DOI:
10.1039/D3SD00307H
(Paper)
Sens. Diagn., 2024,
3, 448-454
A fluorescent sp2c-covalent organic polymer with an aggregation-induced emission unit to suppress aggregation-induced quenching for sensing furazolidone†
Received
18th November 2023
, Accepted 24th January 2024
First published on 29th January 2024
Abstract
Furazolidone (FZD) is a nitrofuran antibiotic that has an inhibitory effect on a variety of Gram-negative and positive bacteria. However, excessive use of FZD can produce major adverse responses and irreversible harm to the central nervous system, so it is necessary to develop a simple, rapid, highly selective and sensitive method for the detection of FZD. Here, a sp2c-covalent organic polymer (COPPDAN-BTT) containing benzotrithiophene was synthesized for fluorescence sensing FZD. The benzotrithiophene in the BTT monomer is a typical aggregation-induced quenching (ACQ) fluorophore, and the cyanide diphenylethylene unit has certain aggregation-induced emission (AIE) properties. The connection of the ACQ fluorophore and the AIE fluorophore can fully improve the ACQ degree of COPPDAN-BTT. Under excitation at 370 nm, COPPDAN-BTT exhibits green fluorescence at 500 nm. Based on the internal filtering effect, COPPDAN-BTT was used for FZD detection. The I0/I showed a good linear relationship with the concentration of FZD in the range of 0.034–20 μg mL−1 (0.149–88.9 μM), and the detection limit was as low as 0.011 μg mL−1 (0.049 μM). Based on the AIE properties of COPPDAN-BTT, a fluorescent test strip was prepared for visual detection of FZD. This work provides a simple yet effective method for detection of FZD and improving the fluorescence of COPs.
1. Introduction
Furazolidone (FZD) is a nitrofuran antibiotic that has an inhibitory effect on a variety of Gram-negative and positive bacteria and can be used to stimulate growth and treat a range of gastrointestinal diseases caused by bacterial and protozoal infections in poultry and aquaculture animals.1 However, many studies have shown that FZD residues and their metabolites (3-amino-2-oxazolidinone) in foods cause potentially carcinogenic, mutagenic, and genotoxic effects. It can also damage the human liver and deoxyribonucleic acid (DNA) as well as inhibit cell growth.2 Thus, it is necessary to develop a simple, rapid, highly selective and sensitive method for the detection of FZD residues.3 At present, high performance liquid chromatography,4 liquid chromatography-mass spectrometry,5 triple tandem liquid chromatography-mass spectrometry6 and enzyme-linked immunoassay7 have been used for the detection of FZD. However, shortcomings such as time-consuming, expensive, and complex equipment, complicated sample preparation and difficulty in field inspection limit the wide application of the above traditional methods. Fluorescent sensors have attracted more and more attention because of their advantages such as simple operation, high sensitivity, good selectivity and strong visual recognition ability in recent years.8–10
Covalent organic polymers (COPs) are materials formed by stable covalent bonds with backbones composed of lightweight elements such as C, H, N, O, F, and S.11–14 COPs have the advantages of structural diversity, large specific surface area, controllable chemical and physical properties, abundant π-conjugated systems, and various synthesis strategies.15,16 In addition, they can interact well with other molecules through non-covalent interactions, so they can be widely used in gas separation and storage, energy storage, catalysis, energy conversion and sensing.17,18 Compared with metal–organic frameworks (MOFs),19 COPs connected by covalent bonds have higher stability. In addition, COPs are metal-free materials with excellent biocompatibility and low biotoxicity.20 Therefore, COPs with extended π-conjugated main chains and high stability are widely used in the construction of fluorescent sensors.21 However, the aggregate fluorescence quenching (ACQ) phenomenon is a serious obstruction in fluorescent sensors based on COPs.
Traditional luminescent groups with planarity and strong intermolecular interaction generally emit bright light in the monodisperse state, while the luminescence is weakened or even quenched in the aggregated state, which is called ACQ. Nevertheless, several strategies have been developed to improve the fluorescence of COPs. For example, Wang et al. designed and synthesized a hydrazine-linked TFBE-TD COP with yellow-green fluorescence. The COP exhibited a good backbone structure and aggregation-induced limitation of synergistic effects of tetraphenylene (TPE) molecules in the solid state, which resulted in improved fluorescence quantum yields of the relaxation.22 Zhang et al. designed and synthesized a novel TPE-based COP by the Suzuki coupling reaction, which showed high selectivity. Meanwhile, some molecules emit weak or no light in dilute solution, but emit more light in the aggregated state or solid state. This phenomenon is called “aggregation-induced emission” (AIE). By connecting the AIE group with the traditional planar ACQ molecule,23 the solid state luminescence of the molecule is promoted through the restricted intramolecular rotation process of the AIE unit,24 the ACQ effect of the planar luminescent group is effectively eliminated, and the conversion from ACQ to AIE is realized.25,26
In this work, a sp2c-covalent COP was synthesized by the Knoevenagel condensation reaction between benzo[1,2-b:3,4-b′:5,6-b′′]trithiophene-2,5,8-tricarbaldehyde (BTT) and 1,4-phenylenediacetonitrile (PDAN). Cyanide diphenylethylene, which is the linkage unit in the sp2c-covalent COPPDAN-BTT, is an AIE active unit with a distorted conformation. The twisted molecular conformation originating from the steric hindrance between the neighboring phenyl rings and the nitrile moiety obstructs the intermolecular π–π stacking and thus prevents the emission quenching in the aggregated state.27 Under excitation at 370 nm, the synthesized COPPDAN-BTT has greenish-yellow fluorescence emission at 500 nm. There was an inner filter effect (IFE) between COPPDAN-BTT and FZD, which significantly quenched the yellow-green fluorescence of COPPDAN-BTT. Using COPPDAN-BTT as the fluorescent probe, the I0/I showed a good linear relationship with the concentration of FZD in the range of 0.034–20 μg mL−1 (0.149–88.9 μM), with the lowest detection limit as low as 0.011 μg mL−1 (0.049 μM).28
2. Experimental section
2.1. Synthesis of COPPDAN-BTT and COPPDAN-TF
For the preparation of COPPDAN-BTT, BTT (0.1 mmol) and PDAN (0.15 mmol) were dissolved in a mixture of 2 mL mesitylene/1,4-dioxane (1
:
5), respectively. After complete dissolution by ultrasonication, the two solutions were transferred to a Schlenk tube with a capacity of 10 mL and mixed evenly. Then, NaOH (0.4 mL, 4 mol L−1) solution was added as a catalyst. Next, the tube was frozen at 77 K (liquid N2 bath) and degassed by three freeze–pump–thaw cycles. After that, the tube was sealed off and then heated at 120 °C for 3 days. After the reactor was cooled to room temperature, the precipitate was obtained by centrifugation at 10
000 rpm for 10 min. DMF and ethanol were added to wash the product to remove the unreacted reagent, and the above operation was repeated at least 3 times. After centrifugation, the yellow-brown product was obtained. The COPPDAN-BTT was dried in a freeze dryer for 6 h. In order to obtain good dispersion, the obtained COPPDAN-BTT was ground in an agate mortar.
As a comparison, COPPDAN-TF was also prepared. The preparation process of COPPDAN-TF is similar to that of COPPDAN-BTT. Only BTT (0.1 mmol) was changed to TF (0.1 mmol), and the reaction solvent was changed to 1,4-dioxane, and finally a white powder was obtained. The synthesis process is shown in Scheme 1.
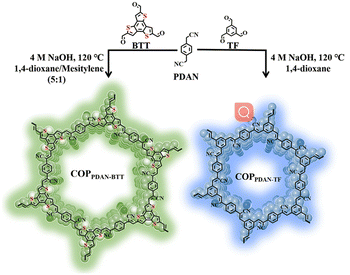 |
| Scheme 1 Synthesis route of COPPDAN-BTT and COPPDAN-TF. | |
2.2. Fluorescence detection method
The COPPDAN-BTT and COPPDAN-TF powders (2.0 mg) were dissolved in PBS (1.0 mL) to prepare a solution (2.0 mg mL−1). The concentration of FZD was determined by fluorescence titration in PBS with pH = 8 (COPPDAN-BTT) and pH = 7 (COPPDAN-TF) with COPs. The emission spectrum was recorded under 370 nm (COPPDAN-BTT) and 350 nm (COPPDAN-TF) excitation, and the emission peak intensities at 500 nm (COPPDAN-BTT) and 430 nm (COPPDAN-TF) were recorded and analyzed. Selectivity experiments were carried out using the above method. FZD was replaced with interferents, and the concentration of the interferents in the probe solution was 100 μg mL−1, and the concentration of NF was 10 μg mL−1.
2.3. Production of fluorescent test strips
Non-fluorescent filter paper was cut into 1 cm diameter discs and immersed in well-dispersed COPPDAN-BTT and COPPDAN-TF solutions at a concentration of 5 mg mL−1 for 6 h, then removed and laid flat on Petri dishes and dried under an infrared lamp for subsequent experiments.
3. Results and discussion
3.1. Structural characterization of COPs
The morphology of COPs was characterized by scanning electron microscopy (SEM) and atomic force microscopy (AFM). It can be seen from the SEM images that both COPPDAN-BTT and COPPDAN-TF are ultra-thin two-dimensional lamellated structures (Fig. 1a and b). The thickness of COPPDAN-BTT and COPPDAN-TF measured by AFM was about 1.5 nm (Fig. 1d and e). The bonding, crystallinity and structure of COPPDAN-BTT and COPPDAN-TF were further characterized by FTIR, PXRD and N2 adsorption/desorption isotherms. The FTIR spectrum of COPPDAN-BTT is shown in Fig. 1c. It was found that the C–H stretching vibration (2860 cm−1) of the aldehyde group coming from BTT disappeared and the C
O stretching vibration (1670 cm−1) of the aldehyde group decreased significantly. The –CH
C–CN formation caused an increase in π-electron delocalization, which shifted the C
N stretching vibrational peak from 2249 cm−1 in PDAN to 2214 cm−1 in COPPDAN-BTT. The change of the FTIR spectrum of COPPDAN-TF in Fig. S1a† is similar to that of COPPDAN-BTT. There was a significant difference between the FTIR spectra of the COPs and their monomers, indicating the successful synthesis of the COPs.29
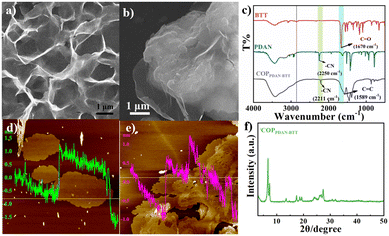 |
| Fig. 1 SEM (a and b) and AEM (d and e) images of COPPDAN-BTT (a and d) and COPPDAN-TF (b and e). FTIR spectra of COPPDAN-BTT (c) and its monomers. XRD pattern of COPPDAN-BTT (f). | |
The crystallinity of COPPDAN-BTT and COPPDAN-TF was studied by powder X-ray diffraction (PXRD) (Fig. 1f).30 COPPDAN-BTT has strong peaks at 6.68° and 7.30°, indicating that COPPDAN-BTT has good crystallinity. However, COPPDAN-TF has a wide diffraction peak at 20°, which indicates that COPPDAN-TF is amorphous. As shown in Fig. S2,† the N2 adsorption/desorption isotherm of COPPDAN-BTT belongs to the typical type IV isotherm, while the N2 adsorption/desorption isotherm of COPPDAN-TF belongs to the typical type II isotherm. The Brunauer–Emmett–Teller (BET) surface areas of COPPDAN-BTT and COPPDAN-TF are 117.9 m2 g−1 and 110.2 m2 g−1, respectively. According to the pore size distribution of COPPDAN-BTT and COPPDAN-TF, the pore sizes of COPPDAN-BTT and COPPDAN-TF are 2.84 nm and 1.53 nm, respectively. The thermogravimetric analysis (TGA) of COPPDAN-BTT and COPPDAN-TF is shown in Fig. S3,† revealing their good thermal stability.
3.2. Optical properties of COPs
BTT with large conjugate planes tends to produce strong π–π interaction in the aggregated state, resulting in energy loss, and shows no or weak fluorescence, but green fluorescence can be observed in dilute solution (Fig. 2a). Due to their small conjugation degree, PDAN and TF, consisting of one benzene ring, exhibit weak fluorescence under 360 nm excitation (Fig. 2b and c).31 Since the conjugation degree of COPPDAN-TF synthesized by TF and PDAN was significantly increased, strong blue fluorescence was observed at 430 nm and its CIE chromaticity coordinates were (0.1627, 0.1248) (Fig. 2e and f). It has been shown that the linking unit (cyanide diphenylethylene) is an AIE active unit with a non-planar twisted configuration. The π–π interactions between parallel cyanide diphenylethylene units in the two-dimensional COP plane can be confined by the large volume and strong polarity of –CN in cyanide diphenylethylene, resulting in J-aggregates with AIE activity. sp2c-COPs with a cyanide stilbene structure as the connecting unit have certain AIE properties.32 Under excitation at 400 nm (Fig. 2h), the COPPDAN-TF powder exhibits strong yellow-green fluorescence at 500 nm, and its CIE chromaticity coordinates are (0.3171, 0.3982) (Fig. 2i), which proves the existence of their AIE properties. In order to inhibit the ACQ properties of BTT, COPPDAN-BTT was synthesized with BTT and PDAN.33 The aqueous solution of COPPDAN-BTT has green fluorescence at 500 nm (Fig. 2d), which is derived from the monomer BTT, with CIE chromaticity coordinates of (0.2583, 0.4013) (Fig. 2f). Under 420 nm excitation (Fig. 2g), the COPPDAN-BTT powder exhibited bright orange-yellow fluorescence with CIE chromaticity coordinates of (0.5319, 0.4460) (Fig. 2i). The strong solid fluorescence of COPPDAN-BTT indicates that COPPDAN-BTT has a significant AIE effect, that is, ACQ to AIE conversion is realized. Compared with the fluorescence in aqueous solution, the solid fluorescence of COPPDAN-BTT and COPPDAN-TF showed an obvious redshift phenomenon (Fig. 2).34
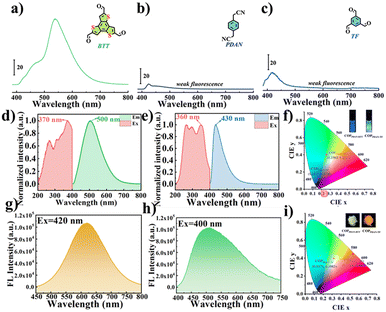 |
| Fig. 2 Fluorescence spectra of aqueous solutions of (a) BTT, (b) PDAN and (c) TF. Fluorescence excitation and emission spectra of aqueous solutions of (d) COPPDAN-BTT and (e) COPPDAN-TF. (f) CIE chromaticity diagrams of aqueous solutions of COPPDAN-BTT and COPPDAN-TF. Fluorescence emission spectra of (g) COPPDAN-BTT and (h) COPPDAN-TF powders. (i) CIE chromaticity diagrams of COPPDAN-BTT and COPPDAN-TF powders. Insets: Photographs of COPPDAN-BTT and COPPDAN-TF under a UV lamp at 365 nm. | |
Then, the solubility of COPPDAN-BTT and COPPDAN-TF in different solvents was investigated. COPPDAN-BTT is well dispersed in water and partially dispersed in moderate-polar DMA and DMF, but it is completely insoluble in weakly polar THF (Fig. 3a). Under a 365 nm UV lamp, it can be observed that COPPDAN-BTT presents yellow-green fluorescence in aqueous solution, light yellow fluorescent and some orange fluorescent particles exist in DMA, scattered orange fluorescent particles exist in DMF, but orange fluorescent particles with obvious aggregation are found in THF (Fig. 3b).35 The orange fluorescence of the orange fluorescent particles can be considered as solid fluorescence of COPPDAN-BTT. The results show that COPPDAN-BTT has good solubility in strong polar solvent. As shown in Fig. 3d and e, compared with COPPDAN-BTT, COPPDAN-TF was less decentralized. When COPPDAN-TF was dispersed in water, it showed blue fluorescence.36 With the decrease of polarity, the solubility decreased gradually. As shown in Fig. S4,† the stability of COPPDAN-TF is poor due to its easy re-aggregation in solution, and the Tyndall effect is weak. The standard deviation of the fluorescence emission peaks at 430 nm was 36.2038. However, COPPDAN-BTT had good dispersion and an obvious Tyndall effect. The standard deviation of the fluorescence emission peaks at 500 nm was 0.7071. The good solubility of COPPDAN-BTT in water can be attributed to the presence of a large number of cyanide groups in the COP skeleton. As COPs are composed of a large number of benzene rings, generally speaking, the solubility of COPs in water is very poor, while the good solubility of sp2c-COPs in water provides convenient conditions for the detection of FZD in the actual environment.37
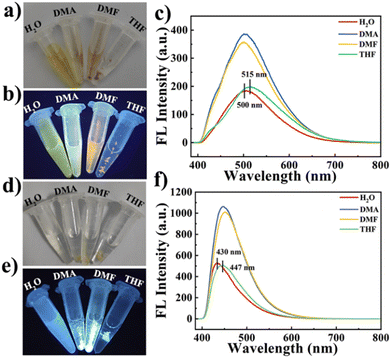 |
| Fig. 3 Photographs of COPPDAN-BTT dissolved in different solvents (from left to right: H2O, DMA, DMF and THF) under visible light (a) and a UV lamp (b). (c) Fluorescence emission spectra of COPPDAN-BTT in different solvents. COPPDAN-TF dissolved in different solvents (from left to right: H2O, DMA, DMF and THF) under visible light (d) and a UV lamp (e). (f) Fluorescence emission spectra of COPPDAN-TF in different solvents. The dissolution concentration is 1 mg mL−1 (a, b, d and e) and the fluorescence test concentration is 120 μg mL−1 (c and f). | |
Then the fluorescence properties of COPPDAN-BTT and COPPDAN-TTF in different solvents were studied. As shown in Fig. 3c, the fluorescence intensity of COPPDAN-BTT in water was significantly lower than that in DMA and DMF. This is attributed to the AIE properties of COPPDAN-BTT, that is, in a medium-polar solvent due to low solubility, COPPDAN-BTT agglomerates into small particles suspended in a solution and accordingly the fluorescence is enhanced greatly. In addition, in water the fluorescence emission peak of COPPDAN-BTT was at 500 nm, but in THF the fluorescence emission peak of COPPDAN-BTT was at 515 nm. The blueshift with increasing polarity can be attributed to the intramolecular charge transfer (ICT) nature of COPPDAN-BTT, where the cyanide group of COPPDAN-BTT is a strong electron-absorbing group and benzotrithiophene is an electron-donating group, and there is an ICT effect from benzotrithiophene to cyano. The binding of the electron donor to the solvent weakens the ability to drive electrons and reduces the charge separation of the system, resulting in a blue shift. The case of COPPDAN-TTF is similar to that of COPPDAN-BTT. The cyanide group of COPPDAN-TF is a strong electron-absorbing group, and the benzene ring is an electron-giving group, and there exists an ICT effect. In THF, the fluorescence emission peak of COPPDAN-TF is positioned at 447 nm, while with increasing polarity, the fluorescence emission peak of COPPDAN-TF in water is blue-shifted to 430 nm (Fig. 3f).38
To verify the AIE properties of COPPDAN-BTT and COPPDAN-TF, the fluorescence emission of COPPDAN-BTT and COPPDAN-TF in solvents with different viscosities was further examined. The conversion of AIE molecules from weak fluorescence in the single-molecule state to strong fluorescence in the aggregated state is achieved through restricted intramolecular motion. Therefore, in high-viscosity solvents, AIE molecules exhibit enhanced fluorescence due to restricted vibration or rotation. Glycerol alcohol is a liquid with high viscosity (800 at 25 °C). Solutions with different viscosities can be obtained by mixing glycerol and water in different proportions. As shown in Fig. S5,† with the enhancement of viscosity, the fluorescence of COPPDAN-BTT and COPPDAN-TF is gradually limited in intramolecular vibration and rotation, and the fluorescence shows an increasing trend. Therefore, the results show that COPPDAN-BTT and COPPDAN-TF have AIE properties.
3.3. Sensing performance of COPPDAN-BTT and COPPDAN-TF for FZD
In order to obtain better detection results, the amount of probe and the pH of the detection environment were optimized (Fig. S6†). The fluorescence responses of COPPDAN-BTT and COPPDAN-TF to FZD were studied under the above optimized conditions.39 As shown in Fig. 4a, with the increase of the concentration of FZD, the fluorescence of COPPDAN-BTT at 500 nm was continuously quenched, and the fluorescence emission peak of COPPDAN-TF at 430 nm was continuously decreased (Fig. 4d). As shown in Fig. 4b, FZD was detected in the concentration range of 0.034–20 μg mL−1 (0.149–88.9 μM) with a good linear relationship between I0/I and the concentration of FZD, and the detection limit was as low as 0.011 μg mL−1 (0.049 μM) at S/R = 3. As shown in Fig. 4e, two linear relationships were obtained for FZD in the concentration range of 0.069–14 μg mL−1 (0.307–62.2 μM) and 14–28 μg mL−1 (62.2–124.4 μM), respectively. The I0/I of COPPDAN-TF obtained two linear relationships with the corresponding detection limit of 0.023 μg mL−1 (0.101 μM), which was lower than that of COPPDAN-BTT. Compared with other analytical methods and other reported FZD fluorescent sensors, COPPDAN-BTT and COPPDAN-TF both have better detection performance (Table S1†). To evaluate the selectivity of COPPDAN-BTT and COPPDAN-TF for FZD detection, we added FZD and some potential interfering substances (Na+, K+, Ag+, Fe3+, Al3+, Hg2+, EF, AA, L-dopa, tBHQ, AMX, AK, MT, FT) to a series of blank fluorescent probe solutions, respectively, and the corresponding fluorescence intensity changes were recorded. Apparently, there was no significant quenching of the fluorescence of COPPDAN-BTT and COPPDAN-TF in the presence of other interfering substances, indicating that COPPDAN-BTT and COPPDAN-TF have high selectivity for FZD fluorescence detection (Fig. 4c and f).40
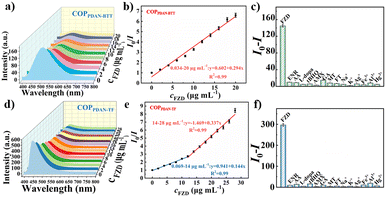 |
| Fig. 4 (a) Fluorescence emission spectra after the addition of different concentrations of FZD to COPPDAN-BTT (λex = 370 nm). (b) Linear plot between the FZD concentration and I0/I. (c) Fluorescence emission spectra of COPPDAN-BTT in the presence of FZD and other interferents (Na+, K+, Ag+, Fe3+, Al3+, Hg2+, ENR, AA, L-dopa, tBHQ, AMX, AK, MT, FT). (d) Fluorescence emission spectra (λex = 350 nm) after the addition of different concentrations of FZD to COPPDAN-TF. (e) Linear plot between the FZD concentration and I0/I. (f) Fluorescence emission spectra of COPPDAN-TF in the presence of FZD and other interferents (Na+, K+, Ag+, Fe3+, Al3+, Hg2+, ENR, AA, L-dopa, tBHQ, AMX, AK, MT, FT). The concentration of FZD was 10 μg mL−1 and the concentration of all other interferents was 100 μg mL−1. | |
The fluorescent colorimetric test strip can be used to observe the fluorescence brightness and color change by the naked eye, which has a better field survey effect. Using the AIE properties of COPPDAN-BTT and COPPDAN-TF, COPPDAN-BTT and COPPDAN-TF were fixed on filter paper to make portable test strips for visualization of FZD. Fig. 5 shows a test strip under UV lamp irradiation at 365 nm and bright green and blue fluorescence appeared. 5 μL of different concentrations of FZD solution (0.2, 0.4, 0.6, 0.8, 1.0, 1.2, 1.5 mg mL−1) were dropped on the test strips, and both COPPDAN-BTT and COPPDAN-TF test strips showed a significant change from bright to dark with the increase of FZD concentration. At the same FZD concentration, compared with COPPDAN-TF, the quenching of COPPDAN-BTT was more significant.41
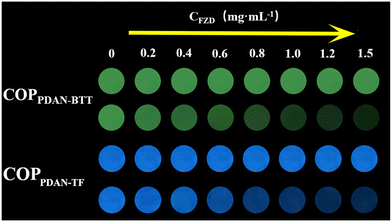 |
| Fig. 5 After 5 μL of different concentrations of FZD solution (0.2, 0.4, 0.6, 0.8, 1.0, 1.2, 1.5 mg mL−1) were dropped on the test strips, fluorescence changes of COPPDAN-BTT and COPPDAN-TF test paper with the addition of FZD under a UV lamp at 365 nm. | |
3.4. Detection mechanism of COPPDAN-BTT and COPPDAN-TF for FZD
The mechanism of FZD quenching luminescence was further investigated by fluorescence spectra and UV-vis absorption spectra. According to previous work, there are two possible mechanisms for the detection of FZD by COPPDAN-BTT and COPPDAN-TF: fluorescence resonance energy transfer (FRET) and internal filtering effect (IFE).42 An obvious absorption peak of FZD can be observed at 375 nm, and the fluorescence emission peaks of COPPDAN-BTT and COPPDAN-TF are at 500 nm and 430 nm, respectively, without obvious overlap with the UV absorption peaks of FZD (Fig. 6a and c).43 The possible mechanism of fluorescence resonance energy transfer (FRET) is ruled out. However, the excitation wavelengths of COPPDAN-BTT and COPPDAN-TF were 370 nm and 350 nm, respectively, which were in the range of the UV absorption peak of FZD. This proved that the quenching mechanism of COPPDAN-BTT and COPPDAN-TF on FZD was the IFE (Fig. 6b and d).44
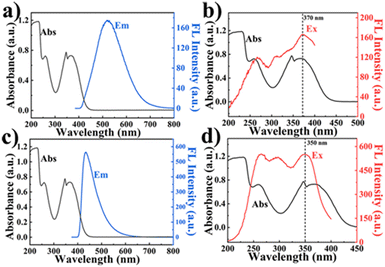 |
| Fig. 6 (a) Fluorescence emission spectrogram of COPPDAN-BTT and UV-vis absorption spectra of FZD. (b) Fluorescence excitation spectrogram of COPPDAN-BTT and UV-vis absorption spectra of FZD. (c) Fluorescence emission spectrogram of COPPDAN-TF and UV-vis absorption spectra of FZD. (d) Fluorescence excitation spectrogram of COPPDAN-TF and UV-vis absorption spectra of FZD. | |
3.5. Detection of FZD in the actual samples
We examined the FZD residues in lake water samples using COPPDAN-BTT and COPPDAN-TF, respectively. The samples were filtered with filter paper to remove the solid impurities in the lake water before testing, and then different concentrations of FZD (5, 10, 20 μg mL−1) were added to the samples using the standard addition method for the determination. The results showed that there was no significant FZD residue in the lake water, and the spiked recoveries were within 95.8–102.6% (Table S2†).
4. Conclusions
In summary, we have successfully synthesized COPPDAN-BTT by the Knoevenagel reaction. BTT and PDAN were selected to synthesize COPPDAN-BTT with AIE properties for the first time, which achieves the conversion from ACQ to AIE and is used to detect FZD. Under 370 nm excitation, the yellow-green fluorescence emission of COPPDAN-BTT was present at 500 nm. The addition of FZD quenched the yellow-green fluorescence of COPPDAN-BTT due to the presence of the IFE with FZD. Using COPPDAN-BTT as the fluorescent probe, the I0/I showed a good linear relationship with the concentration of FZD in the range of 0.034–20 μg mL−1 (0.149–88.9 μM), with the lowest detection limit as low as 0.011 μg mL−1 (0.049 μM). Thus, COPPDAN-BTT showed great activity with a lower detection limit, wide linear range, good interference and stability for FZD detection. The AIE properties were used to produce test strips for the visualization of FZD. With these excellent properties, the proposed test strips could be applied for future analytical applications in real-world samples, such as residual furazolidones in aquaculture.
Author contributions
Xinyi Wang: data analysis, resources, investigation, writing – original draft. Lijuan Kuang: methodology, validation. Meiling Ye: investigation. Liangmei Zou: investigation. Li Wang: methodology, writing – review & editing, supervision. Yonghai Song: conceptualization, funding acquisition, supervision, writing – review & editing.
Conflicts of interest
The authors declare that they have no conflicts of interest.
Acknowledgements
This work was financially supported by the National Natural Science Foundation of China (21665012, 21765009, 21964010, and 22264016).
References
- N. Riswana Barveen, T.-J. Wang and Y.-H. Chang, Chem. Eng. J., 2021, 423, 130191 CrossRef CAS.
- E. A. Kumar, T. Kokulnathan, T.-J. Wang, K. M. A. Kumar and Y.-H. Chang, J. Environ. Chem. Eng., 2023, 11, 1706–1712 Search PubMed.
- S. Zhang, Y. Mao, T. Song, X. Zhao, Z. Song and W. Wang, Carbon, 2023, 213, 118213 CrossRef CAS.
- R. S. van den Hurk, M. Pursch, D. R. Stoll and B. W. J. Pirok, TrAC, Trends Anal. Chem., 2023, 166, 117166 CrossRef CAS.
- M. F. Zaki, Y.-H. Wu, P.-C. Chen and P.-S. Chen, Sens. Actuators, B, 2023, 393, 134243 CrossRef CAS.
- R. S. Minhas, D. A. Rudd, H. Z. Al Hmoud, T. M. Guinan, K. P. Kirkbride and N. H. Voelcker, ACS Appl. Mater. Interfaces, 2020, 12, 31195–31204 CrossRef CAS PubMed.
- N. Li, H. Chen, M. Zhang, Y. Zha, Z. Mu, Y. Ma and P. Chen, Sens. Actuators, B, 2020, 315, 128135 CrossRef CAS.
- M. Li, H. Wan, J. Xiong, L. Wang, L. Yang, L. Wang and Y. Song, Chem. Eng. J., 2023, 468, 143538 CrossRef CAS.
- S. Wang, L. Guo, L. Chen, L. Wang and Y. Song, ACS Appl. Nano Mater., 2022, 5, 1339–1347 CrossRef CAS.
- L. Yang, M. Li, L. Kuang, Y. Li, L. Chen, C. Lin, L. Wang and Y. Song, Biosens. Bioelectron., 2022, 214, 114527 CrossRef CAS PubMed.
- L. Guo and D. Cao, J. Mater. Chem. C, 2015, 3, 8490–8494 RSC.
- H. Zhang, J. Zhou, G.-G. Shan, G.-F. Li, C.-Y. Sun, D.-X. Cui, X.-L. Wang and Z.-M. Su, Chem. Commun., 2019, 55, 12328–12331 RSC.
- E. Özdemir, D. Thirion and C. T. Yavuz, RSC Adv., 2015, 5, 69010–69015 RSC.
- G. Das, T. Prakasam, S. Nuryyeva, D. S. Han, A. Abdel-Wahab, J.-C. Olsen, K. Polychronopoulou, C. Platas-Iglesias, F. Ravaux, M. Jouiad and A. Trabolsi, J. Mater. Chem. A, 2016, 4, 15361–15369 RSC.
- K. Prakash Subodh and D. T. Masram, J. Mater. Chem. C, 2020, 8, 9201–9204 RSC.
- D. Yadav and S. K. Awasthi, Green Chem., 2020, 22, 4295–4303 RSC.
- Y. Chen, W. Li, R.-Z. Gao, X.-H. Wang, A.-N. Tang and D.-M. Kong, J. Mater. Chem. C, 2022, 10, 1236–1245 RSC.
- S. Qu, N. Song, G. Xu and Q. Jia, Sens. Actuators, B, 2019, 290, 9–14 CrossRef CAS.
- Y.-Q. Sun, Y. Cheng and X.-B. Yin, Anal. Chem., 2021, 93, 3559–3566 CrossRef CAS PubMed.
- L. Guo, L. Yang, M. Li, L. Kuang, Y. Song and L. Wang, Coord. Chem. Rev., 2021, 440, 213957 CrossRef CAS.
- L. Yang, Y. Song, C. Lin, L. Hou, L. Guo, Y. Lei and L. Wang, Sens. Actuators, B, 2021, 341, 129995 CrossRef CAS.
- R. Wang, C. He, Z. Xu, Y. Lei, Y. Jiang, J. Zhang, S. Chen and D. Zhang, Dyes Pigm., 2022, 206, 24570–24579 Search PubMed.
- D. Hu, H. Huang, R. Li, J. Yuan and Y. Wei, Sci. China: Chem., 2022, 65, 1532–1537 CrossRef CAS.
- Z. Gong, X. Rong, G. Sui, X. Jiang, M. Xu, C. Wang and S. Meng, Adv. Opt. Mater., 2023, 11, 2203075 CrossRef CAS.
- S. Dalapati, E. Jin, M. Addicoat, T. Heine and D. Jiang, J. Am. Chem. Soc., 2016, 138, 5797–5800 CrossRef CAS PubMed.
- P. Albacete, J. I. Martínez, X. Li, A. López-Moreno, S. a. Mena-Hernando, A. E. Platero-Prats, C. Montoro, K. P. Loh, E. M. Pérez and F. Zamora, J. Am. Chem. Soc., 2018, 140, 12922–12929 CrossRef CAS PubMed.
- J. Mei, N. L. C. Leung, R. T. K. Kwok, J. W. Y. Lam and B. Z. Tang, Chem. Rev., 2015, 115, 11718–11940 CrossRef CAS PubMed.
- Z. Cong, Z. Song, Y. Ma, M. Zhu, Y. Zhang, S. Wu and E. Gao, Chem. – Asian J., 2021, 16, 1773–1779 CrossRef CAS PubMed.
- R. Chen, J. L. Shi, Y. Ma, G. Lin, X. Lang and C. Wang, Angew. Chem., Int. Ed., 2019, 58, 6430–6434 CrossRef CAS PubMed.
- S. Ballur Prasanna, R. Sakthivel, L.-Y. Lin, Y.-F. Duann, J.-H. He, T.-Y. Liu and R.-J. Chung, Appl. Surf. Sci., 2023, 611, 155784 CrossRef CAS.
- C. Huang, S. Zhou, C. Chen, X. Wang, R. Ding, Y. Xu, Z. Cheng, Z. Ye, L. Sun, Z. J. Wang, D. Hu, X. Jia, G. Zhang and S. Gao, Small, 2022, 18, 220506 Search PubMed.
- G.-J. Li, J.-L. Ni, A. Mensah, M. Li, L.-Z. Chen and F.-M. Wang, J. Porous Mater., 2022, 29, 1531–1538 CrossRef CAS.
- Y. Cai, H. Zhu, W. Zhou, Z. Qiu, C. Chen, A. Qileng, K. Li and Y. Liu, Anal. Chem., 2021, 93, 7275–7282 CrossRef CAS PubMed.
- Y. Shen, Y. Wei, C. Zhu, J. Cao and D.-M. Han, Coord. Chem. Rev., 2022, 458, 759–770 Search PubMed.
- H. Sun, X. Li, Z. Hu, C. Gu, D. Chen, J. Wang, B. Li, T. Jiang and X. Zhou, Appl. Surf. Sci., 2021, 556, 149748 CrossRef CAS.
- H. Tan and Y. Li, Microchim. Acta, 2021, 188, 254–263 CrossRef CAS PubMed.
- J. Yang, Y. Cao, W. Si, J. Zhang, J. Wang, Y. Qu and W. Qin, ChemSusChem, 2022, 15, 578496 Search PubMed.
- Y. Yang, J. Chen, Z. Chen, Z. Yu, J. Xue, T. Luan, S. Chen and S. Zhou, Water Res., 2022, 223, 118979 CrossRef CAS PubMed.
- X. Yue, Z. Zhou, M. Li, M. Jie, B. Xu and Y. Bai, Food Chem., 2022, 367, 130763 CrossRef CAS PubMed.
- J. Zhang, F. Xue and Z. Wang, Chem. Mater., 2022, 34, 10701–10710 CrossRef CAS.
- Z. Zhao, H. Zhang, J. W. Y. Lam and B. Z. Tang, Angew. Chem., Int. Ed., 2020, 59, 9888–9907 CrossRef CAS PubMed.
- Z. Gao, M. Liu, K. Xu, M. Tang, X. Lin, S. Hu and X. Ren, Anal. Methods, 2020, 12, 2551–2554 RSC.
- Q.-X. Luo, W.-R. Cui, Y.-J. Li, Y.-J. Cai, X.-L. Mao, R.-P. Liang and J.-D. Qiu, ACS Appl. Electron. Mater., 2021, 3, 4490–4497 CrossRef CAS.
- X. Niu, X. Bo and L. Guo, Food Chem., 2021, 364, 130368 CrossRef CAS PubMed.
|
This journal is © The Royal Society of Chemistry 2024 |
Click here to see how this site uses Cookies. View our privacy policy here.