DOI:
10.1039/D4LP00325J
(Review Article)
RSC Appl. Polym., 2025,
3, 499-531
Polymer electrolytes: evolution, challenges, and future directions for lithium-ion batteries
Received
26th October 2024
, Accepted 20th December 2024
First published on 26th December 2024
Abstract
Electrolytes are indispensable in the field of energy storage and generation. Many types of electrolytes are currently available for various purposes. This review paper explains the various existing polymer electrolytes and the methodologies involved in their preparation. The evolution, history, classification and applications of polymer electrolytes in different fields are reviewed. The properties, like ionic conductivity, electrochemical stability, thermal stability and mechanical strength, needed for an ideal polymer electrolyte are critically explored. Also, the influence of the dielectric constant and temperature over the ionic conductivity of the polymer electrolytes and the necessary properties for an ideal polymer electrolyte are elucidated. In addition, the Arrhenius and Vogel–Tammann–Fulcher ion transport models are demonstrated and analysed to explain the ion conduction mechanisms of polymer electrolytes. Moreover, characterization techniques like electron impedance spectroscopy, transference number measurement and voltammetry for investigating the essential properties of the polymer electrolytes are discussed.
1. Introduction
Batteries are anticipated to be pivotal and inevitable in energy storage applications such as electric vehicles (EVs) and portable electronic devices in the emerging technological world.1 Batteries are generally grouped as primary and secondary. Batteries that are not capable of recharging are said to be primary, and those that could be rechargeable are secondary. Lithium-ion batteries are categorized as secondary batteries, and exhibit multiple benefits like low self-discharge rate, long cycle life, high working voltage, and high energy density.2,3 Conventional lithium-ion batteries (LIB) depend on the Li+ intercalation mechanism4,5 as shown in Fig. 1, with low charge capacity, which is problematic for applications requiring high charge capacities like aerospace applications, electric and hybrid electric vehicles and so on.1,4,5 Conventional lithium-ion batteries have attained an energy density value of around 260 W h kg−1 and are limited, as reported in ref. 2.
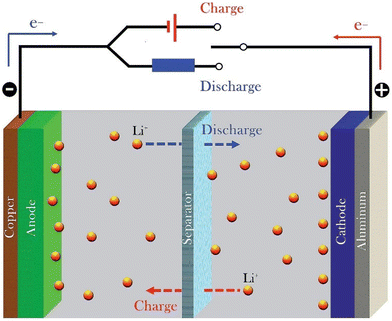 |
| Fig. 1 Schematic mechanism of Li+ ion battery. Reprinted with permission from ref. 7. Copyright Elsevier 2008 (license number: 5673181018376). | |
Therefore, it is necessary to address some crucial drawbacks associated with these traditional lithium-ion batteries for the betterment of technology. Along with the limited energy density, conventional LIBs also have demerits like commercialization concerns, volume expansion, particle surface reconstruction, electrolyte resolution, solid electrolyte interface formation, and phase transition.4,6 Studies suggest that the next generation of lithium-ion batteries with high energy density could be a potential candidate for employment in various applications. Low costs, higher payloads, and lower toxicity will be some of the benefits of these batteries.4
As a matter of an alternative for intercalation anodes, the utilization of graphite to form lithium–metal anodes could avoid energy density problems. However, this would undoubtedly pose a serious safety issue when operated with the conventional liquid electrolyte.8 Moreover, the plating/stripping test confirms the non-uniform distribution of Li+ ions, leading to dendrite formation. Lithium-ion conductive polymer electrolytes (PEs) could be a potential candidate for better energy density and improved safety too.2,9,10 Solid polymer electrolytes can efficiently hamper the development and growth of dendrites. Unlike conventional liquid electrolytes, these polymer electrolytes are not inflammable.11
The polyethene-oxide (PEO)-based system was the primary solid polymer electrolyte (SPE) classification proposed by Armand et al.12 The conductivity of the SPEs generally relies on the crystalline and amorphous phase proportion, where the ion transport occurs at the viscoelastic (amorphous) region.13,14 In the amorphous portion of the polymer, ionic mobility is associated with the polymer motion. Fortunately, the first polymer (PEO) utilized to make PEs was found to be semicrystalline, which contains amorphous and crystalline regions.15,16 The segmental motion of the PE plays a critical role in the ion transport process and helps in obtaining higher conductivity at room temperature, which is a complex phenomenon.17 The selection of polymers relies upon the polar groups with sufficient electron donors, which can coordinate with the cations. Also, polymers should possess low hindrance to bond rotation.18 Some natural polymers that could be used as PEs are chitosan, rice starch, and corn starch.19 Based on modelling and simulation, chitosan based electrolytes possess high proton conductivity. These PEs are less expensive than Nafion (per-fluorinated sulphonic acid membrane), which has been employed for years as a proton exchange membrane in fuel cell technology.20,21 Some of the polymers which may be used as PEs exhibiting high room temperature conductivity are polyethylene oxide (PEO), polyvinyl alcohol (PVA), polyvinyl chloride (PVC), polypropylene glycol (PPG), poly-vinylidene chloride (PVdC).22 The polymer's molecular weight influences the conductivity of the PE, and the conductivity seems to vary with respect to the different salt concentrations. Initially, high molecular weight polymers were used for the SPE system rather than the lower ones.23
Researchers24 reported that single ion-type PEs and polyanionic lithium salts resulted in higher values of lithium transference number. In the field of polymer electrolytes, research has been done to attenuate the crystallinity and glass transition temperature by adding fillers and plasticizers to improve conductivity. These plasticizers contributed to the enhancement of ion transport and DC electrical conductivity by giving up their mechanical properties.25,26 Moreover, adding nanofillers to the polymer matrix can improve the properties,15 and the effect of these fillers on properties still has to be explored. Consequently, some inert fillers were identified as prominent substances to boost these properties.17,27 Also, the addition of clay composites forms composite solid polymer electrolytes (CSPE), which exhibit better properties.28 In PEs, the ion transport mechanism has not yet been fully explored and reported. It depends on various factors like salt concentration, aggregation of ions, dielectric constant of the host polymer matrix, degree of salt dissociation and polymer chain mobility.15,16 Ion transport behaviour and ion/molecular interaction in SPEs can be analysed by dielectric analysis.16,29
To summarize, lithium polymer (Li-pol) batteries are another evolution from lithium-ion batteries. Li-pol batteries use PEs rather than liquid electrolytes, which have a huge impact and many advantages and few disadvantages over lithium-ion batteries, which shall be discussed in the next section. To date, rechargeable batteries with less toxicity and more economic benefits have been found. These batteries are composed of sodium, magnesium and aluminium, which may act as an alternative to lithium-ion batteries (LIBs). These elements are abundant on Earth, and replacement of LIBs by these batteries must be seriously considered.30,31 This review paper explains the types and applications of polymer electrolytes and their significance with respect to lithium-ion batteries. The different types of techniques that are utilized to prepare the PEs are discussed. The necessary properties like ionic conductivity, electrochemical stability window, mechanical properties, etc. are also discussed to understand the properties required for an ideal electrolyte. In particular, the mechanisms to illustrate the ion transport within the PEs are described and analysed using suitable models. In addition, future research directions are also emphasized towards developing high-performance polymer-based electrolytes for the advancement of battery technology.
2. Polymer electrolytes
The field of polymer electrolytes is approximately four decades old. The use of polymers in many applications has recently intensified.32 For safety purposes, PEs have been used to replace conventional liquid electrolytes in energy storage applications,33 and three types of classification are discussed in the later sections. Safety issues like fire, explosion, and electrolyte leakage could occur in liquid electrolytes, which are the crucial drawbacks of Li-ion batteries.34,35 Polymer electrolytes could help to overcome these problems efficiently.33,35 Various benefits of PEs are added resistance to the alterations in the volume of the electrodes during charging/discharging, exceptional flexibility, processability, and improved safety over liquid and inorganic solid electrolytes.7 This section explains the history and evolution of PEs, as well as the classification and various methodologies that are being used to produce PEs.
2.1 History and evolution of polymer electrolytes
The PE concept was first introduced in 1973 by Fenton et al.36 They reported that polyethylene oxide (PEO) when mixed or dissolved with alkali metal salts resulted in conductive complexes.7,37 In 1978, Armand et al.12 introduced a PEO-Li-based solid PE operating at 40 to 50 °C for lithium batteries. It exhibited an ionic conductivity of about 10−4 S cm−1. This SPE was formed by dissolving Li+ salt in PEO, where a coordination bond was established between lithium and ether oxygen in the PEO matrix.7 This PEO-based SPE exhibited high energy density but poor ionic conductivity when compared with lithium batteries made of liquid electrolytes.38 In recent research, high molecular weight PEO has been utilized in PEs, and a particular metal salt was found to control the solubility parameter of the polymer.32 The authors also discovered that the PEO–salt complex was helical, and Chatani et al.39 demonstrated the spatial arrangement of the same using powder X-ray diffraction.
In 1975, gel polymer electrolytes (GPEs) were reported by Feuillade et al.,40 in which the authors added organic plasticizers into the polymer matrix. These GPEs exhibited ionic conductivity of about 10−2 to 10−3 S cm−1. Consequently, this system has limitations like liquid leakage upon squeezing, specific power loss, and specific energy loss due to an inert polymer matrix. The ionic conductivity of GPEs is slightly higher than that of solid PEs, but the mechanical properties are compromised.7 Other than organic plasticizers, incorporating inorganic fillers has contributed to the advancement in mechanical, electrochemical, and transport properties. Skaarup et al.41 used the PEO-LiCF3SO3 as matrix and added the fast ionic conductor particles Li3N as a filler, accomplishing a breakthrough that yielded an electrolyte with higher room temperature ionic conductivity of about 10−3 S cm−1. Wieczorek et al.42 discovered that adding inorganic filler Al2O3 improved the ionic conductivity because of the increased amorphous region, which resulted in higher mobility of ions in PEO-based electrolytes. Weston and Steele17 also found that adding Al2O3 enhanced the conduction, thermal, electrochemical and mechanical properties. The inorganic fillers used are mostly ceramics and hence termed ceramic solid PEs.
The evolution and history of polymer electrolytes from liquid electrolytes in lithium-ion batteries are shown in Fig. 2. For high ionic conductivities, PEs with more amorphous regions are chosen, which are obtained by either disturbing the crystallinity of the polymer or by synthesizing as amorphous electrolytes.43 Sony was the first company to commercialize lithium-ion batteries in 1991. This breakthrough came to the market through the work of Asahi Kasei. In the Goodenough laboratory, scientists discovered that the group of transition metal oxides of NaFeO2 structure along with the lithium salts, at relatively high potentials exhibited deintercalation and intercalation of cations (lithium ions). LiCoO2 was used as the positive electrode of the battery, which was patented in 1979.38,44 As mentioned earlier, due to various problems and research progress, PEs replaced liquid electrolytes eventually. In comparison with liquid electrolytes, PEs have definite advantages like low flammability, easy processability, resistance to dendrite formation, good electrode/electrolyte interfacial contact and compatibility, and endurance to vibration, mechanical deformation and shock.7,45 The stipulations for a polymer to be an electrolyte are high ionic conductivity (close to 10−4 S cm−1 at room temperature), higher electronic resistance, optimum Li-ion transference number, good mechanical strength, good thermal stability, low interfacial resistance, high electrochemical stability, low cost and ease of synthesis.33,46,47
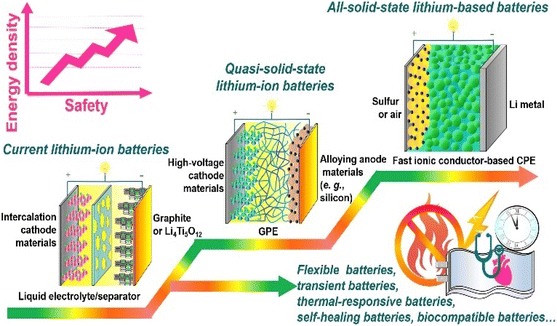 |
| Fig. 2 History and evolution of polymer electrolytes. Reprinted with permission from ref. 33. Copyright Elsevier 2019 (license number: 5673190287905). | |
2.2 Classification of polymer electrolytes
Polymer electrolytes are alternatives to liquid electrolytes with significant advantages, which contributed to the discovery of Li-pol batteries and are used in many electrochromic devices.48 There are various predecessors like ionic plastic crystal electrolytes, nanostructured liquid crystal electrolytes, inorganic solid electrolytes, etc. In liquid crystal (LC) PEs, thermotropic LCs, which are carbonate-based LCs, are generally used.49 Liquid crystal PEs exhibit low conductivity of about 10−6 to 10−8 S cm−1, and a few other similar electrolytes with good conductivities have also been reported.50
Through continuous research and diligent efforts, PEs with enhanced properties have been produced. These PEs are categorized into dry solid PEs, gel/plasticized PEs and composite PEs, as shown in Fig. 3.19 This classification explains the evolution and application of several PEs in energy storage applications; we will discuss them with regards to battery applications in particular. These PEs are made with enhanced/necessary properties by utilizing various methods/techniques like cross-linking, adding fillers/plasticizers, copolymerization, blending, etc., which make them suitable as electrolytes. Nowadays, various categories of PEs are available, but this review paper establishes a strong foundation on fundamental classifications to understand the concepts of PEs clearly. As well as the classification of polymer electrolytes, some of the critical ways to improve the properties (especially conductivity) are also discussed in the following sections accordingly.
 |
| Fig. 3 Classification of polymer electrolytes and their properties. | |
Dry solid polymer electrolytes.
Dry solid polymer electrolytes (DSPEs) are also known as solvent-free or polymer–salt complexes in which organic liquid is not used.19 Thus, a polar (functional) polymer mixed with inorganic salts enhances the polymer's ionic conductivity, and is called the dry solid polymer electrolyte. Here, the synergism between the polymer and metal ions results from electrostatic forces and coordination bonds.48 The PEO-based electrolyte was the first solid PE reported, but this SPE system still needs to be improved due to its poor conductivity at room temperature.19 The behaviour of the functional groups embedded in the polymer matrix, molecular weight, degree of branching, crystallinity, the space between functional groups, nature and charge of metal cation, and nature and charge of anions are the elements affecting the interaction between the polymer and metal cation.48
Some of the conditions33 required for a polymer matrix to be a solid PE are:
(a) Good cation solvation nature for dissociation of cations in host polymer.
(b) High dielectric constant for high concentration of charge carriers.
(c) High backbone flexibility to reduce the bond rotation energy barrier, enhancing the polymer chains’ segmental mobility.
(d) High molecular weight to get good mechanical strength.
PEO-based SPEs were found to exhibit a room temperature conductivity of around 10−6 S cm−1.51 Nanofillers like TiO2 improved the conductivity to 10−5 S cm−1 at 30 °C and 10−4 at 50 °C. Wang et al.52 demonstrated that by maintaining a temperature of about 40 to 60 °C, the SPE system PEO-LiClO4-TiO2 exhibited enough ionic conductivity (10−4 S cm−1). However, the complete dissociation of salts in the PEO matrix is unattainable due to ion aggregation. Since carbonates are generally used in liquid electrolytes to improve the dielectric constant,53 researchers thought of using carbonate-functionalized polymers, which could lead to high ionically conductive and electrochemically stable SPEs.54 Cellulose-supported polypropylene carbonate-based SPE was prepared by Zhang et al.55 which was found to possess high ionic conductivity of about 3 × 10−4 S cm−1 at 20 °C for lithium-ion batteries. Polyester-based SPEs are believed to possess low Tg and high dielectric constant. Even though they showed high electrochemical stability, the conductivity seems to be lower than the conventional PEO-based SPEs.33
Therefore, blending is generally done in PEs because blending is the most feasible technique as it helps in improving the electrical and physical properties of the PEs.57Fig. 4 shows the schematic representation of polymer blend electrolytes made by poly solvent and polyanion. Solvent casting is the most preferred technique for this blending method. XRD studies have proved that the enhanced conductivity of this blended PE is because of its inferior crystalline portion.19,58 These blended PEs were used in applications like electrochromic devices and lithium polymer batteries.59 For example: PMMA-PVC blends were developed in which 70% PMMA was blended with 30% PVC. This blend was made as a thin film and exhibited an ion-hopping mechanism explained by the Arrhenius model.57 In other research, attempts were made to blend the linear PEO and a hyperbranched polymer (i.e., poly-[bis(triethylene glycol)benzoate]) mixed with LiN(CF3SO2)2, which seemed to improve the conductivity of ions and interfacial resistance of lithium/electrolyte when compared with PEO-based PEs.19
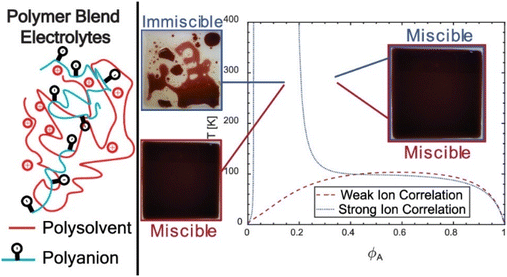 |
| Fig. 4 Blending of polymer electrolytes and their impacts. Reused with permission from ref. 56. Copyright 2021 American Chemical Society. | |
Sometimes, co-polymerization has been utilized in DSPEs to get wide range of properties. Here, co-polymerization disturbs the order/crystalline phase of the homopolymer, which in turn enhances the conductivity.60 Panday et al.61,62 prepared PS-PEO co-polymer in which PEO acted as a conductive channel and PS provided mechanical strength. Here, the conductivity of the co-polymer electrolyte depended on the molecular weight of PEO. As the degree of salt dissociation is high in PEO rather than the interface of the co-polymers, the increase in molecular weight of PEO enhanced the ionic conductivity. Gomez et al.63 also found that the molecular weight increase of PEO boosted the ionic conductivity, as there are more ether oxygens available for ion transport. Nevertheless, crosslinking of polymer is employed to enhance the conductivity and mechanical properties of polymer electrolytes in some cases.
Therefore, DSPEs are nothing but a combination of a functional polymer matrix and a conductive inorganic salt mixture. Dry solid PEs were also found to work as a separator between electrodes. This application requires properties like sufficient mechanical properties, elasticity and thermal resistance. Some of the lithium salts widely used are LiClO4, LiPF6, LiBF4, LiAsF6, and LiCF3SO3. The mobility of these ions is as follows: LiBF4 > LiClO4 > LiPF6 > LiAsF6 > LiCF3SO3.7 However, DSPEs are solvent-free, leakproof, low volatile, have good mechanical and electrochemical properties, high energy density, high process automation, flexibility, easy fabrication, and so on.19 Due to low ionic conductivities shown by solid PEs (significantly below 65 °C or at room temperature), many methods have been adopted for enhancing conductivity. Organic plasticizers/ionic liquids have been added to SPEs, leading to the development of GPEs.33
Plasticized/gel polymer electrolytes.
GPEs can be considered as a transition/intermediate state, which lies between SPEs and liquid electrolytes, where plasticizers are added to the polymers. Plasticizers are low molecular weight compounds that lower the Tg of the polymer by settling between the polymer chains and increasing the interchain distance of the polymer, through which the amorphous portion increases.19 The regularly used organic plasticizers are carbonates of ethylene and propylene, diethyl carbonate, and so on.64 This also contributes to ionic cluster dissociation, increase in charge carriers for ion transport, dielectric permittivity, and increased ionic conductivity.48 The Tg mitigation as a result of plasticizer addition results in local chain flexibility and coupling of chain flexibility and ionic mobility,65 as shown in Fig. 5.
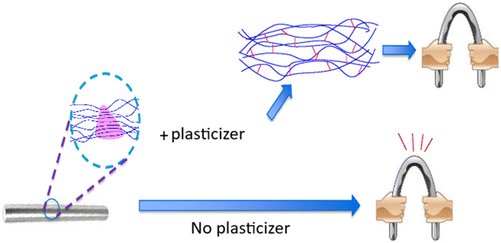 |
| Fig. 5 Schematic diagram illustrating the function of plasticizer in polymer matrix. Reused with permission from ref. 68. Copyright IJERT 2020 (open access CC-BY license 4.0). | |
As stated in the literature,33 when there is a larger amorphous region, ion charge carrier transportation will be good, enhancing ionic conductivity. Therefore, the solvents/plasticizers/ionic liquids included in the host SPE matrix will be in the liquid phase, leading to better ionic mobility. This kind of plasticized PE is also known as gel PE, which possesses the diffusive property of liquids as well as the cohesive property of solids.33,48 When more charge carriers are dissolved, the addition of plasticizers enhances the ionic mobility of GPEs. Liquid plasticizers like dioctyl adipate, dimethyl carbonate, diethyl carbonate, dibutyl phthalate, and propylene carbonate have been commonly incorporated into the polymer matrix to fabricate electrolytes with better properties.19 The “lubricity theory” emphasizes that plasticizers are employed as lubricants, which improve the motion of charge carriers, and the “free volume theory” says that the plasticizers influence the free volume of the polymer, leading to an increase in the amorphous region.66 A plasticizer's chemical structure, its miscibility and compatibility with polymers, its amount and molecular weight are the factors that determine the degree of plasticity in polymers.67 Some demerits are narrow electrochemical stability window (ESW), high vapour pressure, low flash point, narrow range of working voltage and reactivity of polar plasticizers with lithium electrodes.19
PEO is semicrystalline in nature, and adding a plasticizer reduces its crystallinity. The plasticizer used (in PEO) contributes to a decrease in surface roughness, leading to a smooth surface, which is evident in the increment of the amorphous region.69 Polyethylene glycol (PEG) is commonly used as a plasticizer in PEO-based SPE. With the increase in PEG plasticizer accompanied by a decrease in the molecular weight of the polymer, the ionic conductivity is enhanced.67 For example: PMMA-based Gel PEs exhibited conductivity around 10−4 S cm−1.70 PAN-based GPEs have shown high ionic conductivity of around 2 × 10−3 S cm−1, but the transference number remains the same as that of SPE.71 PAN/PVC blend-based microporous GPE had high ambient conductivity of about 5.01 × 10−3 S cm−1 when mixed with plasticizers, LiClO4, and it exhibited excellent electrochemical stability.72 Lithium superionic conductor (LISICON)-type SPEs were made, but they have limitations like a hygroscopic nature and low chemical stability. Kuo et al.73 developed LISICON-based GPEs using PVdF-HFP. These LISICON-based GPEs exhibited conductivity of about 10−4 S cm−1 and a high discharge capacity of about 134.6 mA h g−1. Gambe et al.74 prepared a UV-curable GPE, which was 3D printed, supporting the easy processing of thermally unstable materials. This 3D-printed GPE consisted of monomers, ionic gels, ionic liquids and silica nanoparticles for mechanical stability.
Ionic liquids have gained a considerable amount of attention in the case of GPEs, and are used to improve properties. These ionic liquids are simply room-temperature molten salts that consist of bulky cations (organic) and a highly delocalized anion (inorganic).7 Kim et al.75 proposed the room-temperature inclusion of ionic liquids into the dry SPEs to boost the electrolytic property. A sequence of ionic liquids was explored, which contained pyrrolidinium-based cations and TFSI-based anions, which were found to improve the conductivity of the electrolyte.75,76 Yin et al.77 synthesized a novel polymeric ionic liquid which exhibited good conductivity, electrochemical stability, and high thermal stability. However, the mechanical property was not up to the mark. In the case of blending GPEs, the purpose of one polymer is to absorb the electroactive species, and another one improves the mechanical integrity of the electrolyte.57,58 Therefore, blending is commonly done when a single polymer does not have a significant property needed for a particular application. PMMA is one of the ideal polymers used for Gel PE systems. The conduction and mechanical properties of lithium batteries were increased with the addition of PMMA/PVdF blend-based polymer as an electrolyte. This blend in a GPE system has been proved to enhance conduction properties.58
In summary, GPEs consist of a solid polymer matrix encompassing liquid electrolytes. Due to this design, they can act as both an electrolyte and a separator.35 To be used in commercial applications, GPEs should possess better ionic conductivity, good mechanical properties, the ability to hold liquid electrolytes and good electrochemical stability.7 The significant drawback of GPEs is poor mechanical strength. Block copolymer could be utilized to overcome this problem in GPEs78 in which one polymer exhibits good conductivity and the other helps to attain good mechanical stability. Other than mechanical properties, wide electrochemical resistance, high vapour pressure, low flash point, narrow working voltage and the reaction of plasticizers with electrodes also must be considered.19
Composite polymer electrolytes.
Researchers found that ion–ion association (either pairs or triplets) is the prime reason for the limited ionic conductivity of the PEs due to the weak dielectric constant.48,79 So, inorganic fillers with high dielectric constants are considered for solving this issue in the polymer matrix. The dielectric permittivity is controlled significantly by the type and quantity of the filler added. Ceramic materials are dispersed in PEs to enhance conductivity.48,80 The characteristics of particles to be considered are particle size, porosity, surface area, concentration and synergism between filler particles and polymer matrices, as they influence the electronic and ionic conductivity in the composite solid PE.19 By using these PEs, the properties of both polymer and ceramics can be attained. Whenever nano-sized composite fillers are utilized, the compounded PE is called a nanocomposite polymer electrolyte (NCPE). Dielectric constant and ionic conductivity are critical in determining the electrical properties of CSPE. Ceramic fillers enhance the ionic mobility of SPEs without compromising the mechanical properties.81
Inorganic fillers are of two types: inert and active, as shown in Fig. 6. In active fillers, nanoparticles are directly involved in ionic mobility by enhancing the free lithium-ion concentration, surface conduction of lithium-ions, or anion interaction. LiAl2O3, Li3N, and LiN2O3 are examples of active fillers. In the case of inert fillers, the dispersed nanoparticle fillers indirectly influence the ion transport mechanism, by increasing free volume and plasticization effects and by retarding polymer crystallization.2 Passive/inert fillers like Al2O3 and TiO2 nanoparticles indirectly enhance the ionic conductivity of SPEs. Ferroelectric fillers like BaTiO3 and PbTiO3 have also been used to improve transport properties by facilitating the dielectric constant of the system, reducing the crystallinity. Due to permanent dipoles, these fillers inhibit the interfacial stability between the electrode and electrolyte.81 These inorganic fillers also reduce crystallinity and glass transition temperature, resulting in superior interfacial stability, and this helps to stabilize the highly conductive amorphous phase.19 The ionic conductivity relies on the concentration and defect distribution. The diffusion of cations is based on vacancy mechanisms like Frenkel and Schottky point defects. When added, layered nano-clays provide a high interfacial contact area and dielectric properties, enhancing the solvation of cations.49 CSPEs can also be used as separators and possess good mechanical strength. The merits of CSPEs are good flexibility, high ionic conductivity, excellent thermal stability, good interfacial contact, etc.19,83
 |
| Fig. 6 Schematic representation of various types of inorganic fillers and their function in ion transport. Reused with permission from ref. 82. Copyright Carbon Energy 2021 (open access CC-BY license 4.0). | |
Micro/nanoparticles, Al2O3, SiO2, TiO2, ZrO2, magnetic sieves, and ferroelectric materials are used as inert ceramic fillers.2 The literature shows that nano Al2O3, SiO2 and TiO2 have been proved to augment the ionic conductivity of PEs by enhancing the interactions between the cations and the O2−/OH− present on the surface of the filler.37,84 With these nano-sized ceramics, nanocomposite polymer electrolytes (NCPE) are made. The interaction between nanofillers and polymer host matrix within a broad span of temperature (30 to 100 °C) boosts ionic conductivity and mechanical properties.47,85 Beyond the rise in conductivity and mechanical properties, inert filler addition leads to widening the ESW up to 5.5 V.47 Borgohain et al.85 found that hydrotalcite-doped nanocomposite PEs showed good mechanical strength. The concentration, structure, and size of nanofillers influence the conductivity, flexibility, and workability of the NCPEs. There are different types of nanofillers, such as nano-clays, metallic nanoparticles, nanofibers, and so on, as illustrated in Fig. 7. Nano-clays enhance the solubility of Li+ ions and provide a high interfacial contact area.2 High cation exchange, high aspect ratio, specific surface area, suitable charge within the interlayer and the length scale are the various essential significant properties of inorganic clays.81 Fumed silica (SiO2) nanofiller was incorporated into PEO-LiClO4, prepared as a nanocomposite PE, and exhibited broader electrochemical stability.19 PEO8-LiN(CF3SO2)2 with 5 wt% SiO2 PE system calcined at 900 °C was found to exhibit the highest conductivity of about 1.4 × 10−4 mho cm−1.87
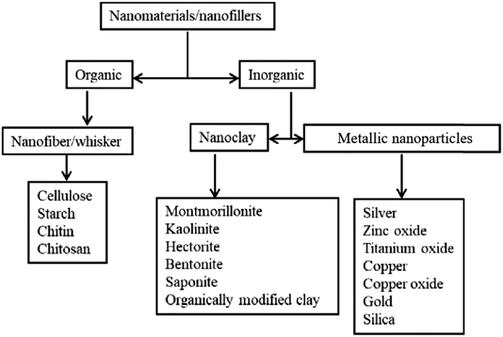 |
| Fig. 7 Classification of various nanomaterials which could be used as fillers in PEs. Reused with permission from ref. 86. Copyright Elsevier 2018 (license number: 5677260445174). | |
We can use the same blending technique here in CSPE too. A CSPE system was made by blending PEO, a branched polymer, a lithium salt, and a ceramic filler (BaTiO3). The conductivity of the blend system improved with respect to filler content and maximum conductivity at 10 wt% of the filler (BaTiO3).88 Therefore, blending of polymers contributed to the decrease of crystalline regions, leading to an increase in the amorphous portion and thus resulting in enhanced ionic conductivity. Li et al.89 worked on the in situ polymerization of TiO2 in PVdF-co-HFP. They reported that the interconnected pores on the sample surface made improving ionic conductivity and mobility difficult. The ionic conductivity of CSPE reached 10−6 S cm−1 at 30 °C when the polymer was compounded with Montmorillonite. Here, the MMT composite intercalated with the PEO and inhibited the crystallization, which enhanced the cationic mobility.90 Montmorillonite has distinct features like high cation exchange capacity, high aspect ratio, appropriate interlayer charge, large specific surface area and length scale.91 Studies have shown that polyvinyl alcohol doped with ammonium iodide possesses an ionic conductivity of 5.7 × 10−4 S cm−1.92 Sometimes, inorganic compounds/nanoparticles can be surface modified to enhance their dispersibility and interaction with the organic polymer molecules. Walkowiak et al.93 developed different surface-modified forms of SiO2, which led to a two-fold increase in the specific conductivity of a gel (about 10−2 S cm−1 at 20 °C) compared with the gel consisting of unmodified SiO2.
2.3. Methodologies for polymer electrolyte preparation
Polymer electrolytes are processed into films, membranes, fibres, and slurries and are used as electrolytes. These electrolytes are categorized into dry solid, gel/plasticized and composite PEs. These classifications have been discussed in detail in the previous section. Some of the methodologies utilized to prepare PEs are the solvent casting method, hot pressing, extraction–activation method, phase separation method, foaming, electrospinning, in situ polymerization and dip coating.
Solvent casting method.
Solvent casting is a method well known for its simplicity. A suitable polymer is dissolved in a low boiling solvent acetonitrile or tetra hydro furan, along with a non-aqueous lithium salt electrolyte. The mixture is cast into a substrate, and the solvent evaporates to give a film (Fig. 8).7,47,94,95 The solvent must have a boiling point of less than or equal to 65 °C and should be moisture-free. Usually, GPEs are mainly prepared by this method.7 The GPEs produced can have a variety of film thicknesses from 50–300 μm, and the solvent must be free of moisture because lithium is sensitive to moisture.7,81
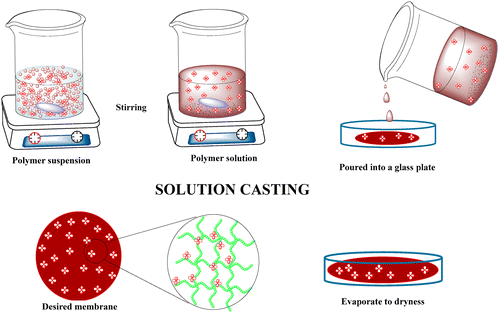 |
| Fig. 8 Preparation of polymer membranes through solvent casting process. Reprinted with permission from ref. 95. Copyright MDPI 2017 (open access under CC-BY 4.0). | |
The major limitations of the GPEs are stickiness, low mechanical strength, and the difficulty in evaporation of the solvent completely. Even though it ameliorates the ionic conductivity, it impacts the ESW of electrolytes; i.e., the residual amount of solvent will degrade under a certain voltage, and the voltage resistance will be disrupted.7,94 Because of the low mechanical strength, hardening is done either by physical or chemical crosslinking to enhance it.6 Another critical criterion is that the solubility parameter of the polymer and the salt must be equal so that they will be dissolved in the same solvent. In particular, the solvent can be eliminated by directly adding the salt species into the polymer matrix for solvent-free electrolytes. If the polymer is solid, it must be heated and melted, and salt must be added to obtain the required film. However, this will be time-consuming as the heating and cooling should be done repeatedly. Thus, we could use a low molecular weight polymer which exists in a liquid state to save time and energy.81
Hot-pressing.
The hot-pressing technique is widely considered for preparing polymer films as solid PEs are introduced. Initially, a suitable amount of polymer (usually pellets) along with the salts and fillers are added and mixed well in a mortar.96 The slurry is obtained by slowly heating the mixture near the polymer's melting point in a heating chamber.81,96 The mixture is then placed between steel blocks, as shown in Fig. 9, with a controlled pressure provided by a weighing cylinder. Finally, the required shape of the PE is obtained.81
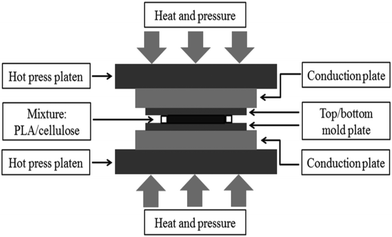 |
| Fig. 9 Schematic representation of hot-pressing technique to produce polymer films. Reprinted with permission from ref. 97. Copyright IOP SCIENCE 2016 (open access under CC-BY 3.0). | |
An alternative method is placing the mixture obtained between two heated mylar sheets/metal plates, where the temperature is enough to melt the polymer (i.e., the temperature should be below its decomposition temperature). Generally, the temperature range maintained inside a hot press is approximately 70 °C to 100 °C.94 For instance, the temperature applied to PEO is about 80 °C. The temperature and pressure to be applied depend on the type of polymer used. This technique leaves no solvent residue behind. The major advantage of this technique is that it can also be implemented for dense materials, requiring low cost and less time.81,98 Hot-pressed PEO electrolytes showed higher conductivity and more homogeneity when combined with freeze-drying than solvent-cast PEO electrolytes.94
Electrospinning process.
Electrospinning is one of the processes to produce fibrous polymer membranes of the required diameter (in nano meters) from polymer solution. The porosity range for the polymer membranes is 30–90%, and the pores contribute to the ion conduction of lithium ions inside the electrolyte.7 Polymer electrolyte membranes made by electrospinning will possess a huge porosity, higher surface-to-volume ratio, and suitable flexibility.47 Initially, a polymer solution is prepared and sprayed over the collector (either a plate or a cylinder). An electric field is created to induce charges within the solution droplets by the application of a suitable voltage between the nozzle and collector93 (see Fig. 10). When the charges within the solution reach a particular amount, the solution can overcome the surface tension forces. Then, it is ejected as a jet from the needle tip, forming a Taylor cone. The jet travels from the spinneret to a lower potential region, where a grounded collector as a sheet or drum is placed. The jet solidifies into nanofibers or nanowires of desired dimensions.99 The distance between the cylinder collector and the needle (nozzle) must be kept constant, and the feed rate is generally controlled by using an infusion pump.100
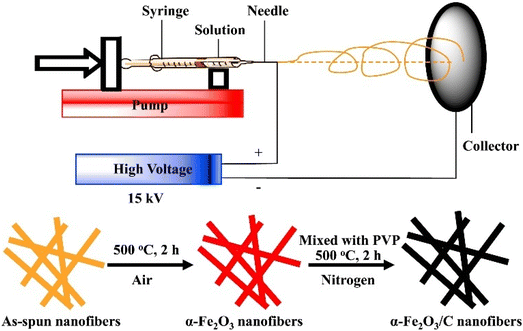 |
| Fig. 10 Schematic illustration of producing polymer-based nanofibers through the electrospinning process. Reprinted with permission from ref. 103. Copyright Elsevier 2016 (license number: 5673190990510). | |
Several parameters like applied voltage, solution concentration and polymer composition must be optimized to achieve good ionic conductivity, which is evident from electrospun poly(vinylidene fluoride) PVdF. However, PVdF–PMMA (poly vinylidene fluoride–poly methyl methacrylate) membranes were found to be solvent-dependent.7 The average fibre diameter is directly related to the polymer concentration, which depends on the viscosity of the solution.101 Obviously, the membrane/film fabricated through electrospinning has good mechanical properties because it produces nanofibers.94 Therefore, we can produce high-performance membranes through this technique. For example: PAN-PVdF (polyacrylonitrile-polyvinylidene fluoride) membranes yielded a maximum ionic conductivity of around 7.89 × 10−3 S cm−1. Huang et al.102 developed a cellulose poly(vinylidene fluoride-co-hexafluoro propylene) P(VdF-HFP) nanofiber in which the cellulose was the core and P(VdF-HFP) was the shell, prepared by co-axial electrospinning. The coaxial fibrous membrane showed high porosity (66%), high thermal stability (until 200 °C), high tensile strength (34.1 MPa), and high electrolyte compatibility (355% electrolyte uptake).7
Extraction activation method.
Bell Core7 introduced the extraction activation method for industrial production. Because of the high sensitivity of Li salts towards water, GPEs should be prepared in a moisture-free environment, which is not possible with techniques like solvent casting. Also, the mechanical properties of GPEs are generally poor and they need to be hardened by physical or chemical curing for better mechanical properties. To overcome these issues, the extraction–activation method72 with four stages for processing is recommended.
a. In the first stage, polymer, acetone, anode, cathode and plasticizer (dibutyl phthalate) are mixed and cast on substrates (Mold or Petri dish) to form a film.
b. The second stage involves the formation of cells laminating the obtained polymer films with Al and Cu collectors.
c. Then, the plasticizer DBP is extracted from the cells using a suitable solvent in the third step.
d. In the last stage, a liquid electrolyte is used to activate the battery after drying and packaging. This method is rarely used because it involves handling volatile solvents.7
To achieve high conductivity, polymer chains with low entanglements should take up more electrolytes.72 For example, synthesized PVdF gel PEs exhibited good electrochemical and mechanical stability.104 PAN–PVC-based microporous GPEs prepared in a 1
:
1 ratio by the extraction method exhibited ionic conductivity of about 5.01 × 10−3 S cm−1.72
In situ polymerization.
In situ polymerization is the process in which the polymerization reaction occurs directly by using a non-woven membrane as a scaffold.94 A network is formed under the required conditions to cure the electrolyte using a curable monomer (precursor), a liquid electrolyte, and an initiator, which are loaded into the lithium-ion battery (see Fig. 11). Simultaneously, the liquid electrolytes are evenly solidified in the gaps. The initiators commonly used are organic peroxides like benzoyl peroxide, peroxy-di-carbonate, t-amyl peroxy-pivalate and azo compounds like 2,2-azobisisobutyronitrile (AIBN).7 The precursor monomer must have a low viscosity, so the electrodes and separator will get wet easily, leading to good electrode–electrolyte contact and affinity. Therefore, the monomer for in situ polymerization should possess:
a. Low molecular weight.
b. Double bonds at their end.
c. Oxide groups for good compatibility with the electrolyte.
d. Good mechanical properties in gel PEs.
 |
| Fig. 11 Schematic representation of preparing PTHF electrolyte through in situ polymerization process. Reprinted with permission from ref. 107. Copyright Elsevier 2019 (license number: 5673191268709). | |
In situ polymerization promises good electrode/electrolyte interfacial contact and inhibition of dendrite formation. The excellent affinity of in situ polymerized PEs towards electrodes was first reported by Sun et al.105 This in situ polymerization is very much trusted for producing high-performance PEs, which could be utilized in flexible thin-film batteries and next-generation electronics.106
Acrylate groups and oligomeric polyethers were commonly used monomers for this technique.7 This method can easily synthesize PAN and PMMA-based gel PEs.106 However, this process has limitations like deterioration of cycle performance due to unreacted residual monomers and bubble formation because of the gas produced in the polymerization. These bubbles could affect the ESW, ionic conductivity and polarization of the PE.33
3 Property requirements of polymer electrolytes
3.1 Ionic conductivity
Ionic conductivity and resistivity are inverse operations, which result in a similar concept. Ionic conductivity measures the conduction/transport of ions through the electrolyte. One of the most critical parameters for electrolytes is ionic conductivity, which can be correlated to resistivity94 as follows:
where k is the ionic conductivity and ρ is the resistivity of the given material. Ionic conductivity is a function of temperature, and it is inversely proportional to the segmental relaxation time of polymers, which can be determined through quasi-elastic neutron scattering.71 As explained earlier, amorphous polymers are anticipated to show more ionic conductivity, because at room temperature, the crystalline and amorphous polymer chains are rigid and immobile. Crystalline polymers melt on heating, whereas amorphous polymers undergo transition above their Tg, and conductivity is gradually enhanced with an increase in temperature. Two models (Arrhenius model and VTF model), which are discussed in later sections, could help us to understand this conduction mechanism of PEs. Conductivity studies of PEs analysed by the Arrhenius model (Arrhenius plots) are mostly exponential.48,94 The PE must possess good ion conductivity and electron insulation capacity to improve ion transport. This conductivity informs about internal impedance and electrochemical behaviour. For a rapid charge/discharge, the ionic conductivity of a PE should be at least 10−4 S cm−1 at ambient temperature.7
Ionic conductivities of various types of electrolyte systems are represented in Fig. 12. The first ever reported PE was PEO-based SPE that exhibited low ionic conductivity below 60 °C and low transference number. To overcome this, researchers found other classes of electrolytes, like GPEs and CPEs, with low crystallinity and increased ionic conductivity. Most SPEs showed about 10−6 to 10−5 S cm−1, which is 3 to 4 times less in magnitude when compared with the conductivity of liquid electrolytes.32,94 GPEs and CPEs were prepared by employing (PEO) polymer matrix with added plasticizer/inorganic fillers, and the electrolytes were subjected to methods like blending, co-polymerization, crosslinking, and the addition of plasticizer and nanofiller etc., to improve the conductivity. PEO-PMMA blends exhibited poor mechanical properties due to more flexibility and amorphous portions in the polymer blend.19 Interestingly, PEO compounded with 0.05% graphene resulted in 10 times more conductivity than virgin PEO.109 High conductivity was achieved in a PAN-based microporous gel electrolyte of about 5.01 × 10−3 S cm−1 at room temperature, which was obtained by removing PVC from the PAN/PVC blend.47 The increased porosity of the polymers might contribute to good conductivity.90 These conductivity measurements for SPEs were done by using the two-probe method and impedance spectroscopy.38,48
 |
| Fig. 12 Ionic conductivities of various types of electrolyte systems. Reprinted from ref. 108 with permission from Royal Society of Chemistry. | |
Inorganic fillers dispersed in SPE help to enhance conductivity. Even though this approach can help to a certain extent, it also has some drawbacks like high cost and low processability.2 GPEs were made using polymer P(VdF-HFP) co-polymer in which 80% of the polymer was found to be amorphous, which seemed to possess an ionic conductivity of 0.5–1.5 mS cm−1. Furthermore, the ionic conductivity of GPEs was enhanced by gelling ionic liquids or ionogels along with the polymer matrix.94 Some of the techniques employed to ameliorate the conductivity of PEs were discussed in earlier sections. Some of the PE systems and their conductivity with their respective temperatures are listed below (Table 1).
Table 1 Conductivities of different polymer electrolyte systems with respect to the temperature
Electrolyte system (polymer/Li salt) |
Conductivity (S cm−1) |
Temperature (°C) |
Ref. |
P(EO)20/LiBF4 |
6.32 × 10−7 |
27 |
59
|
P(EO)20/LiClO4 |
2.78 × 10−7 |
27 |
59
|
PEO/5 wt%-LiPF6 |
1.20 × 10−6 |
25 |
88
|
PEO/11.1 wt%-LiAsF6 |
1.43 × 10−4 |
25 |
87
|
P(EO)20/LiCF3SO3 |
1.88 × 10−9 |
27 |
59
|
PEO/15 wt%-LiCF3SO3 |
1.00 × 10−6 |
RT |
85
|
P(EO)24/LiN(CF3SO2) |
3.84 × 10−4 |
50 |
110
|
P(PO)/10 mol%-LiClO4 |
>10−4 |
41 |
111
|
MEEP/10 wt%-LiCF3SO3 |
1.00 × 10−5 |
25 |
78
|
MEEP/25 wt%-LiCF3SO3 |
2.70 × 10−5 |
30 |
112
|
P(Si)32/LiN(CF3SO2)2 |
4.50 × 10−4 |
25 |
113
|
3.2 Electrochemical stability
The electrochemical stability window (ESW) contributes to selecting a PE. A PE must possess a broad ESW (i.e., the energy gap between conduction and valence bands should be greater than the energy difference between the potentials of two electrodes).112 The operating voltage of the battery plays a crucial role in determining the electrochemical resistance of the electrolyte used. ESW is also influenced by other factors like the molecular structure of the polymer, salt used and gelling liquid.94 The ESWs of the electrolytes are influenced by oxidation and reduction potentials, which are in turn controlled by the conduction band maximum and valence band minimum. When this requirement is not fulfilled, it can result in the development of a passivation layer at the electrode–electrolyte interface. Consequently, the lithium-ion transference number decreases, reducing the cycling life of the battery.112 The voltage resistance (ESW) of a polymer to be electrolyte should be in the range of 4–5 V versus Li+.7 Linear sweep voltammetry, cyclic voltammetry, and optical absorption spectra measurements are generally used to determine the polymer's electrochemical stability window. A schematic illustration of the ESW of Li+ solid electrolyte materials is shown in Fig. 13 and 14.
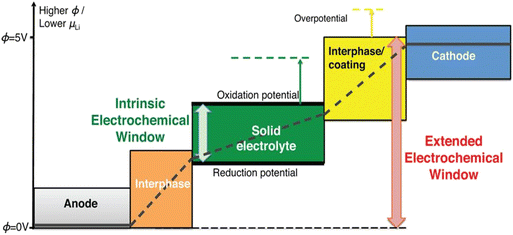 |
| Fig. 13 Electrochemical stability window of lithium solid electrolyte materials. Reprinted with permission from ref. 114. Copyright ACS 2015 (open access, author choice license). | |
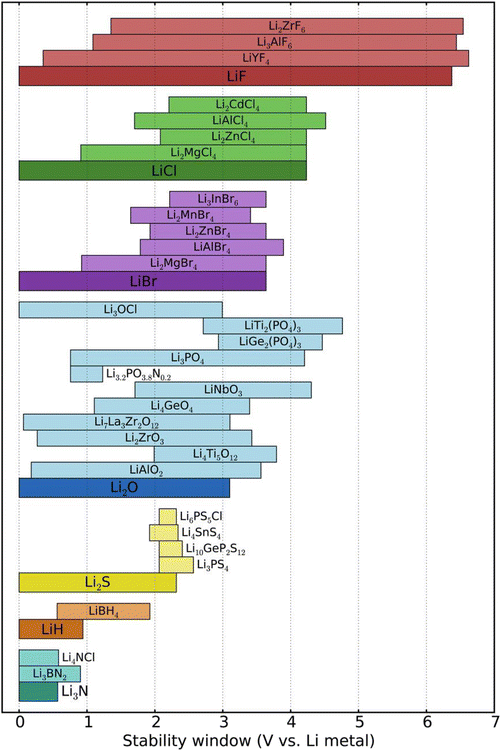 |
| Fig. 14 Electrochemical stability of various electrolytes complexed with anion. Reprinted with permission from ref. 115. Copyright ACS 2015 (open access, author choice license). | |
A method called density functional theory is widely used to investigate the ESW, as the other methods mentioned earlier are time-consuming. The ESW is tested/validated by determining the oxidation and degradation of the electrolyte system at a particular potential.112,116 The ESW of a PE relies on the salt used and its concentration.112 A passivating layer is formed due to the reaction between the electrode and electrolyte in Li-ion batteries. This passivating layer formed on the graphite (electrode) surface results in a limited battery life due to its limited stability.71 Microporous gel polymer membrane seems to exhibit a broad ESW. From the obtained voltammogram, the decomposition voltage was identified to be 5 V versus Li+.72 High electrochemical stability was obtained for composite PEs when Al2O3 was incorporated at 160 °C.113 For solid PEs, the anodic stability was improved by end-capping of PEO chains with methoxy chains to about 4.3 V. Reactivity and SEI formability influenced the electrochemical resistance of the PE in liquid plasticizer in GPEs.94
3.3 Thermal stability
Thermal stability is one of the essential properties for a PE to withstand the voltage generated when ionic transport takes place. So thermal analysis must be done, which is significant in terms of PE characterization. Thermogravimetric analysis and differential scanning calorimetry are helpful in the thermal analysis of polymers by which the thermal stability of the PEs can be found. Internal heat is produced in the batteries during battery operation which may result in short-circuiting and sometimes explosion. To get rid of these troubles, the thermal stability of the polymer plays a crucial role.46 Initially, PEO-based SPEs and Li-pol batteries were proposed, and they were proved to have good thermal stability up to 300 °C, as is evident from Fig. 15.117
 |
| Fig. 15 DSC curves of PEO-based SPE and Li-ion PB to determine the thermal stability. Reprinted with permission from ref. 117. Copyright Elsevier 2003 (license number: 5673210696723). | |
When PEO was incorporated with vermiculite sheets, it enhanced properties like the thermal stability and mechanical modulus of the electrolyte in good numbers.118 PAN-based microporous gel PEs exhibited thermal resistance up to 253 °C, as proved by DSC characterization.72 Polymer electrolytes must have enough thermal stability when used with battery components like electrodes, cell separators, current collectors, etc; this thermal stability assures us about battery safety in the case of electrical shorting, overcharge, flame, or any other issues.7
3.4 Mechanical property
Polymer electrolytes must exhibit desirable mechanical properties such that they can be stable and robust towards the electrode materials, enhancing the cell performances.87 Polymer electrolytes should not be too brittle but be able to relax the stress generated during cell manufacture, storage or assembly.7,119 The incorporation of liquid electrolytes or ionic liquids in polymer electrolytes (GPEs) exhibits poor mechanical strength,120 such that the electrolyte needs more strength to bear the stress between the electrodes.121 Unlike GPEs, dry solid polymer electrolytes possess superior mechanical strength at the cost of low ionic conductivity.19
CPEs are made using ceramic fillers or composite fillers, which boosts the conductivity without giving up the mechanical integrity of the polymers. Using 2D fillers like graphene oxides resulted in increased mechanical modulus along with the conductivity.118 Also, the addition of inorganic fillers like oxides of alumina, silica and titanium was found to improve the mechanical properties of the polymer electrolyte.122,123 Dynamic networks can be exploited to simultaneously achieve the conductivity and shear modulus of the polymer electrolytes without compromising ion transport.124 As explained in earlier sections, blending, copolymerization, cross-linking, addition of fillers, etc., can be utilized to enhance the mechanical strength of the polymer electrolytes.7
3.5 Dielectric constant and temperature effects
The dielectric constant is another essential electrical property of polymers. It can be represented as the ratio between the material's capacitance and capacitance of the empty cell, which correlates directly with the ionic conductivity. When the dielectric constant is high, the salt's lattice energy decreases and the charge carrier concentration will be increased, which provides higher ionic conductivity to the PE system.48 When the dielectric constant is high, the number of anions coordinated with Li+ ions is found to drop. Barteau et al.125 found that the rise in the dielectric constant of the host PE matrix contributed to conductivity enhancement. Ionic conductivity is the multiplication of concentration of charge carrier, electron charge and mobility of ions.49 Therefore, the dielectric constant relies on the charge carrier's concentration and density. In GPEs, the dielectric constant of the considered plasticizer must be greater than the polymer, which leads to the dissociation of the ionic clusters.126
The relative permittivity helps us to understand the ion transport of the plasticized and non-plasticized PVC-PMMA-LiCF3SO3 system which was reported by Ramesh et al.57 An increase in the relative permittivity of this plasticized PE system resulted in the enhanced density of the charge carriers. The plasticized PE systems (ethylene carbonate as plasticizer) have shown high dielectric constant due to increase in charge storage with respect to the ion dissociation.48 DC conductivity is strongly influenced by the relative permittivity of the polymers at different temperatures as reported in ref. 126. Transport of ions is always influenced by the relative permittivity and ion dissociation energy. In contrast, relative permittivity plays a significant role in the dc conductivity of the SPEs and NCPEs.48,126
At high temperatures, a rise in relative permittivity results in enhancement/improvement in the ion transport of the PE. As the temperature rises in polar polymers, it leads to an increment in dielectric constant due to the enhancement of dipole orientation, which is evident from the chitosan–AgTf SPE system shown in Fig. 16.127 High temperature contributes to improved ion transport and good vibration dynamics in PEs with a liquid-like mechanism, i.e., ionic mobility is defined in terms of the rate of segmental relaxation.78 Therefore, an increase in temperature increases dielectric properties, decreasing the hopping length of the ions and potential barrier needed for hopping of ions and increasing the flexibility of the PE.128 Most of the polymers exhibit low conductivity below their Tg because it ensures the amorphous behaviour of the polymer and facilitates the operating range of the SPEs.94
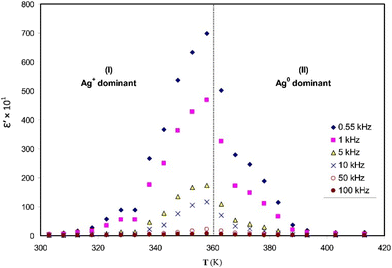 |
| Fig. 16 Temperature dependence of dielectric constant (ε′) at different frequencies for chitosan : AgTf (90 : 10) SPE. Reprinted with permission from ref. 127. Copyright Elsevier 2014 (license number: 5673210989263). | |
4 Ion transport models and mechanism of conduction in PEs
The ion conduction mechanism is very critical for PEs. Polar polymers dissolved with low lattice energy salts contribute to conducting PEs. From this, it is evident that the cations are the reason for the DC conductivity. It has been proposed that cations move by dissociation through the neighbouring coordinating site.129 Especially in polymers, when donor atoms like oxygen are added to the matrix, the polymer is suitable for electrolyte application since these O2 atoms help in the coordination of cations to promote salt dissociation.130 It is assumed that non-labile bonds are established between cations and polar polymers. However, this will not improve DC conductivity, whereas there should be labile bond formation between cations and polymer. As discussed earlier, conductivity principally occurs in the amorphous region.129,131 Many studies have reported that the conduction mechanism of PEs is still not clear. However, it could be observed that the cations are interconnected with neighbouring functional groups through which ion transport occurs.48 The most prevalent Li+ ion transport mechanism in polymer electrolytes that has been created thus far is segmental motion. Another mechanism rarely observed in SPEs is the Grotthuss mechanism/ion hopping.132 Two types of model are proposed through which the ion conduction mechanism in PEs could be studied.
4.1 Arrhenius model
The DC conductivity of the SPEs is the critical characteristic utilized in specific applications. For most of the polymers, the conductivity versus temperature plot will be linear, which comes under the Arrhenius model. The ion hopping mechanism has been used to analyse the polymeric electrolytes with regular crystal structures.134 Therefore, the Arrhenius model explains the correlation between the temperature and DC conductivity. The conductivity for the PEs following the ion hopping mechanism is defined through Arrhenius theory, which is expressed as:
σ(T) = σ0 exp[−Ea/KBT]; |
where σ(T) – conductivity, σ0 – conductivity pre-exponential factor, Ea – activation energy, K – Boltzmann constant, and T – temperature.135 This Arrhenius equation describes the discrete jumps of the ion motion which are independent/decoupled from the segmental motion of the polymer.136
The magnitude of conductivity is influenced by the amount of filler added, and most of the materials exhibited similar characteristics.17 The literature review revealed that the molecular motion of the polymer is not the reason for the movement of cations. When the temperature-reliant DC conductivity complies with the Arrhenius relation, the cations move through the neighbour vacant sites, contributing to high DC conductivity.137 The ion transport mechanism of PEO polymer through interchain and intrachain hopping is represented in Fig. 17. In the case of amorphous PEs above Tg, the temperature-dependent conductivity was found to be non-Arrhenius, which is said to be curved.138 Ravi et al.139 initially found this Arrhenius relationship between temperature and DC conductivity for poly(vinylpyrrolidone)-KClO4 complexed SPE, which is evident from Fig. 18. The temperature increment leads to enhanced conductivity as a consequence of the development of free volume around polymeric chains, facilitating ion transport. Polymer chain motions like breathing, relaxation or segmental mobility are observed along with the ion hopping mechanism.130 Most of the polymers used as PEs are semicrystalline in nature. Below the melting point, there will be no segmental motion/internal Brownian motion (IBM) of the polymeric chains, and hence, this IBM of the chains could be observed above the melting point (which aids in high DC conductivity).48 The plot DC conductivity versus 1000/temperature shown in Fig. 18 is made using the Arrhenius equation. The following are the observations made for the SPE-based system.
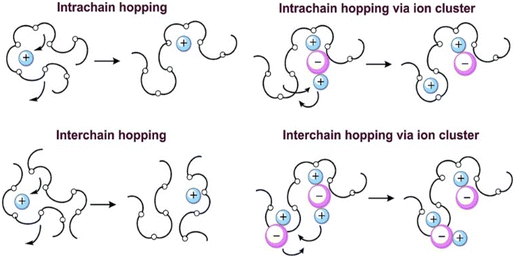 |
| Fig. 17 Cartoon illustrating the ion transport in PEO SPE. Reprinted from ref. 133 with permission from the Royal Society of Chemistry. | |
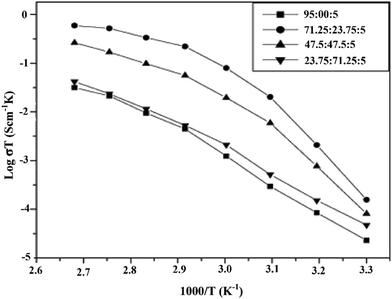 |
| Fig. 18 Temperature dependence of ionic conductivity for PVAc : PVdF : LiClO4 polymer electrolytes containing various blend ratios. Reprinted with permission from ref. 140. Copyright Elsevier 2006 (license number: 5677261076435). | |
Two regions are observed, where region 1 is below Tm and region 2 is above Tm of the polymer (see Fig. 19). In region 1 (below Tm), the DC conductivity increases gradually until 70 °C.141 In region 2 (above Tm), the DC conductivity increased instantaneously compared with region 1. This is because, at high temperatures, segmental motion is observed where free volume will be comparatively higher. This will help the ions to move from one place to another. In region 2 (amorphous region), the bond rotation creates segmental mobility at elevated temperatures.142 Consequently, this segmental mobility contributes to the conductivity of the PE and ion hopping motion, which is interchain or intrachain. Researchers reported that the transport of ions is due to the segmental mobility of the polymer and the hopping/transitional mobility of the ion.48 Amorphous regions begin to swell at high temperatures where the polymer segments exhibit IBM because of the bond rotation. Consequently, this helps in the inter and intra-chain ion hopping mechanism of ion motion, which consequently increases conductivity.142
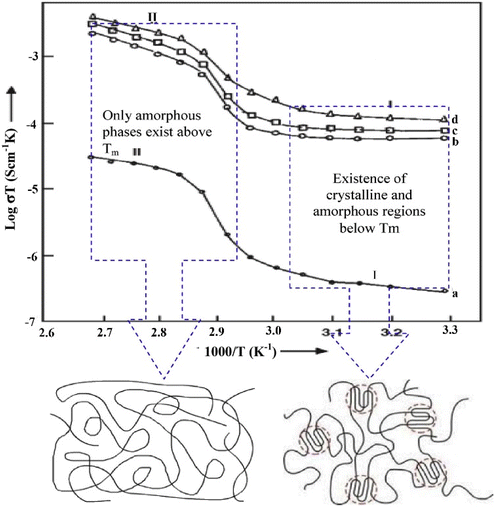 |
| Fig. 19 Temperature dependence of dc conductivity of (a) pure PEO, (b) (PEO + NaClO3) (90 : 10), (c) (PEO + NaClO3) (80 : 20), and (d) (PEO + NaClO3) (70 : 30). Reprinted with permission from ref. 141. Copyright Elsevier 2006 (license number: 5677791086137). | |
The decoupling of segmental relaxation and conductivity has been observed for polymer electrolytes following Arrhenius behaviour. With the help of rheology, a direct comparison between segmental relaxation times and conductivity relaxation times has shown a notable difference.143 However, only a very few works combine conductivity with the segmental relaxation of polymer electrolytes, which prevents us from understanding the decoupling mechanism. For molten salts and ionic liquids, an easier approach has been proposed for understanding the decoupling.144–146 Many polymers exhibit strong decoupling of conductivity and segmental mobility, which shows the solid-like mechanism of ion transport in certain cases (decoupling).78 Therefore, polymer electrolytes seem to follow a decoupling solid-like mechanism below the Tg. A solid-like mechanism is sometimes found above the Tg when the rate of ion rearrangement is greater than the segmental relaxation.
4.2 Vogel–Tammann–Fulcher model
The Vogel–Tammann–Fulcher model is abbreviated as the VTF model, and is used to explain the ion transport mechanism in polymers. The VTF model relates the ionic conductivity and segmental relaxation in the host PEs.48 Here, the curvy behaviour of Arrhenius plots has been observed for the relationship between conductivity and segmental mobility. Therefore, the correlation between ionic mobility and the temperature can be represented as:
σ = σ0T−1/2 exp[−B/kB(T − T0)]; |
where σ0 is the pre-exponential factor related to the number of charge carriers, B is the pseudo activation number and T0 = Tg − 50 K.7,147 Therefore, the segmental mobility and the ion concentration affects the ionic conductivity of the amorphous polymers following the VTF model.148 The free volume model was considered by the researchers to comprehend the relationship between ion transport and IBM, leading to a massive increase in conductivity at high temperatures. From free volume theory, we infer that the polymers can expand and produce free volume at high temperatures. This free volume is a major concern for ion transport here.48 A non-linear curve is obtained for the plots of the VTF conduction mechanism of SPEs and is generally observed above the Tg.7,130 Also, this VTF behaviour was found above the Tg of the solid polymer electrolytes, ionic liquids, and GPEs.149
The diffusion coefficient of the ions was studied, and the VTF model predicted the temperature variation at various concentrations of PMEO-based PEs. This temperature dependence can be explained using configurational entropy or free volume theories.129 For ion-conducting solid electrolytes, the temperature dependency of conductivity was explained well by the VTF model.150 Beyond the polymer segmental motion, the Arrhenius conductivity exhibits a linear curve (straight line) at high temperatures. At high temperatures, conductivity enhancement leads to the vibrational dynamics of the polymer chains and backbone. Through these vibrations, coordination sites come close together, resulting in ion hopping or translational motion that leads to ion transport. So, the graph will be linear for DC conductivity versus the 1000/T plot for the Arrhenius model, like ionic crystals.22 We could observe dual ion conductivity (both cations and anions), which would affect the ion transference number of the system, influencing the performance of the SPEs.151 Uncoordinated cation mobility is anticipated when the transference number value is low. This difference in concentration inside the electrolyte and between the electrodes can pose serious problems, i.e., ionic resistance may develop/enhance due to salt precipitation/depletion at the electrode surface.152,153 As a result, this difference in concentration hampers the charging/discharging process and could also lead to dendritic growth, which reduces the performance of the battery.154 Moreover, studies suggest that solvent molecule selection is pivotal because the solvent is helpful in solvating the Li+ ions, and these ions migrate within the electrolyte. Therefore, the solvent molecules affect the ion transport of the system.155,156
In summary, the coupling and decoupling mechanisms between the ionic and segmental motions contribute to the linear and curved behaviour of DC conductivity.48 However, the plots between conductivity and 1000/T show a non-linear connection, which supports the conductivity mechanism through ion hopping motion coupled with relaxation of chains for PEs modelled using the VTF model, which is evident from Fig. 18.7,141 Polythiophene composites were studied/analysed with the help of the VTF model, and their temperature-dependent conductivity was identified. Here, the composite followed the VTF model until a critical temperature, after which a crossover from VTF to Arrhenius behaviour was found.150 The works of Petrowsky and Frech enabled us to understand that DC conductivity is influenced by temperature. Nevertheless, it is also a function of relative permittivity.16 Summing up all, DC conductivity seems to be a function of both temperature and dielectric constant. Conductivity also relies on the dielectric constant and energy of ion dissociation of the PE. Therefore, these ionic and molecular interactions in ion-conducting PEs could be studied using dielectric relaxation studies.48
5 Characterization of polymer electrolytes
Characterization is a necessary element to identify the physical and chemical properties of polymers, which helps in the testing or validation essential for a material. The characterization techniques can acquire information like conductivity, crystallinity, electrochemical resistance, surface topology, composition, thermal resistance, and other properties of the sample. This section discusses some of the principal electrochemical characterization techniques like electrical impedance spectroscopy, transference number measurement and voltammetry, which are critical for PEs.
5.1 Electrical impedance spectroscopy
Electrical impedance spectroscopy is a transfer function measurement type generally used to analyse linear time-invariant systems.157 This method is also known as dielectric spectroscopy or AC impedance spectroscopy. The electrical properties of the polymer mass or its interfaces for various frequencies and temperatures can be identified using this method. This method is also used in studying dielectric relaxation and ion conduction mechanisms in PEs.48 Thus, complex impedance spectroscopy could be used to study the AC ionic conductivity of the PEs.135 The impedance graph can be achieved by analysing the sample in the frequency range between 100 Hz and 1 MHz, and for resins, suitable lower frequencies are employed for the experiment.158 Hence, the dielectric properties of the PEs like dielectric loss, dielectric constant, ac conductivity, modulus and tangent loss, can be determined from the impedance spectra.128
Electrical impedance spectroscopy theory.
Electrical displacements in a material are attributed to two significant physical phenomena:
1. When electrical charges are diffused for long distances, diffusion takes place, and DC conductivity occurs.
2. When electrical charges are restricted to a localized matter, polarization will occur.159
Therefore, AC conductivity analysis is done using complex impedance spectroscopy to eliminate the interfacial polarization at the electrode/electrolyte interface. Complex impedance spectroscopy (CIS) is used to predict the ac conductivity of PEs by means of applying an electric field, as said earlier.160 This electric field will cause polarization in polymers. The four types of polarization for polymeric materials are electronic, atomic, dipolar, and migrating charge polarization.161 In raw materials, ions frequently emerge as impurities. The dipoles begin to form when there is unequal electronegativity of atoms attached to the polymer backbone.48 Therefore, the dielectric responses of the polymers are due to the electronic and orientational polarization, which can be analysed over the frequency range of less than 109 Hz.161 The inversion of the electric field leads to the reorientation of dipoles with respect to the direction of the field, and migration of ions from one electrode to another is seen. Also, the reactive sites of huge sizes would be difficult to reorient along the direction of the field.48 EIS can be done with a frequency response analyser and a potentiostat governed by a computer with a suitable application. The response of the system results in an electrochemical impedance shown as,
Z(ω) = Zr + jZj = V(ω)/I(ω); |
where
Z is the impedance,
ω is the angular frequency, and
V and
I are phasors, which are complex time-invariant numbers.
157 For impedance data to be considered valid, three basic requirements must be satisfied before doing EIS techniques: 1. linearity, 2. causality, 3. stability.
Linearity: the system must behave linearly with respect to the measuring conditions; i.e., the input and the output signals should pertain to the same frequency. Most of the systems exhibit non-linear relationships, so we apply a small AC signal to make it linear. This linearity condition for a chosen AC signal can be pragmatically tested by changing the magnitude of the applied AC signal. If the impedance remains the same, then the linearity condition is satisfied, or vice versa. Theoretically speaking, the Kronig–Kramers transformation relation is used to check this linearity.162
Causality: causality suggests an exclusive cause-and-effect connection between the input signal and the output of the system. Therefore, there shouldn't be any external interference to the system, which means that the applied perturbation must be the only factor influencing the output of the system.163
Stability: the system under study must be stable over a wide range of frequencies and measurement conditions. This means that the system must be capable of returning to its initial state if the perturbation stops.164
For mixed and amorphous systems, CIS evaluates the conductivity and permittivity which are dependent on frequency at various temperatures.48 The complex impedance plots are very useful in obtaining the ionic conductivity of the polymer electrolyte.135 Using complex impedance spectroscopy, the real and imaginary parts of impedance for broad values of frequencies are obtained through the Nyquist plot. This method depends on the response of the system to AC sinusoidal input waves where impedance is calculated. Complex impedance spectroscopy can also calculate frequency at relaxation, electrode separation, and bulk effects. It is also used to measure the DC conductivity, AC conductivity, dielectric loss, dielectric constant and energy of activation required for the charge carriers.48 Here the polymer electrolyte samples are sandwiched between two blocking electrodes made of stainless steel or aluminium.165
Experiment and analysis.
A sinusoidal voltage of 5 to 20 mV with a wide span of frequencies was applied to the sample PE, and the current measured.81 This EIS was carried out using VMP potentiostat and EC lab software. As mentioned earlier, the two-electrode setup is where the electrode/electrolyte/electrode is built as a coin cell using stainless steel or aluminium.158 As mentioned earlier, the ratio of voltage versus current gives the complex impedance. We shall get the impedance plot from this measurement, which is Z′′(omega) vs. Z′(omega). By fitting the impedance plot using the EQ program, we can get the bulk resistance of the PE sample.163,166 By using the relation between resistance and resistivity, the resistivity of the sample is obtained, from which conductivity is obtained; i.e., we know that conductivity is the inverse value of the resistivity.164 From Fig. 21, we can see the impedance plot of PVA
:
AgNt (75
:
25) at 303 K. Here, the solid polymer electrolyte seems to possess bulk resistance of about 160
578 ohms, which is the value taken from the end of the semicircle in the IS plot.167 From this value, we can obtain the resistivity and conductivity of the PE through the respective relations and formulas as mentioned earlier.
Chang-Yu Hsu et al.168 reported that the maximum proton conductivity for this PE system was 10−1 S cm−1 above 60 °C, which was greater than the conductivity of Nafion-117, evident from Fig. 20. Subramania et al.72 reported on PAN/PVC blended microporous gel electrolytes, which were found to exhibit ionic conductivity of about 5.01 × 10−3 S cm−1 at RT mixed at the ratio of 50
:
50 (w/w). Ionic conductivity of such value was achieved owing to the elevated electrolyte uptake of this microporous gel electrolyte. PEG-DA samples have been produced in different salt concentrations, and the highest conductivity was found to be obtained with 20 wt% of LiClO4.169 The Nyquist plots yielded a semicircle or linear relation for gel-type electrolytes.158 Generally, the impedance plots of electrolytes were found to be complicated, where a deviated semicircle or slanted/curved spikes were found, as shown in Fig. 21 for PVA-AgNt. These distortions in the semicircle can be influenced by the behaviour at the interface of electrode and electrolyte and the homogeneous distribution of properties.164
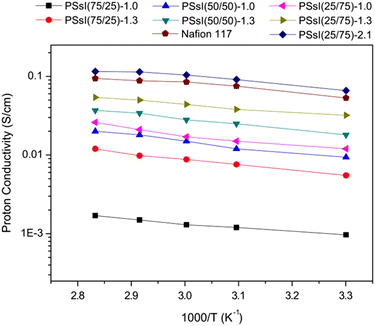 |
| Fig. 20 Arrhenius plot of proton conductivity of Nafion-117 and PSsI membranes at 100% RH. Reprinted with permission from ref. 168. Copyright Elsevier 2015 (license number: 5677791420578). | |
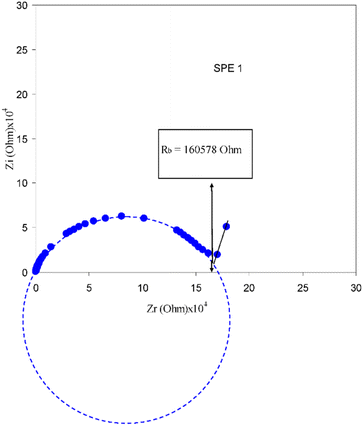 |
| Fig. 21 Impedance plots for PVA : AgNt (75 : 25) at 303 K. Reprinted with permission from ref. 167. Copyright MDPI 2017 (open access CC-BY license 4). | |
5.2 Transference number measurements (TNM)
Theory.
One of the crucial parameters for a PE is transference number measurement, which represents the proportion of electric current transported by the cation (e.g., lithium or sodium).94 Having a high transference number (TN) for a polymer electrolyte is highly recommended; i.e., the anion mobility should be low while the battery is performing because it may indicate wasted energy and lead to decomposition, causing detrimental effects for the cell.170 A TNM of lithium-ions that is close to unity (theoretical value) is desirable. This high TN value lowers the transport overpotential during the charge cycle process, contributing to enhanced specific energy.7 A transference number equivalent to unity was observed for a subsection of GPEs called single-ion conducting polymers, which shrinks the adverse effects of anionic polarization.78
The transference number was measured/determined for pure polymers above 110 °C temperature to allow the diffusion of molecules to pass through at a low frequency. For filled polymers, the temperature limit will be extended.17 Generally, TNM can be determined by using electrical polarization, nuclear magnetic resonance and electromotive force method.171–173 However, the electrical polarization method is the most popular approach because it is straightforward and simple to use.174 TNM generally depends on both blocking and non-blocking electrodes. TNM for a solid PE is generally determined by Wagner's DC polarization method and potentiometric polarization of Bruce and Vincent method.175
Experiment and analysis.
Ion transference number.
This technique predicts the function/input of ions and electrons to the total charge transport of the PEs.175 In total charge transport, Wagner's polarization is adopted to isolate various contributions of electrons and ions. The PE sample is placed between two blocking electrodes (using stainless steel). The experiment is carried out by applying a small DC voltage and obtaining the response of the current with respect to time. Because of the polarization of mobile ions at working/blocking electrodes, the current diminishes as time increases.176 The ion transference number is given by,
where Ie is the total initial current because of the ions and electrons, and It is the constant residual current because of electrons.81
Cation transference number.
This method is well known for its simple and fast procedure. Here, symmetrical metal/polymer/metal disks are polarized by a small voltage of about 10 mV. Then, the initial and equilibrium state current values are obtained.94 The initial current value decreases until a steady-state value is attained when a small constant voltage is applied to an electrolyte between non-blocking lithium electrodes. The anion current will disappear in the steady state, and the cations will be responsible for the whole current if there is no redox interaction with the anions.177 In this instance, it is simple to calculate the cation transference number by dividing the cationic steady-state current by the initial current immediately following the polarizing activation voltage. An extra contact resistance is typically imposed by passivating layers at the electrodes. Deducing the matching extra voltage drop from the applied potential difference is necessary. Therefore, the correction needs to account for this change since the resistances of the lithium electrode surfaces change with time.173 Before and after the process of potentiometric polarization, the contact resistance of electrodes is predicted by using impedance measurements. A DC voltage is applied for polarizing the cell, and the cation transport number is given as,
tcation = Is(V − I0R0)/I0(V − IsRs); |
where V represents the applied DC voltage, I0 is the current before polarization, Is represents the current after polarization, R0 is the initial resistance, and Rs is the steady-state resistance of passivating layers.81,83 The abovementioned equation is referred to as the Bruce–Vincent method for transference number measurement.
PEO-based PE was investigated using Macdonald's analysis, which shows that the transference number improved by adding Al2O3 at a volume percentage of about 10% and showed the temperature independence of the transference number.17 Baskaran et al. investigated the transport numbers of PVAc-PVdF-LiClO4 using Wagner's polarization method. Measurement was carried out using aluminium electrodes and applying 1.0 V DC bias voltage at 333 K as shown in Fig. 22. The tion was found to be 0.96–0.98, which suggested that the ion transport or ionic conductivity was mostly because of the Li+ ions.140 For NCPEs, Wagner's polarization test was done. The transference number was obtained as 0.97, revealing that the characteristic of conductivity was ionic,91 as the anion mobility is inversely proportional to the lithium transference number. So to enhance the transference number, we can (a) anchor the anions to the polymer architecture and (b) introduce anion receptors into the polymer-salt complex.7
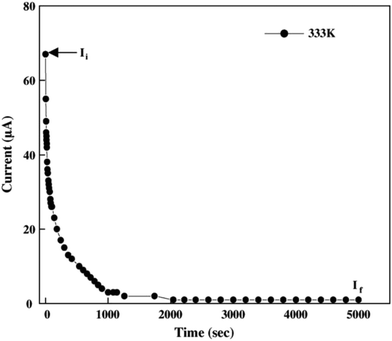 |
| Fig. 22 Current vs. time plot of 71.25PVAc : 23.75PVdF : 5LiClO4 polymer electrolyte at 333 K. Reused with permission from ref. 140. | |
5.3 Voltammetry
Voltammetric studies are commonly used to calculate the ESW of the PEs in which lithium is employed as a counter electrode (Li-ion batteries), whereas for working electrodes, inert materials like carbon and stainless steel can be utilised. Through the voltammetric peaks, the potentials at which the PE sample undergoes redox reactions can be obtained.178
Theory.
To learn about the theoretical background of this technique, we should look over the Nernst or Butler–Volmer equation.179 According to the equation, the applied potential and reaction rate controls the concentration of the redox species at the electrode species. Consider a reversible electrochemical reaction:
Here, the applied potential (E) is responsible for pushing the concentration of O and R (
and
) at the surface of the electrode to a ratio which can be defined by the Nernst equation:
where
E° is the standard reduction potential of the redox couple,
R is the gas constant,
T is the absolute temperature (K),
F is the Faraday constant, and
n is the number of electrons. From this equation, we can see that the potential applied is directly proportional to the concentration ratio

).
180 The Butler–Volmer equation relates concentration, current and potential, which is given as:
where
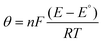
,
A is the area of the electrode,
k° is the heterogeneous rate constant, and
α is the transfer coefficient. The electrode surface and the flux of the material directly affect the current flow. Fick's law defines the mass movement, and difference in concentration due to the destruction of O and R and the formation of new O and R. Fick's law is given by:
where
DO is the diffusion coefficient of O, and
x is the distance from the surface of the electrode. Therefore, the faradaic current in the cell and the rate of the reaction are controlled by the flux of O and R.
181
Experiment and analysis.
Voltammetric measurement is done by using a Li|Ni or Li|stainless steel cell.83 Linear sweep voltammetry and cyclic voltammetry are the two types of techniques to determine the voltage limit of the PE, where we apply the anodic voltage to the cell holding the PE film/membrane.113 The difference is in cycling the potential range. In linear sweep voltammetry, we sweep the potential from low to high value and plot the voltammogram, whereas in the cyclic one, we sweep the potential from low to high value and vice versa. The anodic potential limit of the PE sample can be obtained from the inflection point of the voltammogram, and the cathodic potential limit is also determined in the same way.182 Here, the onset of the current shows that the electrolyte begins to decompose and loses its stability.113 Cyclic voltammetry (CV) offers two key benefits: (i) results can be easily interpreted and (ii) vast potential ranges can be quickly analysed. CV also provides us with information on the formal reduction potential, reversibility of the reaction, energy levels of the semiconducting polymers, and electron transfer kinetics.183
Therefore, the decomposition voltage is determined from the voltammogram where the curve hikes to a positive value from zero, where the degradation of electrolyte starts, which is evident in Fig. 23, where the PVdF and PVdF-MMT samples were subjected for characterization. From Fig. 23, we can find the voltage of PVdF is 4.3 V. We can also observe that the increase in MMT filler clay loading enhances the degradation voltage value.91 Park et al. reported the LSV measurements of CSPE at 16 °C, as shown in Fig. 24. Higher electrochemical stability was observed for the CPE made of acidic activated Al2O3 or neutral activated Al2O3 than for CPE made of activated basic Al2O3. These differences are due to the intermolecular interactions (H-bonding) leading to high electrochemical stabilities.113 However, the working potential of the electrolytes goes beyond the voltage limit because of the development of SEI, which is pivotal regarding lithium-ion batteries.83
 |
| Fig. 23 LSV graph of gel PVdF and PVdF-MMT NCPE with different concentrations of clay. Reprinted with permission from ref. 91. Copyright Elsevier 2011 (license number: 5677800468827). | |
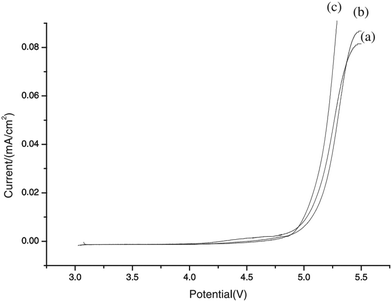 |
| Fig. 24 LSV of the PEO-based CPE at 60 °C. Salt content is [LiClO4]/[EO] = 0.1 and inorganic filler is 10 wt%. (a) Al2O3 activated acid; (b) Al2O3 activated neutral; (c) Al2O3 activated basic. Reprinted with permission from ref. 113. Copyright Elsevier 2003 (license number: 5677800798772). | |
6 Applications of polymer electrolytes in various fields
The crucial utilization of PEs can be seen in the field of battery technology. Polymer electrolytes are the latest development in lithium batteries.33 Solid PEs revolutionized the field of batteries due to their safety (no leakage of electrolyte), light weight, high energy density, good compatibility between electrode and electrolytes, and good thermal and mechanical properties compared with liquid electrolytes.19 The ionic conductivity of solid PEs was poor, but the addition of filler and plasticizer was shown to improve the properties. Also, PEs are pivotal in various electronic applications like dye-sensitized cells, electrical double-layer capacitors, fuel cells, lithium batteries, etc., as shown in Fig. 25. Previous to this section, polymer electrolytes in terms of Li-ion batteries were discussed. Therefore, some of the applications other than batteries are comprehensively explained in this section.
 |
| Fig. 25 Applications of polymer electrolytes in various fields. Reprinted with permission from ref. 46. Copyright Elsevier 2019 (license number: 5683790706860). | |
6.1 Dye-sensitized solar cells
Solar energy is one of the renewable energies that has become significant and exploited in some applications due to the limitations of fossil fuels. Some solar energy applications are solar thermal collectors, photovoltaics, and dye-sensitized solar cells (DSSCs).19 The prime principle of the DSSC is converting light (natural or artificial) energy into electrical energy. Polymer electrolytes are used in DSSCs to improve their performance and properties. Liquid electrolytes in the DSSC cause significant problems like leakage, corrosion, evaporation and poor stability.184 Solid PEs help to overcome these drawbacks by replacing the liquid electrolytes.184,185 To increase the conductivity, we can add some nanofiller or plasticizers, or gel PEs can be synthesized with better conductivity than SPEs, or ionic liquids can be polymerized along with the polymer matrix to produce ionic liquid polyelectrolytes.184 A photoelectrode, a counter electrode and an electrolyte make up the DSSC. The photoelectrode possesses a nanocrystalline mesoporous semiconductor (TiO2) with a wide band gap, generally dipped/soaked in a sensitizing dye to form a monolayer. The counter electrode usually comprises platinum or carbon on a conducting substrate. The electrolyte involves a redox system that connects and transposes charge between two electrodes.185,186 The dyes play a crucial role in converting light into electrical energy.19 Solid-state electrolytes (SSEs) have recently become very significant in this DSSC application. The SSE is constructed by a p-type hole conveying semiconductor and yet another solid-state redox couple. This enhances the electrochemical stability and non-corrosive properties.186
There are two types of DSSC based on the type of polymer electrolyte employed: solid-state DSSCs, which utilize SPEs, and quasi-solid DSSCs, which make use of GPEs.187 The process of casting the PE solution directly over a dye-adsorbed TiO2 photoelectrode typically helps create SPEs that lead to solid-state DSSCs. PEO-based polymer electrolytes became standard SPE systems of analysis after the work of Wright.36 Low molecular weight poly(ethylene oxide dimethyl ether) (PEODME. Mw = 500)/MPII/I2/fume SiO2 SPE was used by Kim et al.188 to replace potassium iodide with 1-methyl-3-propylimidazolium iodide, which increased the solar efficiency by up to 4.5%. An overall conversion efficiency of about 0.01% was achieved for high molecular weight PEO by incorporating the polypropylene glycol oligomer.189 Consequently, a lot of work was done with GPEs to make quasi-solid DSSCs. Researchers from Malaysia and Sweden have reported on GPE based on polyacrylonitrile which exhibits enhanced ion transport properties in DSSCs.190 Çetin et al. proposed a GPE of poly(2-acrylamide-2-methylpropane sulfonic acid/itaconic acid/N,N′-methylene bis acrylamide) hydrogel and its variants doped with halogens (F, Cl, Br) and aniline.191 Lan et al.192 developed a PAN-based GPE that showed a maximum conductivity of 2.37 × 10−3 S cm−1 at RT and an overall energy efficiency of 2.46% for light to electricity. PMMA-based GPE has been synthesized and used in a quasi-solid state DSSC, which offered light to electricity conversion of 4.78%, good long-term stability and desirable ionic conductivity of about 6.89 × 10−3 S cm−1.193 Also, work has been done to prepare GPEs through the swelling technique, and a new class of PEs based on ionic liquids have been employed in DSSCs.187 These PEs exhibit a wide range of properties and various advantages; however, they are not considered in this review.
6.2 Fuel cells
The fuel cell is considered as a replacement for non-renewable energy sources, leading to a sustainable energy source. It is a type of energy-storing device that converts chemical energy to electrical energy. Like batteries and DSSCs, fuel cells are also made of an anode, an electrolyte and a cathode.19 A hydrogen fuel cell necessarily takes in hydrogen and oxygen. When these elements are continuously supplied to the fuel cell, the waste product is removed, and electricity is generated efficiently without any combustion.185 The electrolytes play a vital role in carrying/transporting the electrically charged ions from one end of the electrode to the other end, and the other way around. Membranes like Nafion and Aquivion can be used as electrolytes. There are two types of polymer electrolyte membrane (PEMFC) for fuel cell technology, namely: (i) low temperature (LT-PEMFC) and (ii) high temperature (HT-PEMFC). LT-PEMFC operates at 60–80 °C, whereas HT-PEMFC operates at 120–200 °C.194 In many of the fuel cells, the operating temperature is stringent to about 120 °C. For instance, Nafion can degrade at 80 °C (LT-PEMFC), allowing methanol and water crossover. These drawbacks are rectified by a new class of material, namely hydrocarbon membranes, in which the operating temperature is extended up to 150 °C (HT-PEMFC). Relatively high membrane cost, catalyst poisoning, expensive catalysts, and water management problems were observed from the conventional perfluoro sulfonic acid-based LT-PEMFC.195 Therefore, operating PEMFCs at high temperatures gives us various advantages. By operating PEMFC at high temperatures, we can observe quicker reaction kinetics, better waste heat generation, simpler thermal management, and high tolerance of Pt catalysts to fuel impurities.194,196
Initially, LT-PEMFCs utilized perfluoro sulfonic acid (PFSA) membranes where the main chains are hydrophobic, and the end chain sulfonic groups are hydrophilic. Therefore, good hydration properties must be achieved.197,198 Due to the limitations of LT-PEMFC and the advantages offered by HT-PEMFC (mentioned above), researchers started to work with HT-PEMFCs. So, PFSA membranes were modified to operate at high temperatures, but water was still employed for proton conductivity. Inorganic fillers were also added to these modified PFSA membranes to improve their performance and properties.199–201 Sulphonated hydrocarbon polymers were employed as HT-PEMFC because they offered several advantages in terms of properties like increased water uptake, increased mechanical strength, and enhanced thermal stability. But, both the PFSA and sulfonated hydrocarbon membranes needed liquid H2O and humidification.202 Phosphoric acid (PA) doped poly benzimidazole is a commonly used membrane for HT-PEMFC. Phosphoric acid exhibits high proton conductivity, increasing the polymer's conductivity, and some of the merits of PA are good chemical stability, low gas permeability, good thermal stability, etc.203 Yang et al.204 found that methanol crossover is nil in multilayer sulphonated poly(ether ether ketone) (SPEEK) thin film. Membranes are made through the solution casting technique by blending PVA and different concentrations of chitosan. When this PVA-blended chitosan is crosslinked, the thermal and chemical properties are enhanced, and the swelling ratio decreases.185 The PEs were proved to overcome some of the significant disadvantages like low current density and low-pressure and partial load plaguing the alkaline electrolyser.
6.3 Electrical double layer capacitor (EDLC)
An electrical double-layer capacitor is a rechargeable and clean energy storage system with significant advantages like large capacitance, higher power density, extended cycle life relative to the secondary batteries, low maintenance requirements and good safety.111 It is made of two carbon electrodes (porous activated) with a large surface area inserted in an electrolytic solution.205 The electrical double layer between two electrodes is responsible for storing the energy in the EDLC. The surface structure of the electrode, the potential between the interface charges, and the electrolyte composition are the factors that influence the electrical double layer.19,206 Electrode resistance and conductivity of electrolytes influence the internal resistance and capacitance of EDLCs. The significant point here is that the working voltage of EDLC is directly proportional to the breakdown voltage of the electrolyte used. The charge–discharge characteristics are crucial in determining the performance of EDLCs.207 Polymer electrolytes have replaced liquid ones in the EDLC because of their flexibility, long-term safety, high power density, low risk of leakage, and low cost.19
Organic electrolytes are used in industry mainly because they produce a voltage of 2.7 to 2.8 V, which is used for high electrical energy production.185 PEO was the first PE found, and it was introduced into the EDLC by Lewandowski.208 Due to their low ionic conductivity, SPEs were ruled out, and a new class of polymer electrolytes (GPEs) was prepared. Methylcellulose (GPE) is an example that exhibited conductivity of 2.1 × 10−6 S cm−1 when plasticized with NH4NO3.111 The addition of LiCF3SO3 in poly(ethyl methacrylate) (PEMA)-based polymer electrolytes resulted in ionic conductivity of about 7.20 × 10−6 S cm−1 at room temperature.209 However, the addition of ammonium to the PEMA PE led to enhanced ionic conductivity from 8.6 × 10−11 S cm−1 to 1.02 × 10−5 S cm−1.210
Many works have been done to utilize chitosan-based electrolytes for their abundance and affordability. Chitosan-based biopolymer PEs were plasticized using glycerol and ammonium salts, which resulted in enhanced ionic conductivity of about 1.34 × 10−4 S cm−1. GPEs, along with the incorporation of nano SiO2 developed for EDLC by Ortega et al., showed a high capacitance of about 71.7 Fg−1, whereas unfilled GPE showed 41.6 Fg−1.211 Song et al.212 utilized TiO2 nanofiller and synthesized PVDF-HFP/PMMA-based polymer electrolytes, which possessed enhanced ionic conductivity, thermal stability, electrochemical stability and reduced shrinkage of the polymer electrolyte. Zheng et al.213 enhanced the ionic conductivity and mechanical strength by incorporating benzophenone into the ionic liquid.
7. Conclusion and future perspectives
This review article comprehensively addresses the history and evolution of polymer electrolytes in energy storage applications and their contribution to real-world applications like DSSCs, fuel cells, and electrical double-layer capacitors. The advantages of polymer electrolytes over inorganic liquid electrolytes have been presented, justifying the use of solid polymer electrolytes. Important considerations about selecting the polymer as an electrolyte have been discussed. The different classifications of polymer electrolytes, such as dry solid polymer electrolytes, gel polymer electrolytes, and composite-based solid polymer electrolytes, are explained in detail for clear understanding of the different types of PEs. Generally, GPEs may be useful for achieving good ionic conductivities at the cost of mechanical properties, which can be retained using different ideas like the utilization of fillers or block copolymers/blends. In contrast, CSPEs and NCPEs exhibit high conductivities and good mechanical properties because of the action/interaction of fillers added to the polymer matrix. In GPEs, NCPEs and CSPEs the conductivity is better than in SPEs through disrupting the crystalline regions/improving the amorphous regions. This is because the ion transport in amorphous regions is better than in the crystalline region, which has been highlighted. Therefore, researchers are recommended to work towards improving the amorphous content of PEs to have better properties.
Methodologies like solvent casting, hot pressing, electrospinning, the extraction–activation method, and in situ polymerization in the preparation of PE films were critically reviewed. Out of these, solvent casting and spin-coating techniques are widely used to prepare polymer electrolyte films at the lab scale. Some attention has been paid to the basic property requirements of polymer electrolytes like ionic conductivity, electrochemical stability, thermal stability, and mechanical stability. The dependence of conductivity on dielectric constant, temperature and ion dissociation energy was explored thoroughly. The existing ion transport models, like the Arrhenius model and VTF model, have been explained in detail, from which we have pointed out the influence of the amorphous region over the DC ionic conductivity. However, the ion conduction mechanism is not fully understood yet. Also, the coupling and decoupling mechanism of segmental mobility and ionic conductivity have been emphasized appropriately through the Arrhenius and VTF models. The different qualities and advantages of polymer electrolytes have been reviewed, and deep insight has been provided into the essential properties of PEs. Electrical impedance spectroscopy and impedance plots are discussed in detail. Experimental and theoretical aspects of EIS have been elucidated, and the determination of conductivity through the impedance plot has been revealed. Moreover, the Bruce–Vincent method and Wagner's polarization method are discussed in order to obtain the transference number of the polymer electrolyte which seems to be crucial. The significance of voltammetry techniques has been reviewed and analyzed in detail.
Lithium-polymer batteries are a currently emerging technology with limitations that need certain improvements and developments. The ionic conductivity of contemporary polymer electrolytes is not sufficient to employ them in high-energy applications. We know that the amorphous nature of the polymer plays a significant role in ionic conductivity. Therefore, we could use novel materials like elastomers or rubbers as electrolytic membranes for Li-pol batteries, which could be effective enough to obtain the necessary properties. Researchers have found that liquid crystal polymer interconnected with elastomer could be helpful as a PE.213 However, the use of elastomers or rubber as PE is currently being researched. This elastomeric electrolyte could be the future hope for taking lithium polymer batteries to another dimension. Rubbers have lower Tg and higher amorphous portions than ordinary polymers used as PEs. Consequently, these novel PEs based on rubbers/elastomers in the future could help Li-pol batteries to be used in e-vehicles and many other advanced applications requiring high ionic conductivity. Therefore, we could think about replacing PEs with rubber electrolytes, which would be the future. Also, the sustainability of PEs is critically considered, and research is ongoing. The use of natural, biodegradable and chemically recyclable polymers and sustainable fillers to reduce the environmental impacts of lithium batteries has been critically reviewed.214 This sustainable polymer/filler utilization in polymer electrolytes acts as an environmentally friendly approach and causes less harm to nature. Achieving ionic conductivity and mechanical strength is not easy; researchers can work to decouple the relationship between conductivity and mechanical stability, or they can try to achieve both properties without deterioration of one property. If we try to develop SPE, which possesses high ionic conductivity, it will definitely show lower mechanical strength, and vice versa. For this issue, we have several techniques like blending, co-polymerization, cross-linking, composites, nanofillers, etc., to improve both properties. Nowadays, researchers are working in vitrimeric polymer electrolytes which will possess good mechanical stability along with high ionic conductivity, which has to be optimized. Vitrimer chemistry utilizes dynamic bonds forming associative covalent adaptable networks, which helps in recycling of the polymers and upcycles the mechanical strength of the post-consumer recyclate polymer. The trade-off between conductivity and mechanical stability depends on the dynamic crosslinker, its molecular weight and so on. These vitrimeric electrolytes are anticipated to exhibit self-healing, recyclability, and shape memory characteristics. Therefore, this could be a viable solution for improving mechanical stability.215
With respect to SPEs, LiTFSI is the commonly used salt because of its electrochemical stability and as the TFSI anion possesses delocalized electrons, which results in high transport/coordination of cations. Novel salts which could replace LiTFSI with more ESW and highly delocalized electrons could be explored. Nowadays, ionic liquids are becoming commercially viable. These ionic liquids contribute to the liquid state of the GPEs, in which we could see higher ionic conductivity and mobility. These ionic liquids can be utilized, or novel ionic liquids can be synthesized to get commercially viable and useful PEs. Speaking of commercialization, cost is the major concern when developing electrolytes for pragmatic applications. However, most of the novel ideas remain in the lab. Therefore, researchers should not only focus on the novelty but also on the commercial concerns of the polymer electrolytes. Concerning the thickness of the polymer electrolyte films, we can try to use ultrathin polymer films, which could enhance ion transport and reduce the system's impedance. But at the same time, we should ensure the optimum mechanical properties, as said earlier. This ultrathin film concept seems to drastically improve energy density. However, mechanical strength is lacking. Therefore, researchers have proposed a thin, strong matrix film to get rid of this lower mechanical and electrochemical stability. However, the interfacial stability and electrode portion must be improved.
Author contributions
R. P. S.: conceptualization; data curation; formal analysis; investigation; methodology; software; validation; visualization; roles/writing – original draft. V. P. V.: writing – review & editing. V. K.: supervision; review & editing A. S. A.: project administration; resources; supervision; validation; visualization; writing – review & editing.
Data availability
No primary research results, software or code have been included and no new data were generated or analysed as part of this review.
Conflicts of interest
There are no conflicts to declare.
Acknowledgements
ASA extends sincere gratitude to the Science and Engineering Board (SERB), DST, Government of India, and Board of Research in Nucear Sciences (BRNS) for their generous funding of this project under grants CRG/2018/000362 and no. 2012/34/31/BRNS/1039, respectively. We also acknowledge the Ministry of Human Resource Development for establishing the Centre of Excellence in FAST (F. no. 5-7/2014-TS-VII).
References
- S. Megahed and B. Scrosati, Lithium-ion rechargeable batteries, J. Power Sources, 1994, 51(1–2), 79–104 CrossRef CAS
.
- X. Yu and A. Manthiram, A review of composite polymer-ceramic electrolytes for lithium batteries, Energy Storage Mater., 2021, 34, 282–300 CrossRef
.
- A. Manthiram, A reflection on lithium-ion battery cathode chemistry, Nat. Commun., 2020, 11(1), 1550 CrossRef CAS PubMed
.
- T. Kim, W. Song, D. Y. Son, L. K. Ono and Y. Qi, Lithium-ion batteries: outlook on present, future, and hybridized technologies, J. Mater. Chem. A, 2019, 7(7), 2942–2964 CAS
.
- J. B. Goodenough and K. S. Park, The Li-Ion Rechargeable Battery: A Perspective, J. Am. Chem. Soc., 2013, 135(4), 1167–1176 CAS
.
- H. J. Kim, T. Krishna, K. Zeb, V. Rajangam, C. V. V. M. Gopi and S. Sambasivam,
et al., A Comprehensive Review of Li-Ion Battery Materials and Their Recycling Techniques, Electronics, 2020, 9(7), 1161 CAS
.
- L. Long, S. Wang, M. Xiao and Y. Meng, Polymer electrolytes for lithium polymer batteries, J. Mater. Chem. A, 2016, 4(26), 10038–10069 RSC
.
- J. C. Bachman, S. Muy, A. Grimaud, H. H. Chang, N. Pour and S. F. Lux,
et al., Inorganic Solid-State Electrolytes for Lithium Batteries: Mechanisms and Properties Governing Ion Conduction, Chem. Rev., 2016, 116(1), 140–162 CAS
.
- N. Nitta, F. Wu, J. T. Lee and G. Yushin, Li-ion battery materials: present and future, Mater. Today, 2015, 18(5), 252–264 CAS
.
- A. Manthiram, X. Yu and S. Wang, Lithium battery chemistries enabled by solid-state electrolytes, Nat. Rev. Mater., 2017, 2(4), 16103 CAS
.
- J. Janek and W. G. Zeier, A solid future for battery development, Nat. Energy, 2016, 1(9), 16141 Search PubMed
.
-
M. B. Armand, J. M. Chabagno and M. Duclot, Second International Meeting on Solid Electrolytes, St Andrews, Scotland, 1978, pp. 20–22 Search PubMed
.
- T. Wulandari, D. Fawcett, S. B. Majumder and G. E. J. Poinern, Lithium–based batteries, history, current status, challenges, and future perspectives, Battery Energy, 2023, 2(6), 20230030 Search PubMed
.
- S. Z. Z. Abidin, A. M. M. Ali, O. H. Hassan and M. Z. A. Yahya, Electrochemical Studies on Cellulose Acetate-LiBOB Polymer Gel Electrolytes, Int. J. Electrochem. Sci., 2013, 8(5), 7320–7326 CAS
.
- S. B. Aziz and Z. H. Z. Abidin, Ion–transport study in nanocomposite solid polymer electrolytes based on chitosan: Electrical and dielectric analysis, J. Appl. Polym. Sci., 2015, 132(15), 41774 Search PubMed
.
- S. B. Aziz and Z. H. Z. Abidin, Electrical Conduction Mechanism in Solid Polymer Electrolytes: New Concepts to Arrhenius Equation, J. Soft Matter, 2013, 2013, 1–8 Search PubMed
.
- J. Weston and B. Steele, Effects of inert fillers on the mechanical and electrochemical properties of lithium salt-poly(ethylene oxide) polymer electrolytes, Solid State Ionics, 1982, 7(1), 75–79 CAS
.
- S. B. Aziz, Li+ ion conduction mechanism in poly (ε-caprolactone)-based polymer electrolyte, Iran. Polym. J., 2013, 22(12), 877–883 CAS
.
- K. S. Ngai, S. Ramesh, R. K. Juan and J. C, A review of polymer electrolytes: fundamental, approaches and applications, Ionics, 2016, 22(8), 1259–1279 CAS
.
- E. L. Chávez, R. Oviedo-Roa, G. Contreras-Pérez, J. M. Martínez-Magadán and F. L. Castillo-Alvarado, Theoretical studies of ionic conductivity of crosslinked chitosan membranes, Int. J. Hydrogen Energy, 2010, 35(21), 12141–12146 Search PubMed
.
- C. V. S. Rao, M. Ravi, V. Raja, P. B. Bhargav, A. K. Sharma and V. V. R. N. Rao, Preparation and characterization of PVP-based polymer electrolytes for solid-state battery applications, Iran. Polym. J., 2012, 21(8), 531–536 CrossRef
.
- S. B. Aziz, Z. H. Z. Abidin and A. K. Arof, Effect of silver nanoparticles on the DC conductivity in chitosan–silver triflate polymer electrolyte, Phys. B, 2010, 405(21), 4429–4433 CrossRef CAS
.
- T. J. Singh and S. V. Bhat, Morphology and conductivity studies of a new solid polymer electrolyte: (PEG)xLiClO4, Bull. Mater. Sci., 2003, 26(7), 707–714 CrossRef CAS
.
- T. Itoh, Y. Mitsuda, T. Ebina, T. Uno and M. Kubo, Solid polymer electrolytes composed of polyanionic lithium salts and polyethers, J. Power Sources, 2009, 189(1), 531–535 CrossRef CAS
.
- K. Venkatesh, I. Jenova, S. Karthikeyan, S. Madeswaran, R. Bhuvaneswari and S. Nithya and J. Sheeba, Influence of plasticizer on conductivity and dielectric properties of guar gum-based sodium ion conducting polymer electrolyte, Mater. Today: Proc., 2023 DOI:10.1016/j.matpr.2022.12.236
.
- S. Das and A. Ghosh, Effect of plasticizers on ionic conductivity and dielectric relaxation of PEO-LiClO4 polymer electrolyte, Electrochim. Acta, 2015, 171, 59–65 CrossRef CAS
.
- S. B. Aziz, Z. H. Z. Abidin and M. F. Z. Kadir, Innovative method to avoid the reduction of silver ions to silver nanoparticles $\left({\rm A}{{{\rm g}}^{+}}\to {\rm Ag}{}\deg \right)$ in silver ion conducting based polymer electrolytes, Phys. Scr., 2015, 90(3), 035808 CrossRef
.
- L. Zang, J. Luo, J. Guo, H. Liu and J. Ru, Preparation and characterization of poly(ethylene glycol)/organo-vermiculite nanocomposite polymer electrolytes, Polym. Bull., 2010, 65(7), 669–680 CrossRef
.
-
Y. Wang, Ionic Transport and Dielectric Relaxation in Polymer Electrolytes, in Dielectric Properties of Ionic Liquids, ed. M. Paluch, Springer International Publishing, Cham, 2016, pp. 131–156, [cited 2024 Dec 6] (advances in dielectrics). Available from: https://link.springer.com/10.1007/978-3-319-32489-0_6 Search PubMed
.
- M. Walter, M. V. Kovalenko and K. V. Kravchyk, Challenges and benefits of post-lithium-ion batteries, New J. Chem., 2020, 44(5), 1677–1683 RSC
.
- G. Li, B. Huang, Z. Pan, X. Su, Z. Shao and L. An, Advances in three-dimensional graphene-based materials: configurations, preparation and application in secondary metal (Li, Na, K, Mg, Al)-ion batteries, Energy Environ. Sci., 2019, 12(7), 2030–2053 RSC
.
- M. Armand, The history of polymer electrolytes, Solid State Ionics, 1994, 69(3–4), 309–319 CrossRef CAS
.
- D. E. Fenton, J. M. Parker and P. V. Wright, Complexes of alkali metal ions with poly(ethylene oxide), Polymer, 1973, 14(11), 589 CrossRef CAS
.
- J. Mindemark, M. J. Lacey, T. Bowden and D. Brandell, Beyond PEO—Alternative host materials for Li + -conducting solid polymer electrolytes, Prog. Polym. Sci., 2018, 81, 114–143 CrossRef CAS
.
- D. H. Kuo, R. Lo, T. H. Hsueh, D. J. Jan and C. H. Su, LiSnOS/gel polymer hybrid electrolyte for the safer and performance-enhanced solid-state LiCoO2/Li lithium-ion battery, J. Power Sources, 2019, 429, 89–96 CrossRef CAS
.
- D. E. Fenton, J. M. Parker and P. V. Wright, Complexes of alkali metal ions with poly(ethylene oxide), Polymer, 1973, 14(11), 589 CrossRef CAS
.
- P. A. R. D. Jayathilaka, M. A. K. L. Dissanayake, I. Albinsson and B. E. Mellander, Effect of nano-porous Al2O3 on thermal, dielectric and transport properties of the (PEO)9LiTFSI polymer electrolyte system, Electrochim. Acta, 2002, 47(20), 3257–3268 CrossRef CAS
.
- M. V. Reddy, A. Mauger, C. M. Julien, A. Paolella and K. Zaghib, Brief History of Early Lithium-Battery Development, Materials, 2020, 13(8), 1884 CrossRef CAS PubMed
.
- Y. Chatani and S. Okamura, Crystal structure of poly(ethylene oxide)—sodium iodide complex, Polymer, 1987, 28(11), 1815–1820 CrossRef CAS
.
- G. Feuillade and Ph. Perche, Ion-conductive macromolecular gels and membranes for solid lithium cells, J. Appl. Electrochem., 1975, 5(1), 63–69 CrossRef CAS
.
- S. Skaarup, Mixed phase solid electrolytes, Solid State Ionics, 1988, 28–30, 975–978 CrossRef
.
- W. Wieczorek, K. Such, H. Wyciślik and J. Płocharski, Modifications of crystalline structure of peo polymer electrolytes with ceramic additives, Solid State Ionics, 1989, 36(3–4), 255–257 CrossRef CAS
.
-
V. Dinoto, E. Negro, S. Lavina and M. Vittadello, Hybrid inorganic–organic polymer electrolytes, in Polymer Electrolytes, Elsevier, 2010, pp. 219–277 [cited 2024 Dec 6]. Available from: https://linkinghub.elsevier.com/retrieve/pii/B9781845697723500066 Search PubMed
.
- G. E. Blomgren, The Development and Future of Lithium Ion Batteries, J. Electrochem. Soc., 2017, 164(1), A5019–A5025 CrossRef CAS
.
- J. Muldoon, C. B. Bucur, N. Boaretto, T. Gregory and V. Di Noto, Polymers: Opening Doors to Future Batteries, Polym. Rev., 2015, 55(2), 208–246 CrossRef CAS
.
- A. Arya and A. L. Sharma, Electrolyte for energy storage/conversion (Li+, Na+, Mg2+) devices based on PVC and their associated polymer: a comprehensive review, J. Solid State Electrochem., 2019, 23(4), 997–1059 CrossRef CAS
.
- L. Han, M. L. Lehmann, J. Zhu, T. Liu, Z. Zhou and X. Tang,
et al., Recent Developments and Challenges in Hybrid Solid Electrolytes for Lithium-Ion Batteries, Front. Energy Res., 2020, 8, 202 CrossRef
.
- Z. H. Li, H. P. Zhang, P. Zhang, G. C. Li, Y. P. Wu and X. D. Zhou, Effects of the porous structure on conductivity of nanocomposite polymer electrolyte for lithium ion batteries, J. Membr. Sci., 2008, 322(2), 416–422 CrossRef CAS
.
- J. Sakuda, E. Hosono, M. Yoshio, T. Ichikawa, T. Matsumoto and H. Ohno,
et al., Liquid–Crystalline Electrolytes for Lithium–Ion Batteries: Ordered Assemblies of a Mesogen–Containing Carbonate and a Lithium Salt, Adv. Funct. Mater., 2015, 25(8), 1206–1212 CrossRef CAS
.
- S. M. Alauddin, N. F. K. Aripin, T. S. Velayutham and A. Martinez-Felipe, Liquid Crystalline Copolymers Containing Sulfonic and Light-Responsive Groups: From Molecular Design to Conductivity, Molecules, 2020, 25(11), 2579 CrossRef CAS PubMed
.
- S. Wang, X. Liu, A. Wang, Z. Wang, J. Chen and Q. Zeng,
et al., An ionic liquid crystal-based solid polymer electrolyte with desirable ion-conducting channels for superior performance ambient-temperature lithium batteries, Polym. Chem., 2018, 9(37), 4674–4682 RSC
.
- G. X. Wang, L. Yang, J. Z. Wang, H. K. Liu and S. X. Dou, Enhancement of Ionic Conductivity of PEO Based Polymer Electrolyte by the Addition of Nanosize Ceramic Powders, J. Nanosci. Nanotechnol., 2005, 5(7), 1135–1140 CrossRef CAS PubMed
.
- K. Xu, Electrolytes and Interphases in Li-Ion Batteries and Beyond, Chem. Rev., 2014 Dc 10, 114(23), 11503–11618 Search PubMed
.
- J. Zhang, J. Yang, T. Dong, M. Zhang, J. Chai and S. Dong,
et al., Aliphatic Polycarbonate–Based Solid–State Polymer Electrolytes for Advanced Lithium Batteries: Advances and Perspective, Small, 2018, 14(36), 1800821 CrossRef PubMed
.
- J. Zhang, J. Zhao, L. Yue, Q. Wang, J. Chai and Z. Liu,
et al., Safety–Reinforced Poly(Propylene Carbonate)–Based All–Solid–State Polymer Electrolyte for Ambient–Temperature Solid Polymer Lithium Batteries, Adv. Energy Mater., 2015, 5(24), 1501082 CrossRef
.
- M. P. Blatt and D. T. Hallinan, Polymer Blend Electrolytes for Batteries and Beyond, Ind. Eng. Chem. Res., 2021, 60(48), 17303–17327 CrossRef CAS
.
- S. Ramesh, C. W. Liew, E. Morris and R. Durairaj, Effect of PVC on ionic conductivity, crystallographic structural, morphological and thermal characterizations in PMMA–PVC blend-based polymer electrolytes, Thermochim. Acta, 2010, 511(1–2), 140–146 CrossRef CAS
.
- I. Nicotera, L. Coppola, C. Oliviero, M. Castriota and E. Cazzanelli, Investigation of ionic conduction and mechanical properties of PMMA–PVdF blend-based polymer electrolytes☆, Solid State Ionics, 2006, 177(5–6), 581–588 CrossRef CAS
.
- P. Senthil Kumar, A. Sakunthala, M. V. Reddy and M. Prabu, Structural, morphological, electrical and electrochemical study on plasticized PVdF-HFP/PEMA blended polymer electrolyte for lithium polymer battery application, Solid State Ionics, 2018, 319, 256–265 CrossRef CAS
.
- N. Angulakhsmi, S. Thomas, J. R. Nair, R. Bongiovanni, C. Gerbaldi and A. M. Stephan, Cycling profile of innovative nanochitin-incorporated poly (ethylene oxide) based electrolytes for lithium batteries, J. Power Sources, 2013, 228, 294–299 CrossRef CAS
.
- A. Panday, S.Mullin, E. D. Gomez, N. Wanakule, V. L. Chen, A. Hexemer, J. Pople and N. P. Balsara, Effect of molecular weight and salt concentration on conductivity of block copolymer electrolytes, Macromolecules, 2009, 42(13), 4632–4637 CrossRef CAS
.
- M. Chintapalli, X. C. Chen, J. L. Thelen, A. A. Teran, X. Wang, B. A. Garetz and N. P. Balsara, Effect of Grain Size on the Ionic Conductivity of a Block Copolymer Electrolyte, Macromolecules, 2014, 47(15), 5424–5431 CrossRef CAS
.
- E. D. Gomez, A. Panday, E. H. Feng, V. Chen, G. M. Stone and A. M. Minor,
et al., Effect of Ion Distribution on Conductivity of Block Copolymer Electrolytes, Nano Lett., 2009, 9(3), 1212–1216 CrossRef CAS PubMed
.
- D. K. Pradhan, B. K. Samantaray, R. N. P. Choudhary and A. K. Thakur, Effect of plasticizer on microstructure and electrical properties of a sodium ion conducting composite polymer electrolyte, Ionics, 2005, 11(1–2), 95–102 CrossRef CAS
.
- D. K. Pradhan, R. N. P. Choudhary and B. K. Samantaray, Studies of dielectric and electrical properties of plasticized polymer nanocomposite electrolytes, Mater. Chem. Phys., 2009, 115(2–3), 557–561 CrossRef CAS
.
- K. Pandey, N. Asthana, M. M. Dwivedi and S. K. Chaturvedi, Effect of Plasticizers on Structural and Dielectric Behaviour of [PEO + (NH4)2 C4 H8 (COO)2 ] Polymer Electrolyte, J. Polym., 2013, 2013, 1–12 Search PubMed
.
- H. T. Ahmed, V. J. Jalal, D. A. Tahir, A. H. Mohamad and O. G. h. Abdullah, Effect of PEG as a plasticizer on the electrical and optical properties of polymer blend electrolyte MC-CH-LiBF4 based films, Results Phys., 2019, 15, 102735 CrossRef
.
- S. S. Muobom, A.-M. S. Umar, A.-P. Brolin and Y. Soongseok, A Review on Plasticizers and Eco-Friendly Bioplasticizers: Biomass Sources and Market, Int. J. Eng. Res. Sci. Technol., 2020, 9(5), 1138–1144 Search PubMed
.
- D. K. Pradhan, R. N. P. Choudhary and B. K. Samantaray, Studies of Dielectric Relaxation and AC Conductivity Behavior of Plasticized Polymer Nanocomposite Electrolytes, Int. J. Electrochem. Sci., 2008, 3(5), 597–608 CrossRef CAS
.
- H. Ericson, C. Svanberg, A. Brodin, A. M. Grillone, S. Panero and B. Scrosati,
et al. Poly(methyl methacrylate)-based protonic gel electrolytes: a spectroscopic study, Electrochim. Acta, 2000, 45(8–9), 1409–1414 CAS
.
- D. T. Hallinan and N. P. Balsara, Polymer Electrolytes, Annu. Rev. Mater. Res., 2013, 43(1), 503–525 CAS
.
- A. Subramania, N. T. K. Sundaram, A. R. Priya, R. Gangadharan and T. Vasudevan, Preparation of a microporous gel polymer electrolyte with a novel preferential polymer dissolution process for Li–ion batteries, J. Appl. Polym. Sci., 2005, 98(5), 1891–1896 CAS
.
- D. H. Kuo, R. Lo, T. H. Hsueh, D. J. Jan and C. H. Su, LiSnOS/gel polymer hybrid electrolyte for the safer and performance-enhanced solid-state LiCoO2/Li lithium-ion battery, J. Power Sources, 2019, 429, 89–96 CAS
.
- Y. Gambe, H. Kobayashi, K. Iwase, S. Stauss and I. Honma, A photo-curable gel electrolyte ink for 3D-printable quasi-solid-state lithium-ion batteries, Dalton Trans., 2021, 50(45), 16504–16508 CAS
.
- G. T. Kim, G. B. Appetecchi, M. Carewska, M. Joost, A. Balducci, M. Winter and S. Passerini, UV cross-linked, lithium-conducting ternary polymer electrolytes containing ionic liquids, J. Power Sources, 2010, 195(18), 6130–6137 CAS
.
- M. Wetjen, G. T. Kim, M. Joost, G. B. Appetecchi, M. Winter and S. Passerini, Thermal and electrochemical properties of PEO-LiTFSI-Pyr14TFSI-based composite cathodes, incorporating 4 V-class cathode active materials, J. Power Sources, 2014, 246, 846–857 CAS
.
- K. Yin, Z. Zhang, X. Li, L. Yang, K. Tachibana and S. Hirano, Polymer electrolytes based on dicationic polymeric ionic liquids: application in lithium metal batteries, J. Mater. Chem. A, 2015, 3(1), 170–178 CAS
.
- V. Bocharova and A. P. Sokolov, Perspectives for Polymer Electrolytes: A View from Fundamentals of Ionic Conductivity, Macromolecules, 2020, 53(11), 4141–4157 CAS
.
- S. R. Mohapatra, A. K. Thakur and R. N. P. Choudhary, Effect of nanoscopic confinement on improvement in ion conduction and stability properties of an intercalated polymer nanocomposite electrolyte for energy storage applications, J. Power Sources, 2009, 191(2), 601–613 CAS
.
- P. Thomas, S. Satapathy, K. Dwarakanath and K. B. R. Varma, Dielectric properties of poly(vinylidene fluoride)/CaCu3Ti4O12 nanocrystal composite thick films, eXPRESS Polym. Lett., 2010, 4(10), 632–643 CAS
.
- A. Arya and A. L. Sharma, Polymer electrolytes for lithium ion batteries: a critical study, Ionics, 2017, 23(3), 497–540 CAS
.
- Z. Shen, Y. Cheng, S. Sun, X. Ke, L. Liu and Z. Shi, The critical role of inorganic nanofillers in solid polymer composite electrolyte for Li+ transportation, Carbon Energy, 2021, 3(3), 482–508 CAS
.
- S. Tang, W. Guo and Y. Fu, Advances in Composite Polymer Electrolytes for Lithium Batteries and Beyond, Adv. Energy Mater., 2021, 11(2), 2000802 CAS
.
- C. G. Tan, W. O. Siew, W. L. Pang, Z. Osman and K. W. Chew, The effects of ceramic fillers on the PMMA-based polymer electrolyte systems, Ionics, 2007, 13(5), 361–364 CAS
.
- M. M. Borgohain, T. Joykumar and S. V. Bhat, Studies on a nanocomposite solid polymer electrolyte with hydrotalcite as a filler, Solid State Ionics, 2010, 181(21–22), 964–970 CrossRef CAS
.
-
S. Shankar and J. W. Rhim, Bionanocomposite Films for Food Packaging Applications, in Reference Module in Food Science, Elsevier, 2018, p. B9780081005965218751 [cited 2024 Dec 6]. Available from: https://linkinghub.elsevier.com/retrieve/pii/B9780081005965218751 Search PubMed
.
- Z. Wen, Thermal, electrical, and mechanical properties of composite polymer electrolytes based on cross-linked poly(ethylene oxide-co-propylene oxide) and ceramic filler, Solid State Ionics, 2003, 160(1–2), 141–148 CrossRef CAS
.
- T. Itoh, Composite polymer electrolytes based on poly(ethylene oxide), hyperbranched polymer, BaTiO3 and LiN(CF3SO2)2, Solid State Ionics, 2003, 156(3–4), 393–399 CrossRef CAS
.
- Z. H. Li, H. P. Zhang, P. Zhang, G. C. Li, Y. P. Wu and X. D. Zhou, Effects of the porous structure on conductivity of nanocomposite polymer electrolyte for lithium ion batteries, J. Membr. Sci., 2008, 322(2), 416–422 CrossRef CAS
.
- S. Choudhary and R. J. Sengwa, Dielectric spectroscopy and confirmation of ion conduction mechanism in direct melt compounded hot-press polymer nanocomposite electrolytes, Ionics, 2011, 17(9), 811–819 CAS
.
- M. Deka and A. Kumar, Electrical and electrochemical studies of poly(vinylidene fluoride)–clay nanocomposite gel polymer electrolytes for Li-ion batteries, J. Power Sources, 2011, 196(3), 1358–1364 CAS
.
- M. Hema, S. Selvasekerapandian, G. Hirankumar, A. Sakunthala, D. Arunkumar and H. Nithya, Structural and thermal studies of PVA:NH4I, J. Phys. Chem. Solids, 2009, 70(7), 1098–1103 CAS
.
- M. Walkowiak, A. Zalewska, T. Jesionowski, D. Waszak and B. Czajka, Effect of chemically modified silicas on the properties of hybrid gel electrolyte for Li-ion batteries, J. Power Sources, 2006, 159(1), 449–453 CAS
.
- F. Gebert, J. Knott, R. Gorkin, S. L. Chou and S. X. Dou, Polymer electrolytes for sodium-ion batteries, Energy Storage Mater., 2021, 36, 10–30 Search PubMed
.
- S. Roy and N. Singha, Polymeric Nanocomposite Membranes for Next Generation Pervaporation Process: Strategies, Challenges and Future Prospects, Membranes, 2017, 7(3), 53 Search PubMed
.
- N. Verdier, G. Foran, D. Lepage, A. Prébé, D. Aymé-Perrot and M. Dollé, Challenges in Solvent-Free Methods for Manufacturing Electrodes and Electrolytes for Lithium-Based Batteries, Polymers, 2021, 13(3), 323 CAS
.
- P. Penjumras, R. AbdulRahman, R. A. Talib and K. Abdan, Effect of silanecoupling agent on properties of biocomposites based on poly(lactic acid)and durian rind cellulose, IOP Conf. Ser.: Mater. Sci. Eng., 2016, 137, 012006 Search PubMed
.
- A. Chandra, Hot pressed K+ ion conducting solid polymer electrolytes: synthesis, ion conduction and polymeric battery fabrication, Indian J. Phys., 2016, 90(7), 759–765 Search PubMed
.
- H. Junoh, J. Jaafar, M. N. A. Mohd Norddin, A. F. Ismail, M. H. D. Othman and M. A. Rahman,
et al. A Review on the Fabrication of Electrospun Polymer Electrolyte Membrane for Direct Methanol Fuel Cell, J. Nanomater., 2015, 2015(1), 690965 Search PubMed
, Bhattacharya RN, editor.
- S. N. Banitaba, D. Semnani, E. Heydari-Soureshjani, B. Rezaei, A. A. Ensafi and A. Taghipour-Jahromi, Novel electrospun polymer electrolytes incorporated with Keggin–type hetero polyoxometalate fillers as solvent–free electrolytes for lithium ion batteries, Polym. Int., 2020, 69(8), 675–687 CAS
.
- J. R. Kim, S. W. Choi, S. M. Jo, W. S. Lee and B. C. Kim, Electrospun PVdF-based fibrous polymer electrolytes for lithium ion polymer batteries, Electrochim. Acta, 2004, 50(1), 69–75 CAS
.
- F. Huang, Y. Xu, B. Peng, Y. Su, F. Jiang and Y. L. Hsieh,
et al., Coaxial Electrospun Cellulose-Core Fluoropolymer-Shell Fibrous Membrane from Recycled Cigarette Filter as Separator for High Performance Lithium-Ion Battery, ACS Sustainable Chem. Eng., 2015, 3(5), 932–940 CAS
.
- J. Zhu, Y. Lu, C. Chen, Y. Ge, S. Jasper and J. D. Leary,
et al., Porous one-dimensional carbon/iron oxide composite for rechargeable lithium-ion batteries with high and stable capacity, J. Alloys Compd., 2016, 672, 79–85 CAS
.
- H. Wang, H. Huang and S. L. Wunder, Novel Microporous Poly(vinylidene fluoride) Blend Electrolytes for Lithium-Ion Batteries, J. Electrochem. Soc., 2000, 147(8), 2853 CAS
.
- L. Sun, K. Higaki and R. C. McDonald, Performance characteristics of lithium-ion cells using in situ polymerized electrolytes, J. Power Sources, 1997, 68(2), 352–356 CrossRef CAS
.
- Y. Cui, J. Chai, H. Du, Y. Duan, G. Xie and Z. Liu,
et al., Facile and Reliable in Situ Polymerization of Poly(Ethyl Cyanoacrylate)-Based Polymer Electrolytes toward Flexible Lithium Batteries, ACS Appl. Mater. Interfaces, 2017, 9(10), 8737–8741 CrossRef CAS PubMed
.
- L. Sun, K. Higaki and R. C. McDonald, Performance characteristics of lithium-ion cells using in situ polymerized electrolytes, J. Power Sources, 1997, 68(2), 352–356 CrossRef CAS
.
- X. Lin, M. Salari, L. M. R. Arava, P. M. Ajayan and M. W. Grinstaff, High temperature electrical energy storage: advances, challenges, and frontiers, Chem. Soc. Rev., 2016, 45(21), 5848–5887 RSC
.
- A. Jurkane and S. Gaidukov, Preparation and characterization of hot-pressed Li+ ion conducting PEO composite electrolytes, IOP Conf. Ser.: Mater. Sci. Eng., 2016, 111, 012016 Search PubMed
.
- C. Capiglia, Effects of nanoscale SiO2 on the thermal and transport properties of solvent-free, poly(ethylene oxide) (PEO)-based polymer electrolytes, Solid State Ionics, 1999, 118(1–2), 73–79 CrossRef CAS
.
-
K. H. Kamarudin, M. Hassan and M. I. N. Isa, Lightweight and Flexible Solid-State EDLC based on Optimized CMC-NH4NO3 Solid Bio-Polymer Electrolyte, 2018 Search PubMed
.
- L. Chen, S. Venkatram, C. Kim, R. Batra, A. Chandrasekaran and R. Ramprasad, Electrochemical Stability Window of Polymeric Electrolytes, Chem. Mater., 2019, 31(12), 4598–4604 CrossRef CAS
.
- C. Park, Electrochemical stability and conductivity enhancement of composite polymer electrolytes, Solid State Ionics, 2003, 159(1–2), 111–119 CrossRef CAS
.
- Y. Zhu, X. He and Y. Mo, Origin of Outstanding Stability in the Lithium Solid Electrolyte Materials: Insights from Thermodynamic Analyses Based on First-Principles Calculations, ACS Appl. Mater. Interfaces, 2015, 7(42), 23685–23693 CrossRef CAS PubMed
.
- W. D. Richards, L. J. Miara, Y. Wang, J. C. Kim and G. Ceder, Interface Stability in Solid-State Batteries, Chem. Mater., 2016, 28(1), 266–273 CrossRef CAS
.
- A. Méry, S. Rousselot, D. Lepage and M. Dollé, A Critical Review for an Accurate Electrochemical Stability Window Measurement of Solid Polymer and Composite Electrolytes, Materials, 2021, 14(14), 3840 CrossRef PubMed
.
- C. Capiglia, J. Yang, N. Imanishi, A. Hirano, Y. Takeda and O. Yamamoto, DSC study on the thermal stability of solid polymer electrolyte cells, J. Power Sources, 2003, 119–121, 826–832 CrossRef CAS
.
- W. Tang, S. Tang, C. Zhang, Q. Ma, Q. Xiang and Y. Yang,
et al., Simultaneously Enhancing the Thermal Stability, Mechanical Modulus, and Electrochemical Performance of Solid Polymer Electrolytes by Incorporating 2D Sheets, Adv. Energy Mater., 2018, 8(24), 1800866 Search PubMed
.
- Y. Zhao, L. Wang, Y. Zhou, Z. Liang, N. Tavajohi and B. Li,
et al., Solid Polymer Electrolytes with High Conductivity and Transference Number of Li Ions for Li–Based Rechargeable Batteries, Adv. Sci., 2021, 8(7), 2003675 CAS
.
- J. K. Kim, G. Cheruvally, X. Li, J. H. Ahn, K. W. Kim and H. J. Ahn, Preparation and electrochemical characterization of electrospun, microporous membrane-based composite polymer electrolytes for lithium batteries, J. Power Sources, 2008, 178(2), 815–820 CrossRef CAS
.
- H. S. Kim, K. S. Kum, W. I. Cho, B. W. Cho and H. W. Rhee, Electrochemical and physical properties of composite polymer electrolyte of poly(methyl methacrylate) and poly(ethylene glycol diacrylate), J. Power Sources, 2003, 124(1), 221–224 CAS
.
- S. J. Kwon, D. G. Kim, J. Shim, J. H. Lee, J. H. Baik and J. C. Lee, Preparation of organic/inorganic hybrid semi-interpenetrating network polymer electrolytes based on poly(ethylene oxide-co-ethylene carbonate) for all-solid-state lithium batteries at elevated temperatures, Polymer, 2014, 55(12), 2799–2808 CAS
.
- J. Shim, H. J. Kim, B. G. Kim, Y. S. Kim, D. G. Kim and J. C. Lee, 2D boron nitride nanoflakes as a multifunctional additive in gel polymer electrolytes for safe, long cycle life and high rate lithium metal batteries, Energy Environ. Sci., 2017, 10(9), 1911–1916 CAS
.
- J. T. Bamford, S. D. Jones, N. S. Schauser, B. J. Pedretti, L. W. Gordon and N. A. Lynd,
et al., Improved Mechanical Strength without Sacrificing Li-Ion Transport in Polymer Electrolytes, ACS Macro Lett., 2024, 13(5), 638–643 CAS
.
-
K. P. Barteau and G. H. Fredrickson, Poly(Glycidyl Ether)-Based Battery Electrolytes: Correlating Polymer Properties to Ion Transport, Santa Barbara, Calif., University of California, Santa Barbara, 2015 Search PubMed
.
- M. Kumar and S. S. Sekhon, Role of plasticizer's dielectric constant on conductivity modification of PEO–NH4F polymer electrolytes, Eur. Polym. J., 2002, 38(7), 1297–1304 CAS
.
- S. B. Aziz and Z. H. Z. Abidin, Electrical and morphological analysis of chitosan:AgTf solid electrolyte, Mater. Chem. Phys., 2014, 144(3), 280–286 CAS
.
- A. Arya and A. L. Sharma, Temperature and Salt-Dependent Dielectric Properties of Blend Solid Polymer Electrolyte Complexed with LiBOB, Macromol. Res., 2019, 27(4), 334–345 CAS
.
- C. A. Vincent, Ion transport in polymer electrolytes, Electrochim. Acta, 1995, 40(13–14), 2035–2040 CAS
.
- R. C. Agrawal and G. P. Pandey, Solid polymer electrolytes: materials designing and all-solid-state battery applications: an overview, J. Phys. Appl. Phys., 2008, 41(22), 223001 Search PubMed
.
- G. Mao, R. F. Perea, W. S. Howells, D. L. Price and M. L. Saboungi, Relaxation in polymer electrolytes on the nanosecond timescale, Nature, 2000, 405(6783), 163–165 Search PubMed
.
- H. Yang and N. Wu, Ionic conductivity and ion transport mechanisms of solid–state lithium–ion battery electrolytes: A review, Energy Sci. Eng., 2022, 10(5), 1643–1671 CrossRef CAS
.
- Z. Xue, D. He and X. Xie, Poly(ethylene oxide)-based electrolytes for lithium-ion batteries, J. Mater. Chem. A, 2015, 3(38), 19218–19253 RSC
.
- A. E. Gerdroodbar, H. Alihemmati, S. A. Safavi-Mirmahaleh, M. Golshan, R. Damircheli and S. N. Eliseeva,
et al., A review on ion transport pathways and coordination chemistry between ions and electrolytes in energy storage devices, J. Energy Storage, 2023, 74, 109311 CrossRef
.
- R. Baskaran, S. Selvasekarapandian, N. Kuwata, J. Kawamura and T. Hattori, Structure, thermal and transport properties of PVAc–LiClO4 solid polymer electrolytes, J. Phys. Chem. Solids, 2007, 68(3), 407–412 CrossRef CAS
.
- S. Yu, R. D. Schmidt, R. Garcia-Mendez, E. Herbert, N. J. Dudney and J. B. Wolfenstine,
et al., Elastic Properties of the Solid Electrolyte Li7 La3 Zr2 O12 (LLZO), Chem. Mater., 2016, 28(1), 197–206 CrossRef CAS
.
- L. Othman, K. W. Chew and Z. Osman, Impedance spectroscopy studies of poly (methyl methacrylate)-lithium salts polymer electrolyte systems, Ionics, 2007, 13(5), 337–342 CrossRef CAS
.
- M. Petrowsky and R. Frech, Temperature Dependence of Ion Transport: The Compensated Arrhenius Equation, J. Phys. Chem. B, 2009, 113(17), 5996–6000 CrossRef CAS PubMed
.
- M. Ravi, Y. Pavani, S. Bhavani, A. K. Sharma and V. V. R. N. Rao, Investigations on Structural and Electrical Properties of KClO4 Complexed PVP Polymer Electrolyte Films, Int. J. Polym. Mater., 2012, 61(5), 309–322 CrossRef CAS
.
- R. Baskaran, S. Selvasekarapandian, N. Kuwata, J. Kawamura and T. Hattori, ac impedance, DSC and FT-IR investigations on (x)PVAc–(1−x)PVdF blends with LiClO4, Mater. Chem. Phys., 2006, 98(1), 55–61 CrossRef CAS
.
- J. Siva Kumar, A. R. Subrahmanyam, M. J. Reddy and U. V. S. Rao, Preparation and study of properties of polymer electrolyte system (PEO + NaClO3), Mater. Lett., 2006, 60(28), 3346–3349 CrossRef
.
- S. Sreepathirao, M. Jaipalreddy, K. Narasimhareddy and U. Subbarao, A new Na+ ion conducting polymer electrolyte based on (PEO + NaYF4) and its use as an electrochemical
cell, Solid State Ionics, 1994, 74(3–4), 225–228 CrossRef CAS
.
- F. Fan, Y. Wang, T. Hong, M. F. Heres, T. Saito and A. P. Sokolov, Ion Conduction in Polymerized Ionic Liquids with Different Pendant Groups, Macromolecules, 2015, 48(13), 4461–4470 CrossRef CAS
.
- C. Angell, Fast ion motion in glassy and amorphous materials, Solid State Ionics, 1983, 9–10, 3–16 CrossRef CAS
.
- C. A. Angell, Dynamic processes in ionic glasses, Chem. Rev., 1990, 90(3), 523–542 CrossRef CAS
.
- C. A. Angell, Mobile Ions in Amorphous Solids, Annu. Rev. Phys. Chem., 1992, 43(1), 693–717 CrossRef CAS
.
- W. M. Wang, Study on All Solid-State Composite Polymer Electrolyte, Adv. Mater. Res., 2012, 571, 13–16 CAS
.
- A. M. Stephan, Review on gel polymer electrolytes for lithium batteries, Eur. Polym. J., 2006, 42(1), 21–42 CrossRef
.
- E. Quartarone and P. Mustarelli, Electrolytes for solid-state lithium rechargeable batteries: recent advances and perspectives, Chem. Soc. Rev., 2011, 40(5), 2525 RSC
.
- D. P. Deshmukh, P. D. Shirbhate, S. S. Yawale and S. P. Yawale, Application of Vogel-Tamman-Fulcher (VTF) model to polythiophene composite thin films, J. Polym. Eng., 2012, 32(2), 103–110 CAS
.
- J. B. Goodenough, H. Y. P. Hong and J. A. Kafalas, Fast Na+-ion transport in skeleton structures, Mater. Res. Bull., 1976, 11(2), 203–220 CrossRef CAS
.
- W. Jia, Z. Li, Z. Wu, L. Wang, B. Wu and Y. Wang,
et al., Graphene oxide as a filler to improve the performance of PAN-LiClO4 flexible solid polymer electrolyte, Solid State Ionics, 2018, 315, 7–13 CrossRef CAS
.
- J. N. Chazalviel, Electrochemical aspects of the generation of ramified metallic electrodeposits, Phys. Rev. A, 1990, 42(12), 7355–7367 CrossRef CAS PubMed
.
- C. Monroe and J. Newman, Dendrite Growth in Lithium/Polymer Systems, J. Electrochem. Soc., 2003, 150(10), A1377 CrossRef CAS
.
- H. Jiang, Q. Zhang, Y. Zhang, L. Sui, G. Wu and K. Yuan,
et al., Li-Ion solvation in propylene carbonate electrolytes determined by molecular rotational measurements, Phys. Chem. Chem. Phys., 2019, 21(20), 10417–10422 RSC
.
- S. Han, Structure and dynamics in the lithium solvation shell of nonaqueous electrolytes, Sci. Rep., 2019, 9(1), 5555 CrossRef PubMed
.
- B. Y. Chang and S. M. Park, Electrochemical Impedance Spectroscopy, Annu. Rev. Anal. Chem., 2010, 3(1), 207–229 CrossRef CAS PubMed
.
- D. Sotta, J. Bernard and V. Sauvant-Moynot, Application of electrochemical impedance spectroscopy to the study of ionic transport in polymer-based electrolytes, Prog. Org. Coat., 2010, 69(2), 207–214 CrossRef CAS
.
- F. Henn, C. Durand, A. Cerepi, E. Brosse and J. C. Giuntini, DC conductivity, cationic exchange capacity, and specific surface area related to chemical composition of pore lining chlorites, J. Colloid Interface Sci., 2007, 311(2), 571–578 CrossRef CAS PubMed
.
- S. B. Aziz, Occurrence of electrical percolation threshold and observation of phase transition in chitosan(1−x):AgI x (0.05 ≤ x ≤ 0.2)-based ion-conducting solid polymer composites, Appl. Phys. A, 2016, 122(7), 706 CrossRef
.
- A. Nicolau, A. M. Nucci, E. M. A. Martini and D. Samios, Electrical impedance spectroscopy of epoxy systems II: Molar fraction variation, resistivity, capacitance and relaxation processes of 1,4-butanediol diglycidyl ether/succinic anhydride and triethylamine as initiator, Eur. Polym. J., 2007, 43(6), 2708–2717 CrossRef CAS
.
- M. Urquidi-Macdonald, S. Real and D. D. Macdonald, Applications of Kramers—Kronig transforms in the analysis of electrochemical impedance data—III. Stability and linearity, Electrochim. Acta, 1990, 35(10), 1559–1566 CrossRef CAS
.
- A. C. h. Lazanas and M. I. Prodromidis, Electrochemical Impedance Spectroscopy—A Tutorial, ACS Meas. Sci. Au, 2023, 3(3), 162–193 CrossRef CAS PubMed
.
-
M. A. Careem, I. S. M. Noor and A. K. Arof, Impedance Spectroscopy in Polymer Electrolyte Characterization, in Polymer Electrolytes, ed. T. Winie, A. K. Arof and S. Thomas, Wiley, 1st edn, 2020, pp. 23–64 [cited 2024 Dec 6] Available from: https://onlinelibrary.wiley.com/doi/10.1002/9783527805457.ch2 Search PubMed
.
- Z. Li, Y. Zhao and W. E. Tenhaeff, 5 V Stable Nitrile-Bearing Polymer Electrolyte with Aliphatic Segment as Internal Plasticizer, ACS Appl. Energy Mater., 2019 My 28, 2(5), 3264–3273 Search PubMed
.
- B. Boukamp, A Nonlinear Least Squares Fit procedure for analysis of immittance data of electrochemical systems, Solid State Ionics, 1986, 20(1), 31–44 CrossRef CAS
.
- S. Aziz, R. Abdullah, M. Rasheed and H. Ahmed, Role of Ion Dissociation on DC Conductivity and Silver Nanoparticle Formation in PVA:AgNt Based Polymer Electrolytes, Deep Insights to Ion Transport Mechanism, Polymers, 2017, 9(8), 338 CrossRef PubMed
.
- C. Y. Hsu, M. H. Kuo and P. L. Kuo, Preparation, characterization, and properties of poly(styrene-b-sulfonated isoprene)s membranes for proton exchange membrane fuel cells (PEMFCs), J. Membr. Sci., 2015, 484, 146–153 CrossRef CAS
.
- O. V. Yarmolenko, K. G. Khatmullina, G. Z. Tulibaeva, L. M. Bogdanova and A. F. Shestakov, Towards the mechanism of Li+ ion transfer in the net solid polymer electrolyte based on polyethylene glycol diacrylate–LiClO4, J. Solid State Electrochem., 2012, 16(10), 3371–3381 CrossRef CAS
.
- W. Zhang, Z. Tu, J. Qian, S. Choudhury, L. A. Archer and Y. Lu, Design Principles of Functional Polymer Separators for High–Energy, Metal–Based Batteries, Small, 2018, 14(11), 1703001 CrossRef PubMed
.
- D. A. MacInnes and J. A. Beattie, The Free Energy of Dilution and the Transference Numbers of Lithium Chloride Solutions, J. Am. Chem. Soc., 1920, 42(6), 1117–1128 CrossRef CAS
.
- S. Bublil, G. Peta, M. Fayena-Greenstein, H. Alon-Yehezkel, O. Raskin and Y. Elias,
et al., Electrochemical Methods of Transference Number Determination for Polymer Electrolyte Systems: A Comparative Study, J. Electrochem. Soc., 2022, 169(11), 110523 CrossRef CAS
.
- J. Evans, C. A. Vincent and P. G. Bruce, Electrochemical measurement of transference numbers in polymer electrolytes, Polymer, 1987, 28(13), 2324–2328 CrossRef CAS
.
- M. Siekierski, M. Bukat, M. Ciosek, M. Piszcz and M. Mroczkowska-Szerszeń, Transference Number Determination in Poor-Dissociated Low Dielectric Constant Lithium and Protonic Electrolytes, Polymers, 2021, 13(6), 895 CrossRef CAS PubMed
.
- S. N. F. Yusuf, S. Z. Yusof, M. Z. Kufian and L. P. Teo, Preparation and electrical characterization of polymer electrolytes: A review, Mater. Today: Proc., 2019, 17, 446–458 CAS
.
- L. Y. Lin and C. C. Chen, Accurate characterization of transference numbers in electrolyte systems, J. Power Sources, 2024, 603, 234236 CrossRef CAS
.
- P. G. Bruce and C. A. Vincent, Steady state current flow in solid binary electrolyte cells, J. Electroanal. Chem. Interfacial Electrochem., 1987, 225(1–2), 1–17 CrossRef CAS
.
- Z. Fang, Z. Hong, Y. Luo, Y. Liu, H. Wu and H. Tian,
et al., Systematic study and effective improvement of voltammetry for accurate electrochemical window measurement of solid electrolytes, Electrochim. Acta, 2022, 414, 140210 CAS
.
- F. Scholz, Voltammetric techniques of analysis: the essentials, ChemTexts, 2015, 1(4), 17 Search PubMed
.
-
S. Parhi, N. Dash, S. Praharaj and D. Rout, An overview of voltammetric techniques to the present era, in Electrochemical Sensors Based on Carbon Composite Materials, ed. J. G. Manjunatha, IOP Publishing, 2022, p. 1-1-1–24 [cited 2024 Dec 6]. Available from: https://iopscience.iop.org/book/edit/978-0-7503-5127-0/chapter/bk978-0-7503-5127-<?pdb_no 0ch1?>0ch1<?pdb END?> Search PubMed
.
- . Chapter 37_ Voltammetric Techniques.
- G. B. Appetecchi, P. Romagnoli and B. Scrosati, Composite
gel membranes: a new class of improved polymer electrolytes for lithium batteries, Electrochem. Commun., 2001, 3(6), 281–284 CAS
.
- J. Heinze, Cyclic Voltammetry—“Electrochemical Spectroscopy”. New Analytical Methods (25), Angew. Chem., Int. Ed. Engl., 1984, 23(11), 831–847 Search PubMed
.
- T. Yang, S. X. Zhang, Y. Gao, F. C. Ji and T. W. Liu, Multilayer Membranes Based on Sulfonated Poly(Ether Ether Ketone) and Poly(Vinyl Alcohol) for Direct Methanol Membrane Fuel Cells, Open Fuel Cells J., 2008, 1, 4–8 CAS
.
- G. X. Wang, L. Yang, J. Z. Wang, H. K. Liu and S. X. Dou, Enhancement of Ionic Conductivity of PEO Based Polymer Electrolyte by the Addition of Nanosize Ceramic Powders, J. Nanosci. Nanotechnol., 2005, 5(7), 1135–1140 CrossRef CAS PubMed
.
- K. Rokesh, S. Anandan and K. Jothivenkatachalam, Polymer Electrolytes in Dye Sensitized Solar Cells, Mater. Focus, 2015, 4(4), 262–271 CrossRef CAS
.
- M. S. Su'ait, M. Y. A. Rahman and A. Ahmad, Review on polymer electrolyte in dye-sensitized solar cells (DSSCs), Sol. Energy, 2015, 115, 452–470 CrossRef
.
- J. H. Kim, M. S. Kang, Y. J. Kim, J. Won, N. G. Park and Y. S. Kang, Dye-sensitized nanocrystalline solar cells based on composite polymer electrolytes containing fumed silica nanoparticles, Chem. Commun., 2004,(14), 1662 RSC
.
- M. S. Kang, J. H. Kim, Y. J. Kim, J. Won, N. G. Park and Y. S. Kang, Dye-sensitized solar cells based on composite solid polymer electrolytes, Chem. Commun., 2005, 7, 889 RSC
.
- F. I. Chowdhury, M. H. Buraidah, A. K. Arof, B. E. Mellander and I. M. Noor, Impact of tetrabutylammonium, iodide and triiodide ions conductivity in polyacrylonitrile based electrolyte on DSSC performance, Sol. Energy, 2020, 196, 379–388 CAS
.
- T. Önen, M. Ö. Karakuş, R. Coşkun and H. Çetin, Reaching stability at DSSCs with new type gel electrolytes, J. Photochem. Photobiol., A, 2019, 385, 112082 CrossRef
.
- Z. Lan, J. Wu, D. Wang, S. Hao, J. Lin and Y. Huang, Quasi-solid state dye-sensitized solar cells based on gel polymer electrolyte with poly(acrylonitrile-co-styrene)/NaI + I2, Sol. Energy, 2006, 80(11), 1483–1488 CrossRef CAS
.
- H. Yang, M. Huang, J. Wu, Z. Lan, S. Hao and J. Lin, The polymer gel electrolyte based on poly(methyl methacrylate) and its application in quasi-solid-state dye-sensitized solar cells, Mater. Chem. Phys., 2008, 110(1), 38–42 CAS
.
- R. E. Rosli, A. B. Sulong, W. R. W. Daud, M. A. Zulkifley, T. Husaini and M. I. Rosli,
et al., A review of high-temperature proton exchange membrane fuel cell (HT-PEMFC) system, Int. J. Hydrogen Energy, 2017, 42(14), 9293–9314 CAS
.
- G. Li, W. Kujawski and E. Rynkowska, Advancements in proton exchange membranes for high-performance high-temperature proton exchange membrane fuel cells (HT-PEMFC), Rev. Chem. Eng., 2022, 38(3), 327–346 CAS
.
- T. Myles, L. Bonville and R. Maric, Catalyst, Membrane, Free Electrolyte Challenges, and Pathways to Resolutions in High Temperature Polymer Electrolyte Membrane Fuel Cells, Catalysts, 2017, 7(1), 16 CrossRef
.
- Y. Wang, B. Seo, B. Wang, N. Zamel, K. Jiao and X. C. Adroher, Fundamentals, materials, and machine learning of polymer electrolyte membrane fuel cell technology, Energy AI, 2020, 1, 100014 Search PubMed
.
- Y. Chang, Y. Qin, Y. Yin, J. Zhang and X. Li, Humidification strategy for polymer electrolyte membrane fuel cells – A review, Appl. Energy, 2018, 230, 643–662 CrossRef CAS
.
- A. Ibrahim, O. Hossain, J. Chaggar, R. Steinberger-Wilckens and A. El-Kharouf, GO-nafion composite membrane development for enabling intermediate temperature operation of polymer electrolyte fuel cell, Int. J. Hydrogen Energy, 2020, 45(8), 5526–5534 CrossRef CAS
.
- Z. Jie, T. Haolin and P. Mu, Fabrication and characterization of self-assembled Nafion–SiO2−ePTFE composite membrane of PEM fuel cell, J. Membr. Sci., 2008, 312(1–2), 41–47 CrossRef
.
- X. Sun, S. Simonsen, T. Norby and A. Chatzitakis, Composite Membranes for High Temperature PEM Fuel Cells and Electrolysers: A Critical Review, Membranes, 2019, 9(7), 83 CrossRef CAS PubMed
.
- R. Haider, Y. Wen, Z. F. Ma, D. P. Wilkinson, L. Zhang and X. Yuan,
et al., High temperature proton exchange membrane fuel cells: progress in advanced materials and key technologies, Chem. Soc. Rev., 2021, 50(2), 1138–1187 RSC
.
- S. S. Araya, F. Zhou, V. Liso, S. L. Sahlin, J. R. Vang and S. Thomas,
et al., A comprehensive review of PBI-based high temperature PEM fuel cells, Int. J. Hydrogen Energy, 2016, 41(46), 21310–21344 CrossRef CAS
.
- T. Yang, S. X. Zhang, Y. Gao, F. C. Ji and T. W. Liu, Multilayer Membranes Based on Sulfonated Poly(Ether Ether Ketone) Poly(Vinyl Alcohol) for Direct Methanol Membrane Fuel Cells, Open Fuel Cells J., 2009, 1(1), 4–8 CrossRef
.
- A. Matsuda, H. Honjo, K. Hirata, M. Tatsumisago and T. Minami, Electric double-layer capacitor using composites composed of phosphoric acid-doped silica gel and styrene–ethylene–butylene–styrene elastomer as a solid electrolyte, J. Power Sources, 1999, 77(1), 12–16 CrossRef CAS
.
- S. Nohara, H. Wada, N. Furukawa, H. Inoue, M. Morita and C. Iwakura, Electrochemical characterization of new electric double layer capacitor with polymer hydrogel electrolyte, Electrochim. Acta, 2003, 48(6), 749–753 CAS
.
- N. E. A. Shuhaimi, L. P. Teo, H. J. Woo, S. R. Majid and A. K. Arof, Electrical double-layer capacitors with plasticized polymer electrolyte based on methyl cellulose, Polym. Bull., 2012, 69(7), 807–826 CAS
.
- A. Lewandowski, Supercapacitor based on activated carbon and polyethylene oxide–KOH–H2O polymer electrolyte, Electrochim. Acta, 2001, 46(18), 2777–2780 CAS
.
-
I. Rodi, F. Saaid and T. Winie, PEMA - LiCF3SO3 polymer electrolytes: Assessment of conductivity and transport properties, in Astana, Kazakhstan, 2017, p. 060003 [cited 2024 Dec 6]. Available from: https://pubs.aip.org/aip/acp/article/781756 Search PubMed
.
- N. Anuar, R. Subban and N. Mohamed, Properties of PEMA-NH4CF3SO3 Added to BMATSFI Ionic Liquid, Materials, 2012, 5(12), 2609–2620 CAS
.
- P. F. R. Ortega, J. P. C. Trigueiro, G. G. Silva and R. L. Lavall, Improving supercapacitor capacitance by using a novel gel nanocomposite polymer electrolyte based on nanostructured SiO2, PVDF and imidazolium ionic liquid, Electrochim. Acta, 2016, 188, 809–817 CrossRef CAS
.
- D. Song, C. Xu, Y. Chen, J. He, Y. Zhao and P. Li,
et al., Enhanced thermal and electrochemical properties of PVDF-HFP/PMMA polymer electrolyte by TiO2 nanoparticles, Solid State Ionics, 2015, 282, 31–36 CrossRef CAS
.
- X. Zheng, Novel “Rubber” electrolyte will be applied to long-lasting, safer future EV batteries, Adv. Sens. Energy Mater., 2022, 1(1), 100002 CrossRef
.
- J. C. Barbosa, R. Gonçalves, C. M. Costa and S. Lanceros-Méndez, Toward Sustainable Solid Polymer Electrolytes for Lithium-Ion Batteries, ACS Omega, 2022, 7(17), 14457–14464 CrossRef CAS PubMed
.
- X. Zhou, C. Li, R. Bhandary, Z. Katcharava, F. Du and R. Androsch,
et al., Catalyst-Free, Mechanically Robust, and Ion-Conductive Vitrimers for Self-Healing Ionogel Electrolytes, ACS Appl. Eng. Mater., 2023, 1(8), 1997–2003 CrossRef CAS
.
|
This journal is © The Royal Society of Chemistry 2025 |
Click here to see how this site uses Cookies. View our privacy policy here.