DOI:
10.1039/D4FO04461D
(Paper)
Food Funct., 2025,
16, 268-281
Obesity phenotype and gut microbiota alterations are not associated with anxiety-like behaviour in high-fat diet-fed mice†
Received
14th September 2024
, Accepted 20th November 2024
First published on 10th December 2024
Abstract
Anxiety is a common co-morbidity with obesity and metabolic disease, and can lead to a significant impact on quality of life. The vast differences in the gut microbiota between obese and control individuals provide a potential avenue for therapeutic intervention. A high-fat diet (HFD) in rodent models have been shown to induce anxiety-like behaviour and has been tested through an array of distinct behavioural tests such as the elevated plus maze test, light–dark test and open field test. Despite differences in testing and assessment parameters, the behavioural outcomes have previously yielded similar results. Recent evidence suggests that HFD has an anxiolytic effect on mice, complicating the model. Here, we aimed to confirm whether HFD-fed mice are more susceptible to presenting anxiety-like behaviours. Our findings showed no significant differences in behaviour, plasma corticosterone and inflammation markers between HFD and control diet (CTD) mice, despite considerable differences in adiposity and faecal microbial communities. Additionally, daily oral gavage is one of the most common methods for testing bacterial probiotics in rodent models, but this handling could potentially also cause stress to the mice. Thus, we investigated if daily oral gavage could mask differences in HFD and CTD mice. We found no significant differences in weight, fat mass or anxiety-like behaviour in CTD-fed mice with or without daily oral gavage.
1 Introduction
Globally, the prevalence of anxiety disorders has risen significantly in recent years;1–3 while it is generally diagnosed by clinicians according to the Diagnostic and Statistical Manual of Mental Disorders, Fifth Edition (DSM-5),4 it is described in animals as the behavioural response induced by unknown threats or internal conflict and examined in a variety of tests that incite a confrontation of these situations.5,6 Anxiety-related behaviours in mice have been assessed using a variety of apparatus, including the open field, light–dark box and elevated plus maze. Anxiety and/or depression are common co-morbidities of obesity and can significantly affect the quality of daily life.7,8
Obesity is often associated with profound changes in the gut microbiota and has been associated with modified intestinal permeability.9,10 In metabolic disorders, increased circulation of pro-inflammatory cytokines and other inflammation-induced processes are exacerbated, which has been linked to the development of anxiety and depression.11 Differences in gastrointestinal microbial communities between control (CTD) and high-fat diet (HFD)-fed mice and the general ease of modulating this population via diet/probiotics/dietary fibres have created an opportunistic target for potential treatment (reviewed in ref. 12). Probiotics like Lactobacillus rhamnosus have been shown to alter GABA receptor expression in the brain and reduce anxiety-like behaviour in mice through key interactions via the vagus nerve, supporting the possibility of targeting treatments towards the bi-directional gut–brain–microbiota axis.13
To develop potential microbe based therapeutics, we have to establish a key phenotype of anxiety-related behaviour associated with HFD in mice to target and rescue. In the literature, HFD-induced obesity rodent models have been observed to have an anxiety-like phenotype, typically spending more time in the dark arena of the light–dark test,14 exhibiting reduced locomotion15,16 and increased time spent close to the walls compared to the centre of the arena during the open field test,17–19 as well as spending less time in the open arms of the elevated plus maze.16,17,19,20 Additionally, HFD-fed mice have shown increased depressive behaviours, as assessed by the time spent immobile using the Porsolt forced swim test,21–23 increased compulsive behaviours in the marble burying test, and additional memory deficits in the novel object recognition and object location memory tests.16,20,24 Recently, Huang et al. (2024) reported that mice on HFD for four weeks exhibited anxiolytic effects, with increased time spent in the open arms of the elevated plus maze and increased time in the light arena of the light–dark test.25 Their findings greatly contrast with the historical depiction of the anxiety phenotype associated with HFD-fed mice, highlighting the importance of the HFD duration. Ultimately, a conclusive, well-defined anxiety phenotype with HFD remains contentious. Further research is required to better define this key element in the study of the relationship between diet and anxiety.
Thus, this study aimed to determine whether HFD mice are more susceptible to experiencing stress/anxiety and how this may manifest in their behaviour. Additionally, since the most common method of administering therapeutic bacterial probiotics is oral gavage, we evaluated the effect of behaviour in response to daily gavage.
2 Materials and methods
2.1 Ethics
Mouse experiments were approved by the Ethical Committee for Animal Care of the Health Sector of the Université Catholique de Louvain (UCLouvain), headed by Prof. J-P Dehoux, under number 2022/UCL/MD/41. The experiments were performed in accordance with the guidelines of the Local Ethics Committee and in compliance with the Belgian Law of 29 May 2013 regarding the protection of laboratory animals (agreement number LA1230314).
2.2 Animals
7-week-old male C57BL6/JRj SOPF mice (n = 60) were obtained from Janvier laboratories (Saint-Berthevin, France). Animals were group-housed (3 animals per cage) under standard conditions (room temperature 22 ± 2 °C; 12-hour light–dark cycle) with ad libitum access to irradiated food and autoclaved Milli-Q water. Cage enrichment included a gummy gnawing bone (Bio-Serv; Flemington, NJ, USA), a red mouse house (Tecniplast; Provincia di Varese, Italy), shreded paper towel and corn cob-based bedding material (Rehofix mk1500; Technilab; Mésanger, France). All animals were acclimatised in our SOPF facility for one week prior to starting experimentation.
For the first experiment (n = 24, male), mice were randomly divided into two groups and were fed either a control low-fat diet (CTD) (AIN-93M mature rodent diet, D10012Mi, Research Diets; New Brunswick, NJ, USA) or a high-fat diet (HFD) (Rodent diet with 60 kcal% fat and 20% carbohydrate, D12492i, Research Diets) for a total period of eight weeks. Comprehensive dietary details are provided in ESI Table 1.† All mice in this first experiment had a control vehicle solution containing 15% trehalose administered daily via oral gavage.
In the second experiment, all mice (n = 36, male) were fed the CTD. These 36 mice were randomly allocated into one of the two gavage treatment groups: (1) no gavage intervention and (2) control vehicle gavage solution containing 15% trehalose; these gavage interventions were imposed for a total of eight weeks.
Body composition analysis was performed using a 7.5 MHz time domain-nuclear magnetic resonance machine (LF50 Minispec; Bruker, Rheinstetten, Germany). For the first experiment, body composition was measured each week for nine weeks, and in the second experiment, this was only carried out before starting gavage intervention (week one), before and after behavioural testing (weeks six and eight post gavage intervention). At the end of each experiment, mice were anesthetised with isoflurane (Forene; Abbott, Queenborough, Kent, England). After euthanasia, brain tissue (hippocampus, hypothalamus, and cortex) and adipose tissue (subcutaneous, epidydimal, and visceral) were promptly collected, snap-frozen with liquid nitrogen and stored at −80 °C until further processing.
2.3 Plasma corticosterone ELISA
At three time points: before experimental diet/gavage intervention (week one), pre-behavioural battery testing and post-behavioural battery testing (weeks six and eight post dietary/gavage intervention) for both experiments, ∼75 μl of tail vein blood was collected using haematocrit capillary tubes. These samples were promptly processed, and the plasma was stored at −80 °C. Plasma corticosterone levels were measured using a corticosterone ELISA kit (Enzo Life Sciences; Farmingdale, NY, USA) with a detection range from 32 to 20
000 pg mL−1. The assay was carried out according to the manufacturer's instructions, with an initial 1
:
30 dilution according to the small volume protocol for serum/plasma. Samples were run in duplicate, and the absorbance was measured at 405 nm (with 570 nm correction) using a SpectraMax i3x microplate reader (Molecular Devices; San Jose, CA, USA).
2.4 DNA / RNA extraction and analyses
Freshly defecated mouse faecal samples were collected at two time points (before and after behavioural battery testing – week six and eight respectively post dietary/gavage intervention) and promptly stored in sterile tubes at −80 °C until further processing. DNA extraction blanks using sterile water (n = 4) and the final eluent solution from the DNA extraction kits (n = 4) were used as negative control samples and were treated in parallel with the faecal samples throughout the sequencing preparation and were also sequenced to identify potential contamination.
DNA was extracted from 30–40 mg of faeces using a QIAmp Fast DNA Stool Mini Kit (Qiagen; Hilden, Germany) according to the manufacturer's instructions with the following modifications. First, we carried out a pre-wash step with cold PBS and centrifugation at 20
000 xg before starting the kit procedure. In addition, we included glass bead (0.25–0.50 mm) beating homogenisation; 10 μL RNase (100 mg mL−1, Qiagen; Hilden, Germany) and 40 μL proteinase K (20 mg mL−1, Qiagen; Hilden, Germany) were also added during the extraction process.
The sequencing libraries were prepared according to the Illumina 16S metagenomic sequencing library preparation protocol. Briefly, the V3–V4 regions of the 16S rRNA gene in the faecal DNA were amplified using a two-stage PCR protocol.26 Final libraries were quantified and equimolarly pooled to a final concentration of 2 nM and sequenced on an Illumina NextSeq platform using a P1 flow cell (600 cycles) with a paired-end 2 × 300 bp (PE 2 × 300) sequencing protocol. The sequencing data were analysed using the DADA2 pipeline (v1.22.0
27), and sequences were filtered for quality, dereplicated and merged, with singletons and chimeras removed to generate amplicon sequence variants (ASVs). The SILVA database (v138.1
28) was used for taxonomic assignment of ASVs. Each ASV represented at least 0.00001% of total sequences and had at least 5 reads across the dataset. For alpha diversity analyses, the sequencing data were rarefied prior to analysis (first experiment: 59
107 sequence reads, second experiment: 121
639 sequence reads).
RNA was extracted from brain tissue (collected at sacrifice – week nine) using a Trizol Isolation Reagent (Roche; Basil, Switzerland) with a single 5 mm stainless steel bead and a TissueLyser II machine (Qiagen) at 30 Hz for 2 minutes. Extracted RNA was converted into cDNA using a GoScript Reverse Transcriptase kit (Promega; WI, USA). A QuantStudio 3 RT PCR system (Thermo Fisher; Waltham, MA, USA) was used to carry out Real-Time PCR with SYBR Green (Promega) with the housekeeper gene RPL19 and genes of interest (primers and sequences in ESI Table 2†). Samples were processed in duplicate under the following thermocycling conditions: 95 °C for 2 minutes; 40 cycles of 95 °C for 30 seconds, 60 °C for 30 seconds, 72 °C for 30 seconds; 95 °C for 1 second, 60 °C for 20 seconds and finally 95 °C for 1 second. Results were analysed using the 2−ΔΔCT method.
2.5 Behavioural tests
All mice were habituated in their home cage for at least one hour inside the behaviour room to acclimatise before commencing behavioural testing. All materials were cleaned with 70% ethanol. Behavioural tests were carried out in the order of impact (lowest to the highest): open field test, marble bury test, light–dark test, elevated plus maze, and forced swim test, starting from six weeks post experimental gavage/diet. To reduce stress from the behavioural test battery, a 48-hour rest period was established between each behavioural test, and all tests were completed before the end of week eight on the diet/gavage intervention. A GigE monochrome Basler camera and the Ethovision XT software (v17.0.1630, Noldus Information Technology; Wageningen, Netherlands) were used to capture and assess mouse behaviour. The experimenter was blinded to the experimental group during the tests and was only unblinded after the completion of the analysis.
2.5.1 Open field test.
Mice were placed in the centre of an opaque white Plexiglas chamber (50 cm × 50 cm × 50 cm) and their activity was recorded for 10 minutes. To assess thigmotaxis, the open field arena was divided into a 4 by 4 grid with time spent in the central zones measured in the central four grid squares compared to the peripheral grid squares.
2.5.2 Marble bury test.
Clean cages (44.5 cm × 25.5 cm × 14.0 cm) were filled with clean irradiated corn cob bedding (Rehofix mk1500; Technilab; Mésanger, France) to a height of 4.5 cm. Mice were placed in the arena for 20 minutes to acclimatise, after which the bedding was levelled and a grid was used to place 20 equidistant black glass marbles (1.4 cm diameter). Mice were returned to the experimental arena with their behaviour recorded for 30 minutes; after this time period, the number of marbles buried by more than two-thirds were assessed.
2.5.3 Light–dark test.
The arena was divided into two sections: the light arena (40 cm × 20 cm; 400 lux) and the dark arena (20 cm × 20 cm), which were separated by a door. To assess mouse behaviour inside the dark arena, infrared lights were used and captured with an infrared camera filter. Three seconds after placing the mouse inside the dark arena, the door to the light arena was opened, allowing the mouse to freely explore both arenas for a 10-minute period.
2.5.4 Elevated plus maze.
A plus maze (77 cm × 6 cm × 14 cm), elevated 55 cm from the floor, was used with two sets of dark walls and two open arms. Mice were placed in the centre of the arena facing the open arm and were free to explore all four arms of the maze for 5 minutes.
2.5.5 Forced swim test.
Clear Plexiglas cylinders (13 cm × 24 cm) were filled with room temperature autoclaved Milli-Q water to a height of 13 cm. Mice were gently placed inside the water filled cylinder, and their activity was recorded for 6 minutes. After testing, the mice were placed in a cage with a heating pad and thoroughly dried with clean towels. Once dry, they were returned to their original home cage.
2.5.6 Behavioural testing and statistical analysis.
All behavioural tests were recorded with a GigE monochrome Basler camera lens and analysed using Noldus EthoVision XT (v17
29). Statistical analysis of the behavioural outcomes was performed using R (version 4.1.1; RCoreTeam, 2019) and GraphPad Prism (version 10; GraphPad Software). If the data satisfied the assumptions for an ANOVA, a two-way ANOVA with Šídák's multiple comparisons test was carried out; otherwise, t-test analyses were used.
3 Results
3.1. High-fat diet-fed mice do not exhibit more anxiety-like behaviours than control diet-fed mice
HFD-fed male mice treated with vehicle oral gavage gained significantly more body weight and fat mass than male mice on a CTD (Fig. 1).
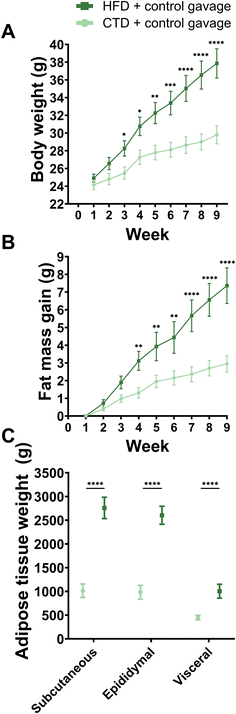 |
| Fig. 1 Body weight gain of male C57BL/JRj mice (A) and fat mass gain determined by 7.5 MHz time domain-nuclear magnetic resonance (B), assessed weekly for nine weeks. Weights of subcutaneous, epididymal and visceral adipose tissue collected at sacrifice (C). All data are shown as mean ± SEM. For each experimental group, n = 12. Comparisons assessed using two-way ANOVA with fdr p-value adjustment. * p < 0.05; ** p < 0.01; *** p < 0.001; and **** p < 0.0001. | |
The anxiety-related behavioural test battery was carried out after six weeks of experimental diet and gavage treatment. HFD and CTD mice showed significantly different body compositions and weights (Fig. 1), but there was no significant difference in phenotypical behaviour between the two groups in the open field, marble bury, light–dark, elevated plus maze, and forced swim tests (Fig. 2). The only significant differences between these experimental groups were detected in stretch attend postures in the open field test (p-value = <0.0001) and the latency to enter the light arena during the light–dark test (p-value = 0.0345) (Fig. 2G). All other parameters in each behavioural test were not significantly different between HFD-fed and CTD-fed mice with vehicle oral gavage.
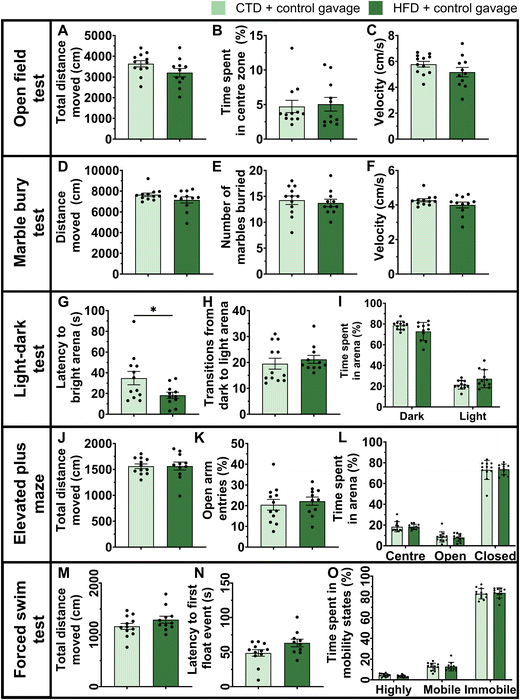 |
| Fig. 2 Main parameters from the behavioural battery testing: open field test, marble bury test, light–dark test, elevated plus maze and the forced swim test carried out between six and eight weeks post dietary/gavage intervention. Each point represents one mouse, and the top of the bars displays the mean with SEM error bars. For each experimental group, n = 12. t-test statistical analyses (A–H, J–K, M–N) and two-way ANOVA with Šidák's multiple comparisons test (I, L, O) were carried out. * p < 0.05; ** p < 0.01; *** p < 0.001; and **** p < 0.0001. | |
To further explore the reason why the HFD mice did not develop distinct phenotypical behaviour, we explored markers of inflammation in the brain. HFD has been associated with the development of brain inflammation,30 depression31 and anxiety.32–34 Therefore, we measured the expression of several genes in the hypothalamus, hippocampus and cortex, related to inflammation (Brain-derived neurotrophic factor (BDNF), Interleukin 1-beta (IL-1b), Interleukin 6 (IL-6), Monocyte chemoattractant protein 1 (MCP-1), Toll-like receptor 4 (TLR4), Tumor necrosis factor alpha (TNFα)) and blood–brain barrier (Claudin 1 (CL-1), Claudin 2 (CL-2), Occludin (OCC), Zonula occludens 1 (ZO-1)). Surprisingly, there were no significant differences between the two experimental groups (Fig. 3).
 |
| Fig. 3 Relative expression of genes related to inflammation detected in the cortex (A), hippocampus (C), and hypothalamus (E); genes related to the blood–brain-barrier in the cortex (B), hippocampus (D) and hypothalamus (F); determined by qPCR using the 2−ΔΔCT method; data shown as mean ± SEM. Brain samples were collected at sacrifice – eight weeks post dietary/gavage intervention. Each colour of the bars represents each experimental group. | |
Another important factor contributing to specific behavioural changes upon HFD feeding is the gut microbiota. Overall, the greatest differences were found in the alpha and beta diversity of the faecal gut microbiota (Fig. 4). HFD-fed mice had lower numbers of observed species (number of ASVs) and a decreased Chao1 index compared to their CTD counterparts. Interestingly, the beta diversity observed in CTD-fed mice faecal microbiota shifted towards those of the HFD-fed mice after the behavioural battery tests, whereas the HFD-fed mice had a similar Bray–Curtis dissimilarity on the nMDS plot before and after the behavioural tests. In addition, 16S rRNA gene sequencing revealed a significant decrease in the relative abundance of the phylum Bacteroidota over time in the HFD-fed group (Fig. 4 and ESI Table 3, ESI Fig. 2A†) (average ± SEM: before behavioural testing 44.21% ± 2.36; after behavioural testing 41.18% ± 3.90; p-value = 0.0002). Significant ASV differences in HFD- and CTD-fed mice at six weeks post dietary intervention included taxa from the family Muribaculaceae, Lachnospiraceae, Ruminococcaceae and Lactobacillaceae (ESI Tables 4 and 5†).
 |
| Fig. 4 Relative abundance taxonomic bar plots of faecal bacteria phyla (A) and the top 20 genera (B); each bar represents one mouse. Chao1 index alpha diversity box plots based on rarefied ASV tables (C). Panels separate groups by time point: before and after behavioural battery testing (six to eight weeks of dietary/gavage intervention respectively). Beta diversity nMDS plot of Bray–Curtis dissimilarity (D). Each point represents one sample with 95% confidence interval ellipses. Circle symbols represent before behavioural testing and triangle symbols represent after behavioural testing. Each colour represents each experimental group (n = 12). Stress represents the degree of distortion in the data when constrained to two-dimensional space. | |
Firmicutes and Verrucomicrobiota showed significant differences between the CTD-fed and HFD-fed groups before and after behavioural testing. In the phyla Firmicutes, the differences between the CTD-fed and HFD-fed groups were mostly evident before behavioural testing (CTD-fed group = 29.58% ± 2.06 and HFD-fed group = 35.76% ± 1.99; p-value = 0.032) and after behavioural testing (CTD-fed group = 29.39% ± 2.01 and HFD-fed group = 40.46% ± 2.61; p-value = <0.0001). In the case of Verrucomicrobiota, significant differences were also observed before (CTD-fed group = 11.33% ± 1.05 and HFD-fed group = 5.35% ± 1.34; p-value = 0.042) and after (CTD-fed group = 11.60% ± 1.30 and HFD-fed group = 4.09% ± 1.23; p-value = <0.0001).
Another possible difference is the effect of diet and/or the gavage on the secretion of corticosterone. We measured plasma corticosterone at three time points: (1) one-week post acclimatisation to our animal facility and before starting the experimental diet (8 weeks old), (2) before the behavioural battery tests (14 weeks old), and (3) after behavioural battery tests (16 weeks old). Plasma corticosterone levels were not significantly different across any of the three time points (Fig. 5).
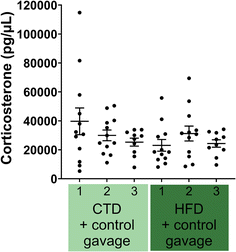 |
| Fig. 5 Plasma corticosterone levels determined by ELISA in both CTD (n = 12) and HFD (n = 12) experimental groups. Each mouse is represented by one point; plasma was analysed at three time points: before starting the experimental diet/gavage intervention – week one (1), before behavioural battery testing (2) and after behavioural battery testing (3) – week six and eight post diet/gavage intervention, respectively. | |
Given the lack of difference between CTD and HFD mice, we hypothesised that the daily oral gavage of the mice could be a significant confounding factor, by increasing stress levels in both groups. Therefore, we designed a second experiment devoted to determining whether oral gavage could influence stress levels in mice.
3.2. Oral gavage treatment does not influence stress in male mice
In the second experiment, both experimental groups were fed a control diet and allocated into either the control vehicle gavage or the no-gavage intervention group.
First, we observed that oral gavage had no effect on weight gain or fat mass gain throughout the experiment (Fig. 6). Similarly, results from the anxiety-related behavioural tests ascertained that mice receiving no-gavage treatment had the same phenotype as mice receiving a daily control vehicle oral gavage (Fig. 7). There were no differences in the qPCR analyses of selected inflammation and blood–brain barrier genes in the brain regions tested (the hypothalamus, hippocampus and cortex; Fig. 8).
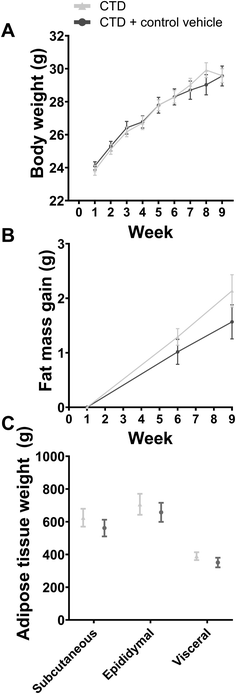 |
| Fig. 6 Body weight gain of male C56BL6/JRj mice assessed weekly for nine weeks (A) and fat mass gain determined by 7.5 MHz time domain-nuclear magnetic resonance (B) carried out before gavage intervention, before and after behavioural testing (week one, six and eight, respectively). Weight of subcutaneous, epididymal and visceral adipose tissues collected at sacrifice (C). All data are shown as mean ± SEM. For each experimental group, n = 12. Comparisons assessed using two-way ANOVA with fdr p-value adjustment. * p < 0.05; ** p < 0.01; *** p < 0.001; and **** p < 0.0001. | |
 |
| Fig. 7 Main parameters from the behavioural battery testing: open field test, marble bury test, light–dark test, elevated plus maze and the forced swim test carried out between six and eight weeks post gavage intervention. Each point represents one mouse; the top of the bars displays the mean with SEM error bars. For each experimental group, n = 12. t-test statistical analyses (A–H, J–K, M–N) and two-way ANOVA with Šidák's multiple comparisons test (I, L, O) were carried out. * p < 0.05; ** p < 0.01; *** p < 0.001; and **** p < 0.0001. | |
 |
| Fig. 8 Relative expression of genes related to inflammation detected in the cortex (A), hippocampus (C), and hypothalamus (E); genes related to the blood–brain barrier in the cortex (B), hippocampus (D) and hypothalamus (F); determined by qPCR using the 2−ΔΔCT method, data shown as mean ± SEM. Brain samples were collected at sacrifice – eight weeks post gavage intervention. Each colour of the bars represents each experimental group. | |
Interestingly, we observed no significant difference in the alpha diversity metrics tested. The beta diversity of both the control vehicle gavage group and the no-gavage group overlapped significantly with the two groups, as well as at the two time points (before and after behavioural battery testing), suggesting that the beta diversities of bacterial microbiota of these groups were very similar (R2 = 0.976). Bacteroidota was the phylum that showed a significant difference in abundance between the two groups (Fig. 9 and ESI Table 3 and ESI Fig. 2B†). Before behavioural testing, the no-gavage group had higher average relative abundance (45.29% ± 1.72) compared to the control gavage group (51.58% ± 2.07; p-value = 0.0076). However, after behavioural testing, no significant difference was found.
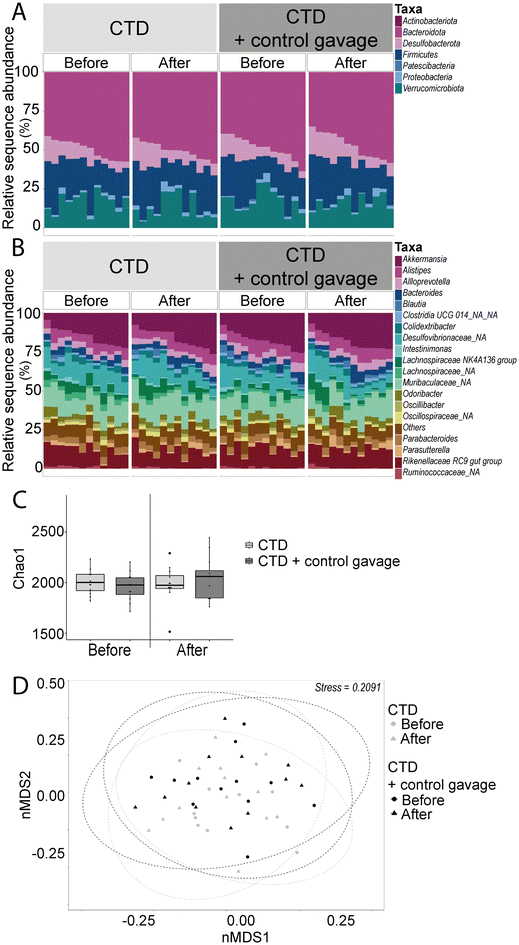 |
| Fig. 9 Relative abundance taxonomic bar plots of faecal bacteria phyla (A) and the top 20 genera (B); each bar represents one mouse. Chao1 index alpha diversity box plots based on rarefied ASV tables (C). Panels separate groups by time point: before and after behavioural battery testing (six to eight weeks of gavage intervention respectively). Beta diversity nMDS plot of Bray–Curtis dissimilarity (D). Each point represents one sample with 95% confidence interval ellipses. Circle symbols represent before behavioural testing and triangle symbols represent after behavioural testing. Each colour represents each experimental group (n = 12). Stress represents the degree of distortion in the data when constrained to two-dimensional space. | |
No differences in plasma levels of corticosterone were found at three time points throughout the experimental period, supporting the lack of phenotypical difference in behaviour (Fig. 10).
 |
| Fig. 10 Plasma corticosterone levels determined by ELISA in both CTD (n = 12) and CTD + control gavage (n = 12) experimental groups. Each mouse is represented by one point; plasma was analysed at three time points: before starting an experimental diet/gavage intervention – week one (1), before behavioural battery testing (2) and after behavioural battery testing (3) – week six and eight post gavage intervention, respectively. | |
4 Discussion
Based on the literature, we expected that HFD mice would spend less time in open arms (elevated plus maze), display reduced total activity (open field test) and increased time spent immobile (forced swim test), with corresponding increases in corticosterone levels.18,22 However, we did not observe a strong phenotype, which has previously been associated with HFD feeding, across almost all parameters in behavioural tests for assessing anxiety in mice. After 6 weeks on HFD, male C57BL6/JRj SOPF mice did not display any major significant difference in anxiety-like behaviour parameters compared to the mice on the control diet, except for latency to enter the light arena in the light–dark test. Although the majority of papers suggest striking differences between HFD and CTD-fed mice,21,22,35 some studies report no significant differences in HFD and anxiety behaviour in mice.14 HFD has also been shown to lengthen the active phase (free-running period) and time spent feeding (during the light phase) while decreasing the amplitude of the corticosterone rhythm;35 yet, we did not observe this when comparing plasma corticosterone before and after the behavioural testing. Due to the heterogeneity of anxiety and the complex translation of mouse to human behaviour associated with anxiety, it can be difficult to encompass all aspects of anxiety in an animal model. However, the success of attenuating anxiety behaviours displayed by mice through the use of anxiolytic drugs provides evidence that using these animal models can provide a mechanism for understanding anxiety and exploring potential therapies.36,37
Since both groups in the first experiment received daily oral gavage treatment, it is possible that the stress from oral gavage contributed to the lack of differences in the behavioural tests. Therefore, in the second experiment, we included a no-gavage intervention experimental group with minimal handling to determine if reducing these stressors associated with oral gavage and experimenter handling could affect the outcomes. Again, after 6 weeks, the two groups did not exhibit distinct phenotypes in the behavioural battery series.
While this 6-week time period of dietary treatment has been utilised for many obesogenic diet studies, perhaps extending the time spent on an HFD would have yielded different results in behaviour. Anxiety-related behavioural testing carried out by Mizunoya et al. (2013) demonstrated that mice on different sources and proportions of dietary fat exhibit distinct behavioural changes, with the greatest difference found on the elevated plus maze, successfully identifying differences despite a short three-day time frame of experimental diet; however, differences were not found in the Porsolt forced swim test.38 According to our results in the first experiment, by extending the experimental dietary period to a total of 13 weeks of dietary intervention, we observed no deviation or significant effects of the anxiety-like behaviours between the 6- and 13-week dietary intervention periods. Additionally, the mice used in these experiments were 14 weeks of age during behavioural testing; it is possible that younger mice may have shown different effects of the HFD. The diet used in this study contained soybean oil in both diets, but our HFD also contains lard, resulting in a 60% kcal fat diet. One of our major findings is that oral gavage does not significantly change the phenotype of anxiety-related behaviour in male mice and that other parameters such as plasma corticosterone, faecal microbiota and expression of various genes involved in inflammation and blood–brain barrier integrity also remain unaffected. This suggests that daily oral gavage does not cause significant effects on stress and anxiety in treated mice.
Depression has been attributed to increased levels of pro-inflammatory factors such as IL-6, IL-1b and TNFα. There have been reports on developing depression-like behaviours as a result of chronic inflammation.39 Female mice tend to display significant differences in depression-like behaviour compared to male mice and this should also be considered in anxiety-related behaviours; thus, we have continued this project with female mice (data to follow). The production of the neurotransmitter serotonin can be influenced by a variety of inflammatory factors that can ultimately reduce the levels of serotonin and subsequently affect the metabolism of tryptophan.40 High dietary cholesterol has been shown to have a significant effect on depression and anxiety behaviour in mice, with an associated increase in the expression of inflammatory factors in plasma serum and in the hippocampus of mice.41 TNFα has also been shown to decrease the tight junction protein expression, thereby increasing the permeability of the blood–brain barrier,42 but we did not observe this trend in our study. The hypothesis that the gastrointestinal microbiota can induce increased neuroinflammation and circulating pro-inflammatory factors, and that the microbes from HFD-fed mice can cause anxiety-like behaviour without the obese phenotype, raises an intriguing possibility for mitigating anxiety behaviour using the gut microbes and/or their metabolites.17
Discrepancies between an HFD and the phenotype of anxiety-like behaviours were observed in a recent study, which reported completely opposite results to the general literature and our results, thus suggesting that the anxiety-like phenotype in HFD mice may be more complex than previously thought.25 Potential confounding factors may contribute to this disparity, such as the age of the mice when they start the HFD, the sources of dietary fat used, and the age at which the behavioural tests are conducted. Moreover, mouse behaviour (including anxiety-like behaviour) is known to be influenced by housing conditions,43,44 types of cage enrichment45 and even the intensity of lights in animal housing units.46 Alternatively, other models of anxiety involving conditioned stress induced stimuli or events, with or without pain, may provide a more profound difference in anxiety-like behaviours. Other rodents, such as rats, have also been widely used in combination with other modes to create rodent models of anxiety, based on predator encounters, social instability and restraint stress. Rodents models evaluating anxiety have been shown to exhibit strong face, construct and predictive validity and rodent based behavioural tests have been paramount in drug development. Therefore, more detailed information about the environment in the HFD-induced anxiety model may be required to allow reproducibility.
5 Conclusions
Overall, our study strongly questions the relevance of using HFD to induce anxiety-related behaviours and ultimately ascertained that daily oral gavage did not enhance or influence these findings.
Author contributions
GCW, MVH, and PDC conceptualised the experiments; PDC acquired funding for this project; J-LC provided the CTMA sequencing platform; GCW and BB processed samples for amplicon sequencing. NMD and PDC provided the MNUT animal and molecular biology platforms. GCW performed all experimentation, microbial and statistical analyses. GCW, MVH, and PDC wrote, reviewed and edited the manuscript. All authors approved the manuscript.
Data availability
16S rRNA amplicon sequencing data are available at the Sequence Read Archive (Bioproject ID: PRJNA1161804) https://www.ncbi.nlm.nih.gov/sra.
Conflicts of interest
PDC is an inventor on patent applications dealing with the use of gut bacteria and their components in the treatment of diseases. PDC was a co-founder of The Akkermansia Company and Enterosys.
Acknowledgements
PDC is a honorary research director at FRS-FNRS (Fonds de la Recherche Scientifique) and the recipient of grants from FNRS (Projet de Recherche PDR-convention: FNRS T.0030.21, FRFS-WELBIO: WELBIO-CR-2022A-02P, EOS: program no. 40007505). We thank Bouazza Es Saadi, Anthony Puel, Henri Danthinne, Luc Gesche and Rose-Marie Goebbels for their skilled technical assistance and support.
References
- A. Slee, I. Nazareth, N. Freemantle and L. Horsfall, Trends in generalised anxiety disorders and symptoms in primary care: UK population-based cohort study, Br. J. Psychiatry, 2021, 218, 158–164 CrossRef PubMed.
- D. F. Santomauro, A. M. Mantilla Herrera, J. Shadid, P. Zheng, C. Ashbaugh, D. M. Pigott, C. Abbafati, C. Adolph, J. O. Amlag, A. Y. Aravkin, B. L. Bang-Jensen, G. J. Bertolacci, S. S. Bloom, R. Castellano, E Castro, S. Chakrabarti, J. Chattopadhyay, R. M. Cogen, J. K. Collins, X Dai, W. J. Dangel, C. Dapper, A. Deen, M. Erickson, S. B. Ewald, A. D. Flaxman, J. J. Frostad, N. Fullman, J. R. Giles, A. Z. Gired, G. Guo, J. He, M. Helak, E. N. Hulland, B. Idrisov, A. Lindstrom, E. Linebarger, P. A. Lotufo, R. Lozano, B. Magistro, D. Carvalho Malta, J. C. Månsson, F. Marinho, A. H. Mokdad, L. Monasta, P. Naik, S. Nomura, J. K. O’Halloran, S. M. Ostroff, M. Pasovic, L. Penberthy, R. C. J. Reiner, G. Reinke, A. L. P. Ribeiro, A. Sholokhov, R. J. D. Sorensen, E. Varavikova, A. T. Vo, R. Walcott, S. Watson, C. S. Wiysonge, B. Zigler, S. I. Hay, T. Vos, C. J. L. Murray, H. A. Whiteford and A. J. Ferrari, Global prevalence and burden of depressive and anxiety disorders in 204 countries and territories in 2020 due to the COVID-19 pandemic, Lancet, 2021, 398, 1700–1712 CrossRef.
- J. Alonso, Z. Liu, S. Evans-Lacko, E. Sadikova, N. Sampson, S. Chatterji, J. Abdulmalik, S. Aguilar-Gaxiola, A. Al-Hamzawi, L. H. Andrade, R. Bruffaerts, G. Cardoso, A. Cia, S. Florescu, G. de Girolamo, O. Gureje, J. M. Haro, Y. He, P. de Jonge, E. G. Karam, N. Kawakami, V. Kovess-Masfety, S. Lee, D. Levinson, M. E. Medina-Mora, F. Navarro-Mateu, B. E. Pennell, M. Piazza, J. Posada-Villa, M. Ten Have, Z. Zarkov, R. C. Kessler and G. Thornicroft, Treatment gap for anxiety disorders is global: Results of the World Mental Health Surveys in 21 countries, Depression Anxiety, 2018, 35, 195–208 CrossRef.
-
American Psychiatric Association, Anxiety disorders: DSM-5 selections, American Psychiatric Association Publishing, Arlington, VA, 2016 Search PubMed.
-
F. E. Bloom and D. J. Kupfer, Environmental factors in the etiology of anxiety, Raven Press, New York, NY, 1995 Search PubMed.
- T. Steimer, The biology of fear- and anxiety-related behaviors, Dialogues Clin. Neurosci., 2002, 4, 231–249 CrossRef.
- S. Fulton, L. Decarie-Spain, X. Fioramonti, B. Guiard and S. Nakajima, The menace of obesity to depression and anxiety prevalence, Trends Endocrinol. Metab., 2022, 33, 18–35 CrossRef CAS PubMed.
- Y. Milaneschi, W. K. Simmons, E. F. C. van Rossum and B. W. Penninx, Depression and obesity: evidence of shared biological mechanisms, Mol. Psychiatry, 2019, 24, 18–33 CrossRef CAS PubMed.
- P. D. Cani, R. Bibiloni, C. Knauf, A. Waget, A. M. Neyrinck, N. M. Delzenne and R. Burcelin, Changes in gut microbiota control metabolic endotoxemia-induced inflammation in high-fat diet-induced obesity and diabetes in mice, Diabetes, 2008, 57, 1470–1481 CrossRef CAS PubMed.
- M. Regnier, M. Van Hul, C. Knauf and P. D. Cani, Gut microbiome, endocrine control of gut barrier function and metabolic diseases, J. Endocrinol., 2021, 248, R67–R82 CAS.
- G. Zhao, E. S. Ford, S. Dhingra, C. Li, T. W. Strine and A. H. Mokdad, Depression and anxiety among US adults: associations with body mass index, Int. J. Obes., 2009, 33, 257–266 CrossRef CAS PubMed.
- M. Van Hul, A. M. Neyrinck, A. Everard, A. Abot, L. B. Bindels, N. M. Delzenne, C. Knauf and P. D. Cani, Role of the intestinal microbiota in contributing to weight disorders and associated comorbidities, Clin. Microbiol. Rev., 2024, e0004523, DOI:10.1128/cmr.00045-23.
- J. A. Bravo, P. Forsythe, M. V. Chew, E. Escaravage, H. M. Savignac, T. G. Dinan, J. Bienenstock and J. F. Cryan, Ingestion of Lactobacillus strain regulates emotional behavior and central GABA receptor expression in a mouse via the vagus nerve, Proc. Natl. Acad. Sci. U. S. A., 2011, 108, 16050–16055 CrossRef CAS.
- S. S. Kang, P. R. Jeraldo, A. Kurti, M. E. Miller, M. D. Cook, K. Whitlock, N. Goldenfeld, J. A. Woods, B. A. White, N. Chia and J. D. Fryer, Diet and exercise orthogonally alter the gut microbiome and reveal independent associations with anxiety and cognition, Mol. Neurodegener., 2014, 9, 36 CrossRef PubMed.
- L. C. Bridgewater, C. Zhang, Y. Wu, W. Hu, Q. Zhang, J. Wang, S. Li and L. Zhao, Gender-based differences in host behavior and gut microbiota composition in response to high fat diet and stress in a mouse model, Sci. Rep., 2017, 7, 10776 CrossRef PubMed.
- H. Wu, W. Zhang, M. Huang, X. Lin and J. Chiou, Prolonged High-Fat Diet Consumption throughout Adulthood in Mice Induced Neurobehavioral Deterioration via Gut-Brain Axis, Nutrients, 2023, 15(2), 392 CrossRef CAS.
- A. J. Bruce-Keller, J. M. Salbaum, M. Luo, E. T. Blanchard, C. M. Taylor, D. A. Welsh and H. R. Berthoud, Obese-type gut microbiota induce neurobehavioral changes in the absence of obesity, Biol. Psychiatry, 2015, 77, 607–615 CrossRef PubMed.
- L. Grover, K. Sklioutovskaya-Lopez, J. K. Parkman, K. Wang, E. Hendricks, J. Adams-Duffield and J. H. Kim, Diet, sex, and genetic predisposition to obesity and type 2 diabetes modulate motor and anxiety-related behaviors in mice, and alter cerebellar gene expression, Behav. Brain Res., 2023, 445, 114376 CrossRef CAS PubMed.
- A. L. Dinel, C. Andre, A. Aubert, G. Ferreira, S. Laye and N. Castanon, Cognitive and emotional alterations are related to hippocampal inflammation in a mouse model of metabolic syndrome, PLoS One, 2011, 6, e24325 CrossRef CAS PubMed.
- C. Andre, A. L. Dinel, G. Ferreira, S. Laye and N. Castanon, Diet-induced obesity progressively alters cognition, anxiety-like behavior and lipopolysaccharide-induced depressive-like behavior: focus on brain indoleamine 2,3-dioxygenase activation, Brain, Behav., Immun., 2014, 41, 10–21 CrossRef CAS PubMed.
- N. Yamada, G. Katsuura, Y. Ochi, K. Ebihara, T. Kusakabe, K. Hosoda and K. Nakao, Impaired CNS leptin action is implicated in depression associated with obesity, Endocrinology, 2011, 152, 2634–2643 CrossRef CAS.
- S. Sharma and S. Fulton, Diet-induced obesity promotes depressive-like behaviour that is associated with neural adaptations in brain reward circuitry, Int. J. Obes., 2013, 37, 382–389 CrossRef CAS.
- Y. Y. Lam, S. F. Tsai, P. C. Chen, Y. M. Kuo and Y. W. Chen, Pioglitazone rescues high-fat diet-induced depression-like phenotypes and hippocampal astrocytic deficits in mice, Biomed. Pharmacother., 2021, 140, 111734 CrossRef CAS.
- F. D. Heyward, R. G. Walton, M. S. Carle, M. A. Coleman, W. T. Garvey and J. D. Sweatt, Adult mice maintained on a high-fat diet exhibit object location memory deficits and reduced hippocampal SIRT1 gene expression, Neurobiol. Learn. Mem., 2012, 98, 25–32 CrossRef CAS.
- H. Huang, J. Huang, W. Lu, Y. Huang, R. Luo, L. Bathalian, M. Chen and X. Wang, A Four-Week High-Fat Diet Induces Anxiolytic-like Behaviors through Mature BDNF in the mPFC of Mice, Brain Sci., 2024, 14(4) CrossRef.
- A. Klindworth, E. Pruesse, T. Schweer, J. Peplies, C. Quast, M. Horn and F. O. Glockner, Evaluation of general 16S ribosomal RNA gene PCR primers for classical and next-generation sequencing-based diversity studies, Nucleic Acids Res., 2013, 41, e1 CrossRef CAS PubMed.
- B. J. Callahan, P. J. McMurdie, M. J. Rosen, A. W. Han, A. J. Johnson and S. P. Holmes, DADA2: High-resolution sample inference from Illumina amplicon data, Nat. Methods, 2016, 13, 581–583 CrossRef CAS PubMed.
- C. Quast, E. Pruesse, P. Yilmaz, J. Gerken, T. Schweer, P. Yarza, J. Peplies and F. O. Glockner, The SILVA ribosomal RNA gene database project: improved data processing and web-based tools, Nucleic Acids Res., 2013, 41, D590–D596 CrossRef CAS PubMed.
- L. P. Noldus, A. J. Spink and R. A. Tegelenbosch, EthoVision: a versatile video tracking system for automation of behavioral experiments, Behav. Res. Methods Instrum. Comput., 2001, 33, 398–414 CrossRef CAS.
- S. J. P. Huwart, A. de Wouters d’Oplinter, M. Rastelli, M. Van Hul, W. M. de Vos, S. Luquet, P. D. Cani and A. Everard, Food Reward Alterations during Obesity Are Associated with Inflammation in the Striatum in Mice: Beneficial Effects of Akkermansia muciniphila, Cells, 2022, 11(16), 2534 CrossRef CAS PubMed.
- E. F. Osimo, T. Pillinger, I. M. Rodriguez, G. M. Khandaker, C. M. Pariante and O. D. Howes, Inflammatory markers in depression: A meta-analysis of mean differences and variability in 5,166 patients and 5,083 controls, Brain, Behav., Immun., 2020, 87, 901–909 CrossRef CAS.
- H. Costello, R. L. Gould, E. Abrol and R. Howard, Systematic review and meta-analysis of the association between peripheral inflammatory cytokines and generalised anxiety disorder, BMJ Open, 2019, 9, e027925 CrossRef PubMed.
- F. Xu, L. Han, Y. Wang, D. Deng, Y. Ding, S. Zhao, Q. Zhang, L. Ma and X. Chen, Prolonged anesthesia induces neuroinflammation and complement-mediated microglial synaptic elimination involved in neurocognitive dysfunction and anxiety-like behaviors, BMC Med, 2023, 21, 7 CrossRef CAS PubMed.
- C. N. Shi, X. M. Wu, Y. Z. Gao, D. Q. Ma, J. J. Yang and M. H. Ji, Oxytocin attenuates neuroinflammation-induced anxiety through restoration of excitation and inhibition balance in the anterior cingulate cortex in mice, J. Affective Disord., 2024, 362, 341–355 CrossRef CAS.
- A. Kohsaka, A. D. Laposky, K. M. Ramsey, C. Estrada, C. Joshu, Y. Kobayashi, F. W. Turek and J. Bass, High-fat diet disrupts behavioral and molecular circadian rhythms in mice, Cell Metab., 2007, 6, 414–421 CrossRef CAS PubMed.
- A. A. Walf and C. A. Frye, The use of the elevated plus maze as an assay of anxiety-related behavior in rodents, Nat. Protoc., 2007, 2, 322–328 CrossRef CAS PubMed.
- S. Pellow and S. E. File, Anxiolytic and anxiogenic drug effects on exploratory activity in an elevated plus-maze: a novel test of anxiety in the rat, Pharmacol., Biochem. Behav., 1986, 24, 525–529 CrossRef CAS.
- W. Mizunoya, K. Ohnuki, K. Baba, H. Miyahara, N. Shimizu, K. Tabata, T. Kino, Y. Sato, R. Tatsumi and Y. Ikeuchi, Effect of dietary fat type on anxiety-like and depression-like behavior in mice, SpringerPlus, 2013, 2, 165 CrossRef PubMed.
- P. J. Pistell, C. D. Morrison, S. Gupta, A. G. Knight, J. N. Keller, D. K. Ingram and A. J. Bruce-Keller, Cognitive impairment following high fat diet consumption is associated with brain inflammation, J. Neuroimmunol., 2010, 219, 25–32 CrossRef CAS PubMed.
- S. M. O'Mahony, G. Clarke, Y. E. Borre, T. G. Dinan and J. F. Cryan, Serotonin, tryptophan metabolism and the brain-gut-microbiome axis, Behav. Brain Res., 2015, 277, 32–48 CrossRef.
- L. Zou, Y. Tian, Y. Wang, D. Chen, X. Lu, Z. Zeng, Z. Chen, C. Lin and Y. Liang, High-cholesterol diet promotes depression- and anxiety-like behaviors in mice by impact gut microbe and neuroinflammation, J. Affective Disord., 2023, 327, 425–438 CrossRef CAS.
- E. Beurel, L. E. Harrington and R. S. Jope, Inflammatory T helper 17 cells promote depression-like behavior in mice, Biol. Psychiatry, 2013, 73, 622–630 CrossRef CAS PubMed.
- I. Abidin, H. Keser, E. Sahin, H. Ozturk, H. Basoglu, A. Alver and S. Aydin-Abidin, Effects of housing conditions on stress, depressive like behavior and sensory-motor performances of C57BL/6 mice, Lab. Anim. Res., 2024, 40, 6 CrossRef CAS PubMed.
- Y. Horii, T. Nagasawa, H. Sakakibara, A. Takahashi, A. Tanave, Y. Matsumoto, H. Nagayama, K. Yoshimi, M. T. Yasuda, K. Shimoi and T. Koide, Hierarchy in the home cage affects behaviour and gene expression in group-housed C57BL/6 male mice, Sci. Rep., 2017, 7, 6991 CrossRef.
- D. P. Wolfer, O. Litvin, S. Morf, R. M. Nitsch, H. P. Lipp and H. Wurbel, Laboratory animal welfare: cage enrichment and mouse behaviour, Nature, 2004, 432, 821–822 CrossRef CAS PubMed.
- A. Kapogiannatou, E. Paronis, K. Paschidis, A. Polissidis and N. G. Kostomitsopoulos, Effect of light colour temperature and intensity on the behaviour of male C57CL/6J mice, Appl. Anim. Behav. Sci., 2016, 184, 135–140 CrossRef.
|
This journal is © The Royal Society of Chemistry 2025 |
Click here to see how this site uses Cookies. View our privacy policy here.