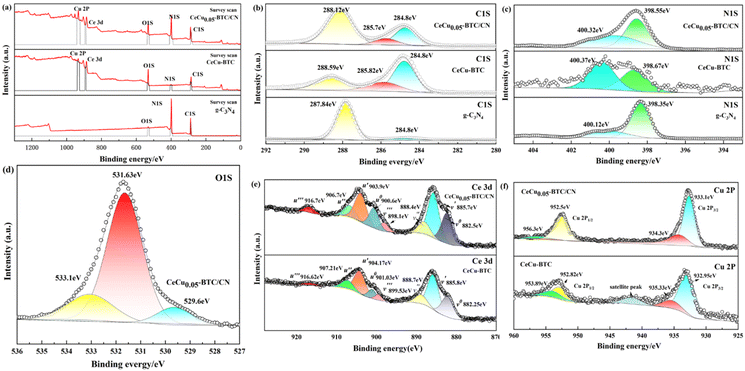 |
| Fig. 4 XPS spectra of the g-C3N4, CeCu-BTC and CeCu0.05-BTC/CN: total elemental spectra (a), C 1s (b), N 1s (c) O 1s (d), Ce 3d (e), Cu 2p (f). | |
As shown in Fig. 4(b), the C 1s spectrum is deconvoluted into three characteristic peaks, of which the characteristic peak of 284.8 eV corresponds to the amorphous carbon (C–C) of the sample, and the characteristic peaks at 285.7 eV and 288.12 eV correspond to C–O–C and C–C
O35,36 respectively. The characteristic peak at 287.84 eV corresponds to the characteristic peak of SP2 hybridized carbon in g-C3N4 (N–C
N),19 The peak is shifted by 0.28 eV to reach 288.12 eV at high binding energy in the composite samples, suggesting that there is a change in charge transport or chemical environment at the surface of the triazine ring, which may be related to the introduction of CeCux-BTC, and also indicates that the density of the electron cloud around the Ce and Cu atoms is increased. Fig. 4(c) shows that the peak of the N 1s energy level appears at the C–N binding energy of 398.35 eV, which corresponds to the pyridine nitrogen in the tricycle (C
N–C) shown in Fig. 4(d) at binding energies of 529.6 eV, 531.63 eV, and 533.1 eV, respectively, can be classified into three component peaks: lattice oxygen (Olat), surface chemisorbed oxygen (OAds), and surface reactive oxygen (OSur), which correspond positively to C
O and C–O.19,36 Among them, surface active oxygen (OSur) is mainly classified as metal oxide-bonded (Cu–O or Ce–O), which is derived from oxygen defects with lower coordination properties or weaker surface oxygen bonding and possesses better oxidative activity against toluene at low temperatures,37,38 so it is speculated that the fabricated samples may possess better catalytic activity. As an example, Ce 3d of CeCu0.05-BTC/CN in Fig. 4(e). The Ce 3d spectrum is deconvoluted into two spin–orbit peaks v and u and assigned to Ce 3d3/2 vs. Ce 3d5/2. Notate v0 (882.5 eV), v′ (885.7 eV), v′′ (888.4 eV), v′′′ (898.1 eV), u0 (900.6 eV), u′ (903.9 eV), u′′ (906.7 eV), u′′′ (916.7 eV). The four characteristic peaks u0, u′, u′′, and u′′′ are attributed to Ce 3d3/2 spectral peaks, and v0, v′, v′′, and v′′′ are attributed to Ce 3d5/2 spectral peaks. u0, u′′, and u′′′ with v0, v′′, and v′′′ can be attributed to Ce4+, and the remaining two characteristic peaks correspond to Ce3+.20,30,38 Therefore, Ce3+ and Ce4+ coexisted on the surface of this sample, and it was hypothesized that the abundant photocatalytic properties could also be attributed to the renewable conversion between Ce3+ and Ce4+. In Fig. 4(f), the Cu 2p spectrum is deconvoluted into two spin orbital peaks, Cu 2p3/2 and Cu 2p1/2, corresponding to binding energies of 933.1, 934.3 eV, and 952.5, 956.3 eV (ref. 19 and 20) respectively. The Cu element in CeCux-BTC mainly exists in the form of Cu+ and Cu2+, while the peaks of Ce 3d and Cu 2p are shifted in the direction of low binding energy, which indicates that there are interactions and electron transfer between CeCu-BTC and g-C3N4. It indicates that the photogenerated electrons on the g-C3N4 surface are transferred to CeCu-BTC, and the electron–hole pair complex is effectively suppressed. This is in agreement with the XRD and TEM data.
Fig. 5(a and b) shows the UV-Vis absorption spectra of the samples in the range of 250–800 nm, which were calculated using the Tauc plot algorithm and the energy band eqn (1).
where
α is the absorbance index,
n is determined by the type of optical jump within the semiconductor,
v is the frequency,
h is Planck's constant (4.1356676969 × 10
−15), and
Eg is the semiconductor bandgap width. Apparently, CeCu
0.05-BTC/CN shows a significant enhancement of visible light absorption compared to g-C
3N
4, while the presence of heterojunction results in red-shifted absorption bands, suggesting that the sample is capable of attracting more visible-light photons in the UV and visible ranges to obtain the active e
−/h
+, which subsequently improves the photocatalytic performance. As shown in
Fig. 5(a and b), the visible absorption band edges of g-C
3N
4, CeCu-BTC and, CeCu
0.05-BTC/CN are about 455 nm, 534 nm, and 509 nm, which is in agreement with earlier reports.
39 The optical band gap energy (
Eg) valuations of 2.72, 2.32, and 2.44 eV for g-C
3N
4, CeCu-BTC, and CeCu
0.05-BTC/CN, respectively, were deduced from
eqn (1). The results show that the introduction of Cu narrows the optical band gap value of CeCu
0.05-BTC/CN composites, and the lower
Eg values indicate that the samples may produce more photogenerated carriers and possess higher visible light trapping ability.
34
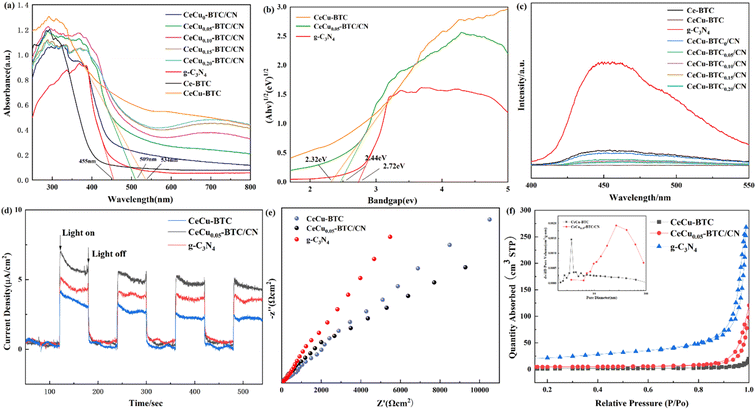 |
| Fig. 5 UV-Vis absorption spectrum (a) and Tauc curve (b), fluorescence spectrum (c), transient photocurrent response spectrum (d), Nyquist plots (e) of the sample, N2 adsorption and desorption isothermal curves and pore size distribution curves (f). | |
Fig. 5(c) shows the separation of photogenerated carriers generated at the contact interface of different samples at the 315 nm excitation wavelength. The stronger the PL peak, the higher the e−/h+ recombination rate, and the lower the corresponding separation efficiency, which is more conducive to the photocatalytic reaction. This is also one of the reasons why the original g-C3N4 has low capture ability for visible light and is easy to compound. CeCu-BTC has no fluorescence in this band, which also shows that the introduction of the metal organic framework helps to reduce the carrier recombination. At the same time, it is speculated that the phenomenon of improving the photoelectron separation efficiency and reducing the recombination rate should also be related to the synergistic effect between g-C3N4 and Cu doping in CeCu-BTC and Ce.30 In summary, the construction of this heterojunction not only enhances the photoelectron transport capacity but also effectively suppresses the recombination rate and, at the same time, has a contributing effect on the improvement of photocatalytic performance.40,41
Fig. 5(d) shows the transient current response of CeCu-BTC, CeCu0.05-BTC/CN, and g-C3N4. The intensity of the photocurrent reflects the separation efficiency of photogenerated electrons and holes.42 The transient photocurrent intensity of CeCu0.05-BTC/CN was significantly higher than that of CeCu-BTC, and g-C3N4, showing a periodic and stable reciprocating law, indicating that the carrier separation efficiency in the synthesized heterostructure is higher and the photocatalytic reaction is more efficient. The radius of the arc area in the EIS Nyquist plot shown in Fig. 5(e) indicates the resistance to the transfer of charge on the surface of the semiconductor electrode.43 With the decrease in semicircular radius, the generation efficiency of electron hole pairs increases. It is observed that the arc radius of CeCu0.05-BTC/CN Nyquist is smaller than that of other samples, which indicates that the former has a smaller transmission impedance and lower resistance to carrier separation, which is more conducive to charge transfer. Fig. 5(f) shows the N2 adsorption–desorption experiments of the samples at 77 K. The g-C3N4, CeCu-BTC, and CeCu0.05-BTC/CN were found to be H3-type hysteresis loops with type IV adsorption isotherms. When the sample is at P/P0 >0.8, the hysteresis loop may be due to the adsorption of N2 in the slit pores of MOFs, and the H3 hysteresis loop is mainly found in the aggregates of layered structure, so it is speculated that it may be the intermolecular graphite-like structure of g-C3N4. This type of hysteresis loop also shows that most of the materials have a mesoporous structure and the pore distribution is uneven. The specific surface area, pore volume, and average pore size of different samples can be obtained in Table 1. It is found that the specific surface area and average pore size of CeCu0.05-BTC/CN are significantly increased compared with CeCu-BTC. It is inferred that the doping of Cu and the recombination of g-C3N4 may lead to more defects and affect the growth of crystals, which is also consistent with the results of electron microscopy. At the same time, we infer that a more porous structure and relatively large specific surface area also provide more active sites and the possibility of reagent adsorption for the reaction, thereby enhancing the photocatalytic ability of the sample.
Table 1 Comparison of SBET, pore volume and average pore size
Sample |
SBET (m2 g−1) |
Pore volume (cm3 g−1) |
Average pore size (nm) |
g-C3N4 |
73.87 |
0.41 |
22.40 |
CeCu-BTC |
4.4905 |
0.03 |
26.04 |
CeCu0.05-BTC/CN |
16.510 |
0.13 |
35.53 |
3.2 Photocatalytic carbon dioxide reduction
Fig. 6 shows the photocatalytic reduction test of carbon dioxide under simulated sunlight. g-C3N4 and CeCux-BTC/CN products are both CO and CH4, where CO is designated as the main product, accompanied by a small amount of methane production. The photocatalytic carbon dioxide reduction experiments were carried out under gas–solid phase conditions with 1 mL of pure water as the proton-providing source and no sacrificial agent, and the reaction mechanism of the different intermediates produced by participating in the carbon dioxide reduction can be represented by the following reaction equations.44
2CO2 + 4e− + 4h+ → 2CO + O2 |
CO + 6e− + 6H+ → CH4 + H2O |
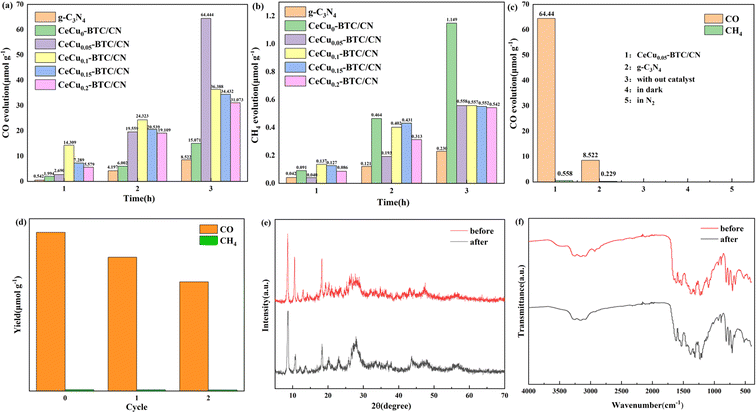 |
| Fig. 6 Photocatalytic CO2 reduction CO yield plot (a), CH4 yield plot (b), control experiment (c), CeCu0.05-BTC/CN cycle test (d), XRD and FT-IR changes of the samples before and after illumination (e and f). | |
For the original g-C3N4, the CO yield is only 8.522 μmol g−1 and the CH4 yield is 0.232 μmol g−1 under this condition, which may be due to the higher charge-carrier recombination rate and rapid recombination. As can be seen in Fig. 6(a), the CO and CH4 reduction capacities of CeCux-BTC/CN, which successfully constitutes the heterostructure, are both higher than those of g-C3N4. Among them, CeCu0.05-BTC/CN CO yield was 64.44 μmol g−1, which was 7.56 times of g-C3N4, and the CO yields of other samples were 15.07, 36.39, 34.43, and 31.07 μmol g−1 (x = 0, 0.1, 0.15.0.2), which were 1.77, 4.27, 4.04, and 3.65 times of g-C3N4, respectively. The yield of the heterostructure CeCu0.05-BTC/CN shown in Fig. 6(b) was 0.5575 μmol g−1, which was 2.42 times higher than that of g-C3N4, and the CH4 yields of the other samples were 1.149, 0.557, 0.552, and 0.542 μmol g−1 (x = 0, 0.1, 0.15, 0.2), which were 5 times higher than that of g-C3N4, respectively, 2.42, 2.4, and 2.36 times that of g-C3N4, respectively. It also shows that the content of Cu doping is the key to realizing the high photocatalytic activity of the composite heterostructure material. The suitable Cu content makes it well dispersed on Ce-BTC and g-C3N4 surfaces, which facilitates carrier transfer and separation.45,46 In addition, when the content of Cu was higher than 5.0 wt%, a further increase in the proportion of Cu led to a decrease in the photocatalytic CO2 reduction performance.
Fig. 6(c) shows that in the absence of a photocatalyst, light source, or N2 atmosphere instead of CO2, the reaction did not produce CO and CH4, indicating that CO2 was the only carbon source involved in the reduction reaction, with no other factors influencing it. Fig. 6(d) shows the cycling test of sample CeCu0.05-BTC/CN, in which the CO and CH4 yields were found to remain good after centrifugal recovery and activation several times. In addition, the recovered XRD spectra (Fig. 6(e)) and FT-IR spectra (Fig. 6(f)) also proved that the main crystal structure and chemical functional groups had no obvious change after the stability test, indicating that it had good photocatalytic stability.
3.3 Research on photocatalytic mechanism
To further explain the sample mechanism, electron paramagnetic resonance (EPR) was used to analyze the direction of electron transfer in the system. In Fig. 7(a and b), DMPO-˙OH was not seen in any of the dark reaction conditions, and CeCu0.0.5-BTC and CeCu0.0.5-BTC/CN were observed in the light reaction, showing a standard fourfold peak and four peaks equidistant with peak heights of 1
:
2
:
2
:
1. The larger DMPO-˙OH may be attributed to its VB potential to be corrected, that is, the VB potential of CeCu-BTC and CeCu0.05-BTC/CN should be greater than 1.99 eV (ref. 47) (OH−/–OH 1.99 eV). On the contrary, DMPO-˙OH was not found in g-C3N4, presumably due to the weak oxidation potential of the photogenerated holes, which also suggests that the photogenerated holes remain in the VB of CeCu-BTC and are not transferred to the VB of g-C3N4. According to the linear extrapolation results, the EVB-XPS of g-C3N4 and CeCu-BTC in Fig. 7(c and d) are 1.65 and 2.03 eV, respectively.
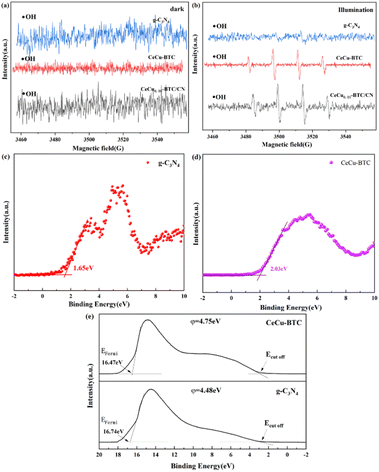 |
| Fig. 7 EPR spectra of DMPO-˙OH spin capture (a and b), VB-XPS (c and d), UV electron spectroscopy UPS (e). | |
In order to obtain the actual VB potential of the samples more accurately and efficiently, the samples were estimated according to eqn (2) and (3). φ is the work function of the XPS instrument, with a value of 4.2 eV, and the NHE at pH = 7 is 4.44 eV. As a result, the values of EVB-NHE for g-C3N4 and CeCu-BTC are obtained as 1.41 and 1.79 eV. Eqn (3) yields ECB's of −1.31 and −0.53 eV for g-C3N4 and CeCu-BTC, respectively.
|
EVB-NHE = φ + EVB-XPS − 4.44
| (2) |
Finally, to verify the accuracy of the above potential calculations, we also utilised the semiconductor electronegativity theory (shown in eqn (4)) to calculate the VB potential. X is the geometric mean of the absolute electronegativity of the atoms within the semiconductor, and Ec is a constant with respect to the standard H electrode, generally taken to be 4.5 eV, with X for g-C3N4 being 4.73 eV,48 as an example. The EVB of g-C3N4 is 1.58ev and the ECB is −1.14. Meanwhile, the results obtained by the two calculation methods were found to be approximate, and the semiconductor electronegativity theory was further used for the explanation of the photocatalytic mechanism.
In addition, Fig. 7(e) shows the ups spectrum of the sample. The work function (φ) of the sample is obtained through formula (5), and ΔE is the distance from the upper starting point to the cut-off edge of the secondary electron after the bias voltage is applied. The φ of g-C3N4 and CeCu-BTC are 16.74 and 16.47 eV, respectively. The results indicate that CeCu-BTC has a larger φ value and a relatively small Fermi energy level, while the opposite is true for g-C3N4. Therefore, when g-C3N4 and CeCu-BTC are in close contact to form a CeCu-BTC/CN heterojunction, the work function difference between the two samples causes the free electrons of CN to spontaneously transfer to CeCu-BTC. With the Fermi level of g-C3N4 gradually decreasing, the Fermi level of CeCu-BTC gradually increases until the interface Ef12 reaches equilibrium. Similarly, a space charge region, i.e. an built-in electric field, will be formed at the interface between the two. The existence of the built-in electric field will bend the Fermi level and pull up the energy band of g-C3N4 and lower the energy band of CeCu-BTC. Therefore, the photogenerated electrons of g-C3N4 have to overcome the potential energy barrier to fall back into CeCu-BTC driven by the built-in electric field and interface chemical bonds (Fig. 8(a and b)). Finally, by analyzing the photocatalytic CO2 reduction mechanism diagram of g-C3N4 and the electron paramagnetic resonance (EPR) capture results of hydroxyl radicals (˙OH) shown in Fig. 7(a), And combined with the position where the energy bands of the photocatalysts are located, it is preferred that the photogenerated electrons on the CB of CeCu-BTC will recombine with the photogenerated holes of the VB of g-C3N4, which also verifies the successful construction of the S-type heterojunction.
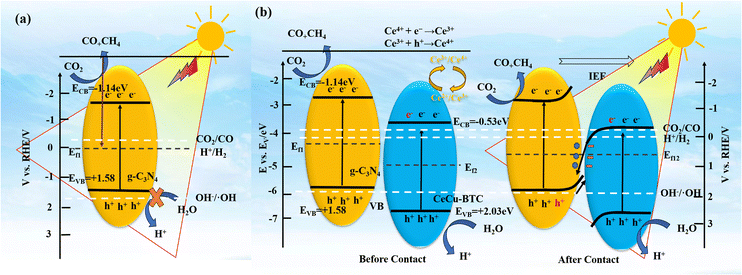 |
| Fig. 8 Photocatalytic mechanism diagram of g-C3N4 (a), the work functions of CN and CeCu-BTC before/after contact and the formation of internal electric field (b). | |
The above conclusions indicate that CeCu-BTC and CN change from a steady state to an excited state upon irradiation with visible light larger than the bandgap of both, producing photogenerated electrons and holes. Due to the interaction of IEF and Coulomb at the interface, the photogenerated electrons on the CB of CeCu-BTC will combine with the photogenerated holes on the VB of g-C3N4, and then the photogenerated electrons accumulated on the CB of g-C3N4 will react with the CO2 adsorbed on the surface of the catalyst to form CO and CH4. Meanwhile, the CB potential of CeCu-BTC and g-C3N4 is more negative than that of Cr(VI)/Cr(III) (+0.51 EV vs. NHE), which indicates that the reduction of adsorbed Cr(VI) to Cr(III) is thermodynamically beneficial to the CB of the photocatalyst. The coexistence of Ce3+ and Ce4+ can also aid in the transfer of electrons from the catalyst surface, thus prolonging the reaction time between electrons and carbon dioxide.
4 Conclusions
In summary, The S-type heterojunction photocatalysts (CeCux-BTC/CN) with cerium-copper bimetallic organic framework (CeCux-BTC) and graphite-phase carbon nitride (g-C3N4) were constructed by a simple solvothermal method for photocatalytic CO2 reduction to CO and CH4. The results show that the built-in electric field constructed by Fermi energy level flattening transfers the electrons in an S-type manner, which not only preserves the strong reducing properties of the electrons in the material but also provides the maximum redox capacity and enables the composite samples to obtain higher visible-light trapping capacity and improve the separation efficiency of the carriers while refining the crystal particles. CeCu0.05-BTC/CN possessed the optimal photocatalytic performance with the addition of only 1 mL of H2O as the proton provision source. The CO and CH4 yields were 64.44 μmol g−1 and 0.5575 μmol g−1, which were 7.56 and 2.42 times higher than that of g-C3N4, respectively, and the catalytic performance was basically stable after the cyclic test, and this heterojunction also paved the way for a small amount of low-cost composite modification of g-C3N4.
Data availability
Crystallographic data for g-C3N4,Ce-BTC, have been deposited at the JCPDS/CCDC under JCPDS 87-1526, CCDC ID: 773084 and can be obtained from http://dx.doi.org/10.1002/smll.202003597, https://doi.org/10.1016/j.matchemphys.2018.04.031, http://dx.doi.org/10.1016/j.apsusc.2017.06.235. The authors confirm that the data supporting the findings of this study are available within the article and/or as its supplementary materials. The data that support the findings of this study are available from the first or corresponding author (Hui Zhang or Rui fen Wang) upon reasonable request.
Author contributions
H. Z. and Y. H. S. completed the sample synthesis and testing. R. F. W., S. L. A., and R. H. G. conceived the idea and accompanied the test and guidance at all stages; H. Z. and Y. H. S. wrote the manuscript; Y. W. M. was responsible for the final review; and all the authors contributed to the manuscript.
Conflicts of interest
The authors declare no competing interests.
Acknowledgements
This work was supported by Basic research business cost project in universities (0406082217), Kundulun District Science and Technology Planning Project (YF2022015), Inner Mongolia Autonomous Region Natural Science Foundation Program (2023QN05040, 2023MS02008).
References
- J. Rockström, W. Steffen and K. Noone, et al., A safe operating space for humanity, Nature, 2009, 461, 472–475 CrossRef PubMed.
- T. Ouyang, H. J. Wang and H. H. Huang, et al., Dinuclear Metal Synergistic Catalysis Boosts Photochemical CO2-to-CO Conversion, Angew Chem. Int. Ed. Engl., 2018, 57, 16480–16485 CrossRef CAS PubMed.
- P. Friedlingstein, M. O'Sullivan and M. W. Jones, et al., Global Carbon Budget 2020, Earth Syst. Sci. Data, 2020, 12, 3269–3340 CrossRef.
- W. Jiang, W. Luo and R. Zong, et al., Polyaniline/Carbon Nitride Nanosheets Composite Hydrogel: A Separation-Free and High-Efficient Photocatalyst with 3D Hierarchical Structure, Small, 2016, 12, 4370–4378 CrossRef CAS PubMed.
- J. Zhou, Y. Li and L. Yu, et al., Facile in situ fabrication of Cu2O@Cu metal-semiconductor heterostructured nanorods for efficient visible-light driven CO2 reduction, Chem. Eng. J., 2020, 385, 123940 CrossRef.
- X. Wang, X. Chen and A. Thomas, et al., Metal-Containing Carbon Nitride Compounds: A New Functional Organic–Metal Hybrid Material, Adv. Mater., 2009, 21, 1609–1612 CrossRef CAS.
- W. Liu, J. Shen and Q. Liu, et al., Porous MoP network structure as co-catalyst for H2 evolution over g-C3N4 nanosheets, Appl. Surf. Sci., 2018, 462, 822–830 CrossRef CAS.
- L. Tian, X. Yang and Q. Liu, et al., Anchoring metal-organic framework nanoparticles on graphitic carbon nitrides for solar-driven photocatalytic hydrogen evolution, Appl. Surf. Sci., 2018, 455, 403–409 CrossRef CAS.
- Y. Sun, H. Zhang and Y. Lv, et al., ZIF-8/g-C3N4 photocatalysts: enhancing CO2 reduction through improved adsorption and photocatalytic performance, RSC Adv., 2024, 14, 17498–17506 RSC.
- X. Liu, R. Ma and L. Zhuang, et al., Recent developments of doped g-C3N4 photocatalysts for the degradation of organic pollutants, Crit. Rev. Environ. Sci. Technol., 2021, 51, 751–790 CrossRef CAS.
- Y. Li, M. Zhou and B. Cheng, et al., Recent advances in g-C3N4-based heterojunction photocatalysts, J. Mater. Sci. Technol., 2020, 56, 1–17 CrossRef CAS.
- S. Patnaik, D. P. Sahoo and K. Parida, An overview on Ag modified g-C3N4 based nanostructured materials for energy and environmental applications, Renewable Sustainable Energy Rev., 2018, 82, 1297–1312 CrossRef CAS.
- J. Zhang, F. Guo and X. Wang, An Optimized and General Synthetic Strategy for Fabrication of Polymeric Carbon Nitride Nanoarchitectures, Adv. Funct. Mater., 2013, 23, 3008–3014 CrossRef CAS.
- M. H. Karimi-Harandi, M. Shabani-Nooshabadi and R. Darabi, Simultaneous determination of citalopram and selegiline using an efficient electrochemical sensor based on ZIF-8 decorated with RGO and g-C3N4 in real samples, Anal. Chim. Acta, 2022, 1203, 339662 CrossRef CAS PubMed.
- Y. Wang, C. Zhang and Y. Zeng, et al., Ag and MOFs-derived hollow Co3O4 decorated in the 3D g-C3N4 for creating dual transferring channels of electrons and holes to boost CO2 photoreduction performance, J. Colloid Interface Sci., 2022, 609, 901–909 CrossRef CAS PubMed.
- C. C. Hou and Q. Xu, Metal–Organic Frameworks for Energy, Adv. Energy Mater., 2019, 9(23), 1801307 CrossRef.
- Z. Ma, B. Guan and J. Guo, et al., State of the art and prospectives of heterogeneous photocatalysts based on metal–organic frameworks (MOFs): design, modification strategies, and their applications and mechanisms in photodegradation, water splitting, and CO2 reduction, Catal. Sci. Technol., 2023, 13, 4285–4347 RSC.
- H. Furukawa, K. E. Cordova and M. O Keeffe, et al., The Chemistry and Applications of Metal-Organic Frameworks, Science, 2013, 341, 974 CrossRef CAS PubMed.
- K. Ye, Z. He and F. Wu, et al., Carbon nitride-supported CuCeO2 composites derived from bimetal MOF for efficiently electrocatalytic nitrogen fixation, Int. J. Hydrogen Energy, 2021, 46, 35319–35329 CrossRef CAS.
- X. Zhang, X. Zhang and L. Song, et al., Enhanced catalytic performance for CO oxidation
and preferential CO oxidation over CuO/CeO2 catalysts synthesized from metal organic framework: Effects of preparation methods, Int. J. Hydrogen Energy, 2018, 43, 18279–18288 CrossRef CAS.
- Y. Zhang, X. Zhang and W. Sun, In Situ Carbon-Encapsulated Copper-Doped Cerium Oxide Derived from MOFs for Boosting CO2-to-CH4 Electro-Conversion, ACS Catal., 2023, 13, 1545–1553 CrossRef CAS.
- S. Juntrapirom, S. Anuchai and O. Thongsook, et al., Photocatalytic activity enhancement of g-C3N4/BiOBr in selective transformation of primary amines to imines and its reaction mechanism, Chem. Eng. J., 2020, 394, 124934 CrossRef CAS.
- L. Fan, K. Wang and K. Xu, et al., Structural Isomerism of Two Ce-BTC for Fabricating Pt/CeO2 Nanorods toward Low-Temperature CO Oxidation, Small, 2020, 16, e2003597 CrossRef PubMed.
- S. E. Sivan, K. H. Kang and S. J. Han, et al., Facile MOF-derived one-pot synthetic approach toward Ru single atoms, nanoclusters, and nanoparticles dispersed on CeO2 supports for enhanced ammonia synthesis, J. Catal., 2022, 408, 316–328 CrossRef CAS.
- D. O. Adekoya, M. Tahir and N. A. S. Amin, g-C3N4/(Cu/TiO2) nanocomposite for enhanced photoreduction of CO2 to CH3OH and HCOOH under UV/visible light, J. CO2 Util., 2017, 18, 261–274 CrossRef CAS.
- Q. Liu, C. Zeng and L. Ai, et al., Boosting visible light photoreactivity of photoactive metal-organic framework: Designed plasmonic Z-scheme Ag/AgCl@MIL-53-Fe, Appl. Catal., B, 2018, 224, 38–45 CrossRef CAS.
- G. Zeng, Y. Chen and L. Chen, et al., Hierarchical cerium oxide derived from metal-organic frameworks for high performance supercapacitor electrodes, Electrochim. Acta, 2016, 222, 773–780 CrossRef CAS.
- L. Ai, T. Tian and J. Jiang, Ultrathin Graphene Layers Encapsulating Nickel Nanoparticles Derived Metal–Organic Frameworks for Highly Efficient Electrocatalytic Hydrogen and Oxygen Evolution Reactions, ACS Sustainable Chem. Eng., 2017, 5, 4771–4777 CrossRef CAS.
- D. Pinheiro, K. R. Sunaja Devi and A. Jose, et al., Box–Behnken design and experimental study of ciprofloxacin degradation over Ag2O/CeO2/g-C3N4 nanocomposites, Int. J. Environ. Sci. Technol., 2021, 18, 2303–2324 CrossRef CAS.
- S. Vignesh, S. Suganthi and B. Palanivel, et al., Design a novel g-C3N4 based Ce2O3/CuO ternary photocatalysts for superior photo-degradation performance of organic mixed pollutants: Insights of Z-scheme charge transfer mechanism, J. Phys. Chem. Solids, 2022, 162, 110514 CrossRef CAS.
- S. Balu, S. Velmurugan and S. Palanisamy, et al., Synthesis of α-Fe2O3 decorated g-C3N4/ZnO ternary Z-scheme photocatalyst for degradation of tartrazine dye in aqueous media, J. Taiwan Inst. Chem. Eng., 2019, 99, 258–267 CrossRef CAS.
- D. J. Martin, K. Qiu and S. A. Shevlin, et al., Highly Efficient Photocatalytic H2 Evolution from Water using Visible Light and Structure-Controlled Graphitic Carbon Nitride, Angew. Chem., Int. Ed., 2014, 53, 9240–9245 CrossRef CAS PubMed.
- B. Hu, F. Cai and T. Chen, et al., Hydrothermal Synthesis g-C3N4/Nano-InVO4 Nanocomposites and Enhanced Photocatalytic Activity for Hydrogen Production under Visible Light Irradiation, ACS Appl. Mater. Interfaces, 2015, 7, 18247–18256 CrossRef CAS PubMed.
- A. Kumar, S. K. Sharma and G. Sharma, et al., CeO2/g-C3N4/V2O5 ternary nano hetero-structures decorated with CQDs for enhanced photo-reduction capabilities under different light sources: Dual Z-scheme mechanism, J. Alloys Compd., 2020, 838, 155692 CrossRef CAS.
- H. N. Abdelhamid, G. A. Mahmoud and W. Sharmouk, A cerium-based MOFzyme with multi-enzyme-like activity for the disruption and inhibition of fungal recolonization, J. Mater. Chem. B, 2020, 8, 7548–7556 RSC.
- R. Yang, Z. Li and J. Zhao, et al., The preparation of bimetallic cerium/copper organic framework-derived CeCuOx/CNH3-SCR catalysts, J. Mater. Sci., 2024, 59, 1298–1311 CrossRef CAS.
- H. Lu, Y. Zhou and W. Han, et al., Promoting effect of ZrO2 carrier on activity and thermal stability of CeO2-based oxides catalysts for toluene combustion, Appl. Catal., A, 2013, 464–465, 101–108 CrossRef CAS.
- L. Ai, Y. Luo and W. Huang, et al., Cobalt/cerium-based metal-organic framework composites for enhanced oxygen evolution electrocatalysis, Int. J. Hydrogen Energy, 2022, 47, 12893–12902 CrossRef CAS.
- Y. Tan, Z. Shu and J. Zhou, et al., One-step synthesis of nanostructured g-C3N4/TiO2 composite for highly enhanced visible-light photocatalytic H2 evolution, Appl. Catal., B, 2018, 230, 260–268 CrossRef CAS.
- X. Wei and H. Wang, Preparation of magnetic g-C3N4/Fe3O4/TiO2 photocatalyst for visible light photocatalytic application, J. Alloys Compd., 2018, 763, 844–853 CrossRef CAS.
- L. Biswal, S. Nayak and K. Parida, Recent progress on strategies for the preparation of 2D/2D MXene/g-C3N4 nanocomposites for photocatalytic energy and environmental applications, Catal. Sci. Technol., 2021, 11, 1222–1248 RSC.
- X. Jiao, K. Zheng and L. Liang, et al., Fundamentals and challenges of ultrathin 2D photocatalysts in boosting CO2 photoreduction, Chem. Soc. Rev., 2020, 49, 6592–6604 RSC.
- Y. Hou, A. B. Laursen and J. Zhang, et al., Layered nanojunctions for hydrogen-evolution catalysis, Angew. Chem., Int. Ed., 2013, 52, 3621–3625 CrossRef CAS PubMed.
- O. K. Varghese, M. Paulose and T. J. LaTempa, et al., High-Rate Solar Photocatalytic Conversion of CO2 and Water Vapor to Hydrocarbon Fuels, Nano Lett., 2009, 9, 731–737 CrossRef CAS PubMed.
- Y. Wang, X. Liu and X. Wang, et al., Metal-organic frameworks based photocatalysts: Architecture strategies for efficient solar energy conversion, Chem. Eng. J., 2021, 419, 129459 CrossRef CAS.
- L. Zhang, K. Wong and Z. Chen, et al., AgBr-Ag-Bi2WO6 nanojunction system: A novel and efficient photocatalyst with double visible-light active components, Appl. Catal., A, 2009, 363, 221–229 CrossRef CAS.
- Z. Jiang, W. Wan and H. Li, et al., A Hierarchical Z-Scheme α-Fe2O3/g-C3N4 Hybrid for Enhanced Photocatalytic CO2 Reduction, Adv. Mater., 2018, 30(10), 1706108 CrossRef PubMed.
- Y. Liu, H. Liu and H. Zhou, et al., A Z-scheme mechanism of N-ZnO/g-C3N4 for enhanced H2 evolution and photocatalytic degradation, Appl. Surf. Sci., 2019, 466, 133–140 CrossRef CAS.
|
This journal is © The Royal Society of Chemistry 2025 |
Click here to see how this site uses Cookies. View our privacy policy here.