DOI:
10.1039/D4SC04979A
(Edge Article)
Chem. Sci., 2024,
15, 16096-16102
Spirobifluorene-based hole-transporting materials for RGB OLEDs with high efficiency and low efficiency roll-off†
Received
26th July 2024
, Accepted 3rd September 2024
First published on 6th September 2024
Abstract
In this work, we designed and synthesized three spirobifluorene (SBF)-based hole-transporting materials (HTMs) by incorporating the di-4-tolylamino group at different positions of the SBF skeleton. These materials demonstrate excellent thermal stability with thermal decomposition temperatures (Td) up to 506 °C and outstanding morphological stability with a glass transition temperature (Tg) exceeding 145 °C. The meta-linkage mode between the conjugated skeleton and functional groups in the molecular structure results in electronic decoupling, giving these 3,6-substituted SBFs higher triplet energies (ET) compared to 2,7-substituted SBFs. This makes the 3,6-substituted SBFs suitable as universal HTMs for red, green, and blue (RGB) organic light emitting diodes (OLEDs). Among the three HTMs, 3,3′,6,6′-tetra(N,N-ditolylamino)-9,9′-spirobifluorene (3,3′,6,6′-TDTA-SBF) exhibits the best device performance, achieving maximum external quantum efficiencies (EQEmax) of 26.1%, 26.4%, and 25.4% for RGB phosphorescent OLEDs, with extremely low efficiency roll-off in both green and blue devices. Utilizing 3,3′,6,6′-TDTA-SBF as the HTM, we have also fabricated narrowband blue OLEDs based on the widely used multiple resonance emitter BCz-BN, which exhibits a EQEmax of 29.8% and low efficiency roll-off.
Introduction
Hole-transporting materials (HTMs) play an important role in organic light emitting diode (OLED) devices because of their hole transportation and electron blocking ability in the interfacial region between the hole-transporting layer (HTL) and the light-emitting layer.1–6 In general, HTMs need to possess high hole mobility, good morphological stability, and an appropriate highest occupied molecular orbital (HOMO) level to ensure a low energy barrier for hole injection to the emitting layer and a suitable lowest unoccupied molecular orbital (LUMO) level to block electron injection from the emitting layer to the HTL.2,7–11 Triarylamine derivatives are the most widely used HTMs due to their excellent optoelectronic properties.12–18 Among them, 1,1-bis(4-N,N-ditolylaminophenyl)cyclohexane (TAPC) is one of the most classical HTMs, owing to its high hole mobility (μh), high transparency for visible light, and high triplet energies (ET) (2.9 eV) for blocking triplet excitons. However, TAPC exhibits a low glass transition temperature (Tg) of 74 °C, lacking proper morphological stability.19,20
The 9,9′-spirobifluorene (SBF) structure possesses excellent thermal stability with high decomposition temperature (Td), high Tg, high ET, and well-matched frontier molecular orbital (FMO) energy levels with adjacent layers.21–23 Therefore, SBF-based organic optoelectronic materials have been widely applied in the field of organic light-emitting diodes (OLEDs), perovskite solar cells, and organic lasers.24–29 In recent years, SBF-based HTMs have also been extensively explored.1,2,30–33 The C2 and C7 positions of SBF have the highest electrophilic reactivity.34 As a result, a considerable amount of research on HTMs is focused on C2 and C7-substituted SBF derivatives.30–33,35–38 However, the C2 and C7-substitutions have been noted to reduce the ET of SBF derivatives due to the electronic coupling between electron-donating groups at C2 and/or C7-positions and the biphenyl of fluorene via a para-linkage mode.31,39 For example, the introduction of arylamines at the C2 and C7 positions results in ET values of 2.31 and 2.29 eV for the compounds, compared to 2.88 eV for SBF.31,39
In this work, we design and synthesize a series of C3 and C6-substituted SBF derivatives containing two or four di-4-tolylamino groups, respectively, including 3,3′,6,6′-tetra(N,N-ditolylamino)-9,9′-spirobifluorene (3,3′,6,6′-TDTA-SBF), 3,3′-di(N,N-ditolylamino)-9,9′-spirobifluorene (3,3′-DDTA-SBF), and 3,6-di(N,N-ditolylamino)-9,9′-spirobifluorene (3,6-DDTA-SBF) (Scheme 1). These SBF-based triarylamine derivatives present high ET and wide HOMO/LUMO differences owing to the electronic decoupling between electron-donating di-4-tolylamino groups at C3 and/or C6-positions and the biphenyl of fluorene via a meta-linkage mode, and are therefore suitable as HTMs for red, green, and blue (RGB) OLEDs. Moreover, SBF endows these HTMs with good thermal stability and excellent hole-transporting properties, beneficial for the assembly of high-performance OLEDs.
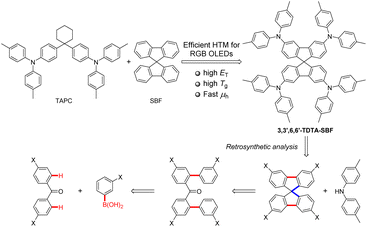 |
| Scheme 1 Molecular design concept and retrosynthetic analysis. | |
Results and discussion
Synthesis and characterization
Chelation-assisted transition metal-catalyzed ortho-C–H arylation has emerged as a powerful method to accomplish aryl–aryl coupling.40–46 Retrosynthetic analysis indicates that 3,3′,6,6′-TDTA-SBF can be prepared through a Buchwald–Hartwig amination of 3,3′,6,6′-tetrahalogenated SBF with di-p-tolylamine (Scheme 1). 3,3′,6,6′-Tetrahalogenated SBF can be synthesized by chelation-assisted transition metal-catalyzed ortho-C–H diarylation of 4,4′-dihalogenated benzophenone, followed by an intramolecular Friedel–Crafts spiroannulation reaction (Scheme 1). Therefore, ortho-C–H diarylation was initially explored by a model reaction of benzophenone with phenylboronic acid under palladium catalysis. However, the diarylated product was not observed under the Pd-catalyzed conditions. Fortunately, when employing benzophenone O-methyl oxime and phenylboronic acid pinacol ester as substrates, the diarylated product 3a could be obtained in a yield of 53% (Table S1,† entry 1). Further optimization of reaction conditions was carried out. Finally, 3a was afforded in 71% yield in the presence of 10 mol% of Pd(dba)2, 20 mol% of N-acetyl-L-phenylalanine as a ligand, and 1.5 equiv. of Ag2CO3 as an oxidant in 1,1,1,3,3,3-hexafluoro-2-propanol (HFIP) solvent at 100 °C for 36 h under a nitrogen atmosphere (Table S1,† entry 4).
Adopting optimized reaction conditions, the chelation-assisted Pd-catalyzed ortho-C–H diarylation of bis(4-chlorophenyl)methanone O-methyl oxime (1b) with meta-methoxyphenylboronic acid pinacol ester produced 3b in 62% yield (Scheme 2). Then, methane sulfonic acid (MsOH)-promoted intramolecular Friedel–Crafts alkylation provided the key 3,3′,6,6′-tetrasubsitituted SBF intermediate 4 in 82% yield. The yield of side reactions occurring at the ortho-position of the methoxy group is very low. The Buchwald–Hartwig amination of 4 with di-p-tolylamine gave 5 in 84% yield. 5 underwent a deprotection of methyl ether in the presence of BBr3 and then an esterification reaction using trifluoromethanesulfonic anhydride (Tf2O) to deliver 6 in 77% yield. Buchwald–Hartwig amination was once again performed to produce 3,3′,6,6′-TDTA-SBF in 75% yield. The triflate group was reductively removed from the SBF core of 6 catalyzed by palladium, yielding 3,3′-DDTA-SBF in 88% yield. Subsequently, 3,6-dibromo-SBF was prepared through the nucleophilic addition of 3,6-dibromo-9H-fluoren-9-one by using 2-iodo-1,1′-biphenyl in the presence of iPrMgCl and a subsequent intramolecular Friedel–Crafts alkylation reaction, which underwent a Buchwald–Hartwig amination to afford 3,6-DDTA-SBF (Scheme 2). The structures of the three compounds were confirmed by 1H NMR, 13C NMR and high-resolution mass spectrometry (HRMS). The structure of 3,6-DDTA-SBF was further confirmed by its single crystal X-ray data. In the single crystal X-ray structure, 3,6-DDTA-SBF molecules mainly adopt an edge-to-face packing mode via multiple intermolecular C–H⋯π interaction networks, accompanied by a weak π⋯π interaction with 3.69 Å between the two benzene rings of the intermolecular di-4-tolylamino group (Fig. S1†). Unfortunately, attempts to acquire single crystals of 3,3′,6,6′-TDTA-SBF were unsuccessful as a result of its low crystallinity.
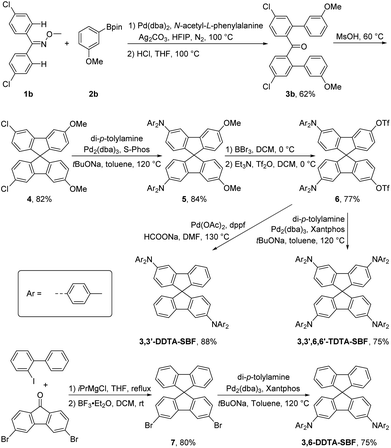 |
| Scheme 2 Synthesis of 3,3′,6,6′-TDTA-SBF, 3,3′-DDTA-SBF and 3,6-DDTA-SBF. | |
Theoretical calculations
Density functional theory (DFT) and time-dependent DFT (TD-DFT) calculations were performed at the B3LYP/6-31G(d) level (Fig. 1). 3,3′,6,6′-TDTA-SBF is essentially composed of four triarylamines through the connections of two Csp2–Csp2 σ-bonds and a quaternary carbon core. Therefore, its HOMO is distributed on all twelve benzene rings and four nitrogen atoms of the four triarylamines due to the highly symmetrical characteristics, while its LUMO is mainly focused on one of the two fluorene units. Similarly, the HOMO of 3,3′-DDTA-SBF is distributed on two triphenylamines, while its LUMO is extended to the two fluorene units of the SBF core. The HOMO of 3,6-DDTA-SBF is also distributed on two triphenylamines, and its LUMO is mainly localized on the fluorene substituted by the di-4-tolylamino group. Calculation results indicate that the three compounds possess similar HOMO energy levels of −4.56 eV for 3,3′,6,6′-TDTA-SBF, −4.70 eV for 3,3′-DDTA-SBF and −4.70 eV for 3,6-DDTA-SBF, while the LUMO energy levels were calculated to be −0.77, −0.78 and −0.81 eV for 3,3′,6,6′-TDTA-SBF, 3,3′-DDTA-SBF and 3,6-DDTA-SBF, respectively. Accordingly, these compounds all exhibit large energy gaps (Eg) of about 3.9 eV. Moreover, the ET1 value was calculated to be 2.74 eV for 3,3′,6,6′-TDTA-SBF, 2.83 eV for 3,3′-DDTA-SBF and 2.75 eV for 3,6-DDTA-SBF. The high ET1 is a key property to prevent leakage of triplet excitons from the emitting layer to the HTL, especially for the blue emitting OLED.
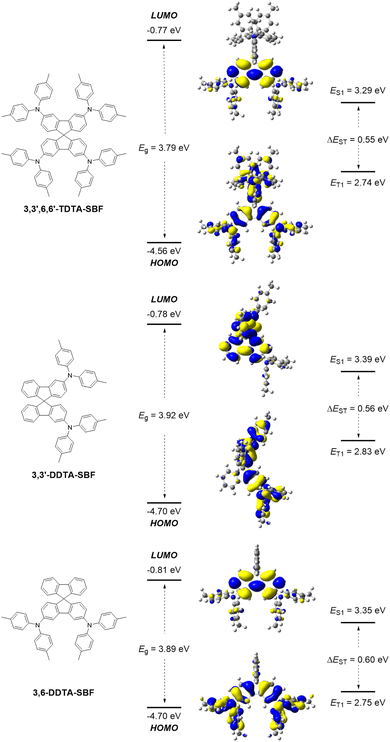 |
| Fig. 1 Frontier orbital distributions and energy level alignments of 3,3′,6,6′-TDTA-SBF, 3,3′-DDTA-SBF and 3,6-DDTA-SBF. | |
Electronic and physical properties
The photophysical properties of these SBF-based triarylamine derivatives were studied. The strong absorption peaks at 310–313 nm correspond to π–π* transitions, which represent the characteristic absorption of the SBF scaffold (Fig. 2a and Table 1). The weak absorption bands at 350–400 nm may be attributed to n–π* transitions (Fig. 2a). The optical band gaps (Eg) of 3,3′,6,6′-TDTA-SBF, 3,3′-DDTA-SBF and 3,6-DDTA-SBF were calculated from the onset of their absorption spectra to be 3.07 eV, 3.12 eV and 3.09 eV, respectively (Table 1). Their maximum emission wavelengths are at 401–402 nm (Fig. 2b and Table 1). Despite having different numbers of diarylamino substituents and different substituted sites, the absorption and emission spectra of the three compounds are almost identical, probably owing to the electronic decoupling of the meta-connection mode in both the ground and excited states. The phosphorescence emission spectra measured at 77 K in toluene provide the corresponding ET1 of 2.66 eV, 2.75 eV and 2.68 eV for 3,3′,6,6′-TDTA-SBF, 3,3′-DDTA-SBF and 3,6-DDTA-SBF, respectively (Fig. 2c and Table 1).
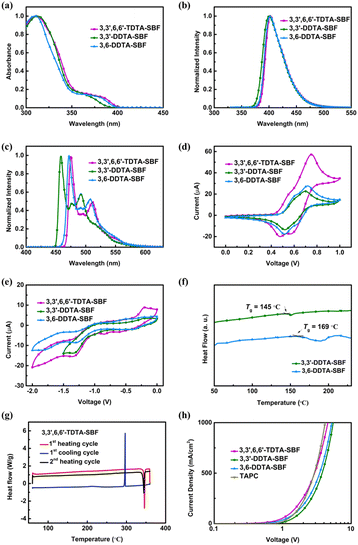 |
| Fig. 2 (a) UV-vis absorption spectra, (b) emission spectra at 298 K, (c) phosphorescence spectra at 77 K in toluene, and cyclic voltammetry (CV) data (d) in oxidation and (e) in reduction: CH2Cl2/[NBu4PF6] 0.1 M of 3,3′,6,6′-TDTA-SBF, 3,3′-DDTA-SBF and 3,6-DDTA-SBF. (f) DSC curves of 3,3′-DDTA-SBF and 3,6-DDTA-SBF. (g) DSC curves of 3,3′,6,6′-TDTA-SBF. (h) The current density–voltage (J–V) curves using 3,3′,6,6′-TDTA-SBF-, 3,3′-DDTA-SBF-, 3,6-DDTA-SBF- and TAPC-based hole-only devices (HODs). | |
Table 1 Electronic and physical properties
Property |
3,3′,6,6′-TDTA-SBF
|
3,3′-DDTA-SBF
|
3,6-DDTA-SBF
|
Measured in toluene solution (1.0 × 10−5 M), where λabs is the absorption peak and λem is the photoluminescence peak at room temperature.
Calculated from the onset of the absorption spectra.
Calculated from the first phosphorescence peak at 77 K in toluene.
Calculated from CV curves with ferrocene as the internal standard using the formulae EHOMO = −[Eox − E(Fc/Fc+) + 4.8] eV and ELUMO = −[Ered − E(Fc/Fc+) + 4.8] eV.
Hole mobility (μh). TAPC: μh = 3.84 × 10−2 cm2 V−1 s−1.
|
λ
abs
[nm] |
311 |
313 |
310 |
λ
em
[nm] |
402 |
401 |
401 |
E
g
[eV] |
3.07 |
3.12 |
3.09 |
E
T1
[eV] |
2.66 |
2.75 |
2.68 |
T
g [°C] |
— |
145 |
169 |
T
d [°C] |
506 |
430 |
413 |
HOMOd [eV] |
−5.20 |
−5.19 |
−5.20 |
LUMOd [eV] |
−3.08 |
−3.16 |
−3.07 |
μ
h
[cm2 V−1 S−1] |
3.83 × 10−2 |
1.61 × 10−2 |
2.53 × 10−2 |
Electrochemical investigations were performed by cyclic voltammetry (CV) in CH2Cl2 solution (Fig. 2d and e). The HOMO levels were measured at −5.20 eV for 3,3′,6,6′-TDTA-SBF, −5.19 eV for 3,3′-DDTA-SBF and −5.20 eV for 3,6-DDTA-SBF (Table 1). These values provide advantages for hole injection into the emitting layer. Additionally, the LUMO levels were measured at −3.08 eV for 3,3′,6,6′-TDTA-SBF, −3.16 eV for 3,3′-DDTA-SBF and −3.07 eV for 3,6-DDTA-SBF (Table 1). The shallow LUMO level would help to prevent electrons from escaping the emission layer. Subsequently, their Td were detected by thermogravimetric analysis (TGA) at a rate of 10 °C min−1 under a nitrogen atmosphere, and the Tg was measured by differential scanning calorimetry (DSC) under a nitrogen atmosphere (Fig. 2f and g). Benefiting from the rigid and orthogonal conjugated skeleton of the SBF core, these compounds exhibit excellent thermal stabilities with a very high Td of 506 °C, 430 °C and 413 °C for 3,3′,6,6′-TDTA-SBF, 3,3′-DDTA-SBF and 3,6-DDTA-SBF, respectively (Table 1). The DSC measurement, performed in the temperature range from 50 °C to 260 °C, demonstrates that 3,3′-DDTA-SBF and 3,6-DDTA-SBF possess high Tg, which is 145 °C and 169 °C, respectively (Table 1). However, the Td of 3,3′,6,6′-TDTA-SBF was not observed to be in this temperature range. Therefore, the DSC measurement of 3,3′,6,6′-TDTA-SBF was subsequently performed in a larger temperature range from 50 °C to 360 °C. The experimental result indicates that 3,3′,6,6′-TDTA-SBF melts at 346 °C. As shown in Fig. 2f, before the sample completely melts, a rather smooth DSC curve is observed without any clue of glass transition or other phase transformation, demonstrating excellent morphological stability.
Subsequently, the hole transport properties of these SBF-based triarylamine derivatives were investigated. A hole-only device (HOD) was fabricated according to the device architecture of ITO/HAT-CN (10 nm)/HTM (120 nm)/HAT-CN (10 nm)/Al (100 nm), which was employed as both the hole injection and electron-blocking layer. The HOD of TAPC was also fabricated for comparison. We use the space-charge-limited current (SCLC) method to calculate the μh values (Fig. S3†). All three compounds exhibit excellent hole-transporting properties, with μh values of 3.83 × 10−2 cm2 V−1 s−1, 1.61 × 10−2 cm2 V−1 s−1 and 2.53 × 10−2 cm2 V−1 s−1 for 3,3′,6,6′-TDTA-SBF, 3,3′-DDTA-SBF and 3,6-DDTA-SBF, respectively (Fig. 3). These values are comparable to that of TAPC (3.84 × 10−2 cm2 V−1 s−1, Fig. 2h), which is well-known for its excellent hole-transporting properties in OLED HTMs.
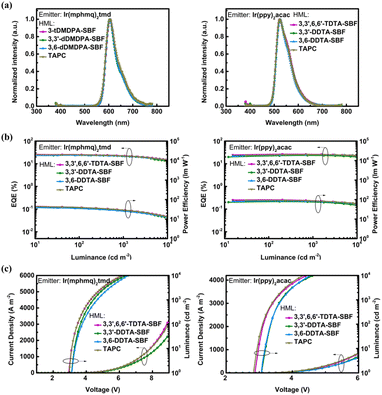 |
| Fig. 3 OLED device performances. (a) Electroluminescence spectra at a luminance of 1000 cd m−2, (b) luminance and current density versus voltage curves, and (c) EQE and power efficiency versus luminance curves of red (left) and green (right) PhOLED devices. | |
Electroluminescence performances
The device performance of 3,3′,6,6′-TDTA-SBF, 3,3′-DDTA-SBF and 3,6-DDTA-SBF as HTMs was further investigated in phosphorescent OLEDs (PhOLEDs). The performances are summarized in Fig. 3, S4, S5† and Tables 2, S1.† The optimized HTM thickness is 30 nm (Fig. S5 and Table S3†). First, bis[4-methyl-2-(3,5-dimethylphenyl)quinoline]tetramethyl heptadionate iridium(III) (Ir(mphmq)2(tmd)) was selected as a red phosphor and 4,4′-bis(9H-carbazol-9-yl)biphenyl (CBP) as a host. The optimized red PhOLED device structure was ITO/HAT-CN (10 nm)/HTM (25 nm)/TCTA (10 nm)/CBP: 1 wt% Ir(mphmq)2tmd (10 nm)/TmPyPB (50 nm)/LiF (0.8 nm)/Al (100 nm). In these devices, HAT-CN and LiF were used as the hole injection and electron injection materials, respectively, 4,4′,4′′-tris(9H-carbazol-9-yl)triphenylamine (TCTA) as an exciton-blocking material, and 1,3,5-tri(m-pyrid-3-ylphenyl)benzene (TmPyPB) as an electron-transporting material (ETM). The molecular structure and the corresponding PhOLED device structure are shown in Fig. S4.† The devices which employed 3,3′,6,6′-TDTA-SBF, 3,3′-DDTA-SBF and 3,6-DDTA-SBF as HTMs exhibit excellent performances with maximum external quantum efficiencies (EQEmax) of 26.1%, 24.0% and 23.9%, respectively, as well as low efficiency roll-off (Fig. 3 and Table 2). Under the same device fabricating conditions, the PhOLED based on TAPC as the HTM exhibits a relatively lower device efficiency with an EQEmax of 25.1% than that of 3,3′,6,6′-TDTA-SBF.
Table 2 Summary of OLED performance
Emitter |
HTM |
ELpeak [nm] |
V
on
[V] |
CIEb [x, y] |
EQEmax/1000/5000c [%] |
PEmax/1000/5000d [lm W−1] |
Turn-on voltage.
Commission Internationale de I'Eclairage (CIE).
Maximum external quantum efficiency and external quantum efficiencies at a luminance of 1000 cd m−2 and 5000 cd m−2.
Maximum power efficiency and power efficiencies at a luminance of 1000 cd m−2 and 5000 cd m−2.
|
Ir(mphmq)2tmd |
3,3′,6,6′-TDTA-SBF
|
605 |
3.1 |
[0.63, 0.36] |
26.1/22.3/17.8 |
46.3/28.6/18.1 |
3,3′-DDTA-SBF
|
605 |
3.2 |
[0.63, 0.37] |
23.9/21.7/15.5 |
41.5/28.0/15.7 |
3,6-DDTA-SBF
|
605 |
3.3 |
[0.63, 0.37] |
24.0/21.1/17.1 |
39.2/25.2/16.5 |
TAPC |
605 |
3.1 |
[0.63, 0.37] |
25.1/22.1/17.0 |
44.9/28.1/17.6 |
Ir(ppy)2acac |
3,3′,6,6′-TDTA-SBF
|
524 |
3.0 |
[0.32, 0.63] |
26.4/26.0/24.3 |
97.6/87.1/71.4 |
3,3′-DDTA-SBF
|
525 |
3.2 |
[0.32, 0.63] |
23.2/23.2/21.6 |
78.6/74.2/61.0 |
3,6-DDTA-SBF
|
525 |
3.2 |
[0.32, 0.64] |
25.0/25.0/24.3 |
85.2/80.4/68.9 |
TAPC |
523 |
3.0 |
[0.32, 0.63] |
25.4/25.3/24.3 |
92.6/84.8/71.3 |
Flrpic |
3,3′,6,6′-TDTA-SBF
|
471 |
3.1 |
[0.16, 0.36] |
25.4/21.4/19.8 |
52.6/31.4/24.6 |
3,3′-DDTA-SBF
|
473 |
3.3 |
[0.16, 0.37] |
23.9/21.0/18.6 |
48.0/31.1/23.4 |
3,6-DDTA-SBF
|
472 |
3.3 |
[0.16, 0.38] |
25.0/21.8/18.1 |
51.1/31.5/21.6 |
TAPC |
474 |
3.1 |
[0.16, 0.37] |
24.8/20.0/17.5 |
51.5/30.3/22.5 |
BCz-BN |
3,3′,6,6′-TDTA-SBF
|
487 |
3.0 |
[0.10, 0.35] |
29.8/24.0/19.2 |
53.4/29.6/19.7 |
3,3′-DDTA-SBF
|
486 |
3.2 |
[0.11, 0.35] |
24.9/20.5/16.8 |
42.0/23.8/16.0 |
3,6-DDTA-SBF
|
486 |
3.2 |
[0.11, 0.34] |
25.7/20.9/17.0 |
42.3/23.9/16.0 |
TAPC |
487 |
3.1 |
[0.10, 0.36] |
28.7/20.9/17.2 |
50.5/25.4/17.4 |
The green PhOLED devices were fabricated employing bis(2-phenylpyridine)iridium(III)-acetylacetonate (Ir(ppy)2(acac)) as a green phosphor and CBP as the host material. Using HAT-CN as a hole injection layer led to a lower device efficiency, and therefore, the optimized green PhOLED device structure was ITO/HTM (30 nm)/TCTA (10 nm)/CBP: 10 wt% Ir(ppy)2acac (20 nm)/TmPyPB (40 nm)/LiF (0.8 nm)/Al (100 nm) (Fig. S4†). 3,3′,6,6′-TDTA-SBF, 3,3′-DDTA-SBF and 3,6-DDTA-SBF all show excellent performance as the HTMs of green PhOLEDs with an EQEmax of 26.4%, 25.0% and 23.2%, respectively (Fig. 3 and Table 2). Notably, 3,3′,6,6′-TDTA-SBF as a hole transport layer exhibits extremely low device efficiency roll-off, and the EQE at 1000 cd m−2 maintained 98% of its peak value. For comparison, the PhOLED device using TAPC instead of 3,3′,6,6′-TDTA-SBF as the hole transport layer was fabricated under identical conditions, which shows a relatively lower EQEmax of 25.4%.
The existing SBF-based triarylamine derivatives have rarely been used as HTMs for blue PhOLED devices due to their low ET. A hole HTM with an ET value is crucial for blue PhOLEDs, as it prevents triplet energy from transferring back from the blue emitter to the neighboring hole-transporting layer.47–50 The SBF-based triarylamine derivatives herein developed possess high triplet energy levels with ET1 from 2.66 eV to 2.75 eV. Therefore, the blue PhOLED devices were fabricated employing bis(3,5-difluoro-2-(2-pyridyl)phenyl)-2-carboxypyridyl iridium(III) (FIrpic) as a blue phosphor, 3,3′-di(9H-carbazol-9-yl)-1,1′-biphenyl (mCBP) as the host material, and 3,3′,6,6′-TDTA-SBF, 3,3′-DDTA-SBF or 3,6-DDTA-SBF as the HTM, respectively. The blue PhOLED device using TAPC as the HTM was also fabricated as a comparison. The optimized blue PhOLED device structure was ITO/HTM (30 nm)/TCTA (10 nm)/mCBP: 15 wt% Flrpic (23 nm)/TmPyPB (40 nm)/LiF (0.8 nm)/Al (100 nm) (Fig. S4†). Among all four tested HTMs, including TAPC, the blue PhOLED device using 3,3′,6,6′-TDTA-SBF as the HTM exhibits the best performance with an EQEmax of 25.4% and low efficiency roll-off (Fig. 4 and Table 2). At luminance levels of 1000 cd m−2 and 5000 cd m−2, the device efficiencies are well-preserved at 21.4% and 19.8%, respectively.
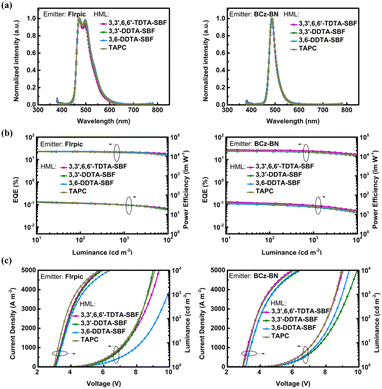 |
| Fig. 4 OLED device performances. (a) Electroluminescence spectra at a luminance of 1000 cd m−2, (b) luminance and current density versus voltage curves, and (c) EQE and power efficiency versus luminance curves of blue PhOLED (left) devices and narrowband-emitting BN-MR OLED (right) devices. | |
In recent years, boron–nitrogen-based multiple resonance (BN-MR) emitters have shown great potential for application in the next generation of wide-colour gamut OLED displays with ultrahigh resolution (UHD) due to their exceptionally narrowband emissions. Among these, BCz-BN is one of the most classical BN-MR emitters. In order to explore the extensive possibility of these SBF-based triarylamine derivatives as HTMs, we fabricated OLED devices based on BCz-BN as the emitter, mCBP as the host, Flrpic as the sensitizer, and 3,3′,6,6′-TDTA-SBF, 3,3′-DDTA-SBF and 3,6-DDTA-SBF as the HTMs, respectively. An OLED device using TAPC as the HTM was also fabricated for comparison. The optimized BN-MR emitter-based OLED device structure was ITO/HML (30 nm)/TCTA (10 nm)/mCBP: 15 wt% Flrpic: 0.5 wt% BCz-BN (23 nm)/TmPyPB (40 nm)/LiF (0.8 nm)/Al (100 nm) (Fig. S4†). The OLED device using 3,3′,6,6′-TDTA-SBF as the HTL achieves an EQEmax of 29.8% and low efficiency roll-off (Fig. 4 and Table 2), revealing the great potential of these molecules as universal HTMs for the assembly of blue OLEDs.
Conclusions
In summary, we have developed a highly efficient palladium catalyst system to conduct the diarylation of benzophenone O-methyl oxime derivatives, which provides convenience for the development of 3,3′,6,6′-tetrasubstituted SBF derivatives. Based on this rapid and concise synthetic strategy, 3,3′,6,6′-TDTA-SBF and 3,3′-DDTA-SBF were successfully obtained. For comparison, 3,6-DDTA-SBF is prepared by a classical route. Further investigation on electronic and physical properties as well as electroluminescence performances indicates that 3,3′,6,6′-TDTA-SBF not only possesses high ET and Tg values but also fast μh, enabling the assembly of high-performance RGB PhOLEDs with an EQEmax of 26.1%, 26.4%, and 25.4%, respectively, as well as narrowband blue OLEDs with an EQEmax of 29.8%. This work not only introduces a universal HTM based on the 3,6-substituted SBF skeleton for assembling high-performance RGB OLEDs but also highlights the effectiveness of the C–H activation method in discovering high-performance organic functional materials.
Data availability
Data are available in the article and ESI.†
Author contributions
Q. Li carried out most parts of the experiments. Y. Guo performed some of the synthesis. J. Lan designed and directed the project. J. Lan, D. Wu, Y. Yang and Z. Bin wrote and modified the manuscript. All authors contributed to discussions.
Conflicts of interest
There are no conflicts to declare.
Acknowledgements
We acknowledge financial support from the National Key R&D Program of China (No. 2021YFA1500100), National Natural Science Foundation of China (No. 22371196, 22071162, 22171188, and 22275127), Natural Science Foundation of Sichuan, China (2022NSFSC0029), and Fundamental Research Funds for the Central Universities. We are also thankful for the support of associate researcher Shaolan Wang from the Analytical and Testing Center of Sichuan University and Comprehensive Training Platform Specialized Laboratory, College of Chemistry, Sichuan University.
Notes and references
- S. Jhulki and J. N. Moorthy, J. Mater. Chem. C, 2018, 6, 8280–8325 RSC.
- Shahnawaz, S. S. Swayamprabha, M. R. Nagar, R. A. K. Yadav, S. Gull, D. K. Dubey and J.-H. Jou, J. Mater. Chem. C, 2019, 7, 7144–7158 RSC.
- Q. Huang, G. A. Evmenenko, P. Dutta, P. Lee, N. R. Armstrong and T. J. Marks, J. Am. Chem. Soc., 2005, 127, 10227–10242 CrossRef CAS PubMed.
- S. Chen, J. Wei, K. Wang, C. Wang, D. Chen, Y. Liu and Y. Wang, J. Mater. Chem. C, 2013, 1, 6594–6602 RSC.
- H. Je, S. Cho, N. Y. Kwon, D. W. Lee, M. J. Cho and D. H. Choi, ACS Appl. Mater. Interfaces, 2022, 14, 35969–35977 CrossRef CAS PubMed.
- S. Kakumachi, T. B. Nguyen, H. Nakanotani and C. Adachi, Chem. Eng. J., 2023, 471, 144516 CrossRef CAS.
- D. E. Loy, B. E. Koene and M. E. Thompson, Adv. Funct. Mater., 2002, 12, 245–249 CrossRef CAS.
- M. S. Park and J. Y. Lee, Chem. Mater., 2011, 23, 4338–4343 CrossRef CAS.
- C. Peng, H. Liu, X. Han, F. Zhang, S. Wang and X. Li, J. Mater. Chem. C, 2022, 10, 14471–14479 RSC.
- V. G. Sree, C. Bathula, H.-K. Youi, H.-S. Kim, J. I. Sohn and H. Im, J. Mol. Liq., 2021, 338, 116708 CrossRef.
- M. R. Nagar, A. Choudhury, D. Tavgeniene, R. Beresneviciute, D. Blazevicius, V. Jankauskas, K. Kumar, S. Banik, S. Ghosh, S. Grigalevicius and J.-H. Jou, J. Mater. Chem. C, 2022, 10, 3593–3608 RSC.
- K. Kutonova, B. Ebenhoch, L. G. von Reventlow, S. Heißler, L. Rothmann, S. Bräse and A. Colsmann, J. Mater. Chem. C, 2020, 8, 16498–16505 RSC.
- Q.-X. Tong, S.-L. Lai, M.-Y. Chan, K.-H. Lai, J.-X. Tang, H.-L. Kwong, C.-S. Lee and S.-T. Lee, Chem. Mater., 2007, 19, 5851–5855 CrossRef CAS.
- Z. Jiang, T. Ye, C. Yang, D. Yang, M. Zhu, C. Zhong, J. Qin and D. Ma, Chem. Mater., 2011, 23, 771–777 CrossRef CAS.
- M. Bender, K. M. Schelkle, N. Jürgensen, S. Schmid, G. Hernandez-Sosa and U. H. F. Bunz, Macromolecules, 2016, 49, 2957–2961 CrossRef CAS.
- N. Nagamura, H. Sasabe, H. Sato, T. Kamata, N. Ito, S. Araki, S. Abe, Y. Sukegawa, D. Yokoyama, H. Kaji and J. Kido, J. Mater. Chem. C, 2022, 10, 8694–8701 RSC.
- X. Zhang, H. Yan, X. Zhang and H. Meng, Sci. China Mater., 2024, 67, 2767–2777 CrossRef CAS.
- K.-K. Tan, W.-L. Zhao, C.-H. Guo, W.-C. Guo, M. Li and C.-F. Chen, Chem. Eng. J., 2024, 482, 149080 CrossRef CAS.
- P. M. Borsenberger, L. Pautmeier, R. Richert and H. Bässler, J. Chem. Phys., 1991, 94, 8276–8281 CrossRef CAS.
- M. C. Suh, B. D. Chin, M.-H. Kim, T. M. Kang and S. T. Lee, Adv. Mater., 2003, 15, 1254–1258 CrossRef CAS.
- Q. Li, Z. Yu, Q. Liu, Y. Guo, Z. Fu, Y. Yang, Z. Bin, D. Wu and J. Lan, Chem. Sci., 2024, 15, 10547–10555 RSC.
- H. Cheng, J. Lan, Y. Yang and Z. Bin, Mater. Horiz., 2024 10.1039/d4mh00634h.
- Y. Luo, Z. Liu, G. Yang, T. Wang, Z. Bin, J. Lan, D. Wu and J. You, Angew. Chem., Int. Ed., 2021, 60, 18852–18859 CrossRef CAS PubMed.
- Y. Tao, C. Yang and J. Qin, Chem. Soc. Rev., 2011, 40, 2943–2970 RSC.
- X. Yang, G. Zhou and W.-Y. Wong, Chem. Soc. Rev., 2015, 44, 8484–8575 RSC.
- S. Gangala and R. Misra, J. Mater. Chem. A, 2018, 6, 18750–18765 RSC.
- Y. Jiang, Y.-Y. Liu, X. Liu, H. Lin, K. Gao, W.-Y. Lai and W. Huang, Chem. Soc. Rev., 2020, 49, 5885–5944 RSC.
- Y.-K. Qu, Q. Zheng, J. Fan, L.-S. Liao and Z.-Q. Jiang, Acc. Mater. Res., 2021, 2, 1261–1271 CrossRef CAS.
- Y. Chen, J. Xu and P. Gao, Org. Chem. Front., 2024, 11, 508–539 RSC.
- Ö. Usluer, J. Mater. Chem. C, 2014, 2, 8098–8104 RSC.
- R. Braveenth, H. W. Bae, Q. P. B. Nguyen, H. M. Ko, C. H. Lee, H. J. Kim, J. H. Kwon and K. Y. Chai, Molecules, 2017, 22, 464 CrossRef PubMed.
- Z. Jiang, H. Yan, X. Zhang, Z. Meng, C. Kuang, X. Zhang, Y. He, Y. Zhu and H. Meng, Adv. Opt. Mater., 2023, 11, 2301014 CrossRef CAS.
- O. Usluer, S. Demic, D. A. M. Egbe, E. Birckner, C. Tozlu, A. Pivrikas, A. M. Ramil and N. S. Sariciftci, Adv. Funct. Mater., 2010, 20, 4152–4161 CrossRef CAS.
- T. P. I. Saragi, T. Spehr, A. Siebert, T. Fuhrmann-Lieker and J. Salbeck, Chem. Rev., 2007, 107, 1011–1065 CrossRef CAS PubMed.
- L. Caliò, M. Salado, S. Kazim and S. Ahmad, Joule, 2018, 2, 1800–1815 CrossRef.
- N. J. Jeon, H. Na, E. H. Jung, T.-Y. Yang, Y. G. Lee, G. Kim, H.-W. Shin, S. I. Seok, J. Lee and J. Seo, Nat. Energy, 2018, 3, 682–689 CrossRef CAS.
- Y. Ren, Y. Wei, T. Li, Y. Mu, M. Zhang, Y. Yuan, J. Zhang and P. Wang, Energy Environ. Sci., 2023, 16, 3534–3542 RSC.
- X. Liu, B. Ding, M. Han, Z. Yang, J. Chen, P. Shi, X. Xue, R. Ghadari, X. Zhang, R. Wang, K. Brooks, L. Tao, S. Kinge, S. Dai, J. Sheng, P. J. Dyson, M. K. Nazeeruddin and Y. Ding, Angew. Chem., Int. Ed., 2023, 135, e202304350 CrossRef.
- L. Sicard, C. Quinton, J.-D. Peltier, D. Tondelier, B. Geffroy, U. Biapo, R. Métivier, O. Jeannin, J. Rault-Berthelot and C. Poriel, Chem.–Eur. J., 2017, 23, 7719–7727 CrossRef CAS PubMed.
- Y.-F. Zhang and Z.-J. Shi, Acc. Chem. Res., 2019, 52, 161–169 CrossRef CAS PubMed.
- Y. Yang, Y. Wu, Z. Bin, C. Zhang, G. Tan and J. You, J. Am. Chem. Soc., 2024, 146, 1224–1243 CrossRef CAS PubMed.
- Z. Huang, Z. Bin, R. Su, F. Yang, J. Lan and J. You, Angew. Chem., Int. Ed., 2020, 59, 9992–9996 CrossRef CAS PubMed.
- Z. Huang, B. Lei, D. Yang, D. Ma, Z. Bin and J. You, Angew. Chem., Int. Ed., 2022, 61, e202213157 CrossRef CAS PubMed.
- F. Wang, L. Zhang, W. Han, Z. Bin and J. You, Angew. Chem., Int. Ed., 2022, 61, e202205380 CrossRef CAS PubMed.
- Z. Lin, J. C. A. Oliveira, A. Scheremetjew and L. Ackermann, J. Am. Chem. Soc., 2024, 146, 228–239 CrossRef CAS PubMed.
- C. Jacob, J. Annibaletto, J. Peng, R. Bai, B. U. W. Maes, Y. Lan and G. Evano, Angew. Chem., Int. Ed., 2024, 63, e202403553 CrossRef CAS PubMed.
- J.-H. Jou, T.-H. Li, S. Kumar, C.-C. An, A. Agrawal, S.-Z. Chen, P.-H. Fang, G. Krucaite, S. Grigalevicius, J. Grazulevicius and C.-F. Sung, Org. Electron., 2015, 24, 254–262 CrossRef CAS.
- W. Jiang, X. Ban, M. Ye, Y. Sun, L. Duan and Y. Qiu, J. Mater. Chem. C, 2015, 3, 243–246 RSC.
- H. Fukagawa, T. Shimizu, H. Kawano, S. Yui, T. Shinnai, A. Iwai, K. Tsuchiya and T. Yamamoto, J. Phys. Chem. C, 2016, 120, 18748–18755 CrossRef CAS.
- S. H. Jeong, H. J. Jang and J. Y. Lee, J. Ind. Eng. Chem., 2019, 78, 324–329 CrossRef CAS.
Footnote |
† Electronic supplementary information (ESI) available: Experimental details, crystallographic data, and photophysical and thermal performances of the compounds. CCDC 2370368 (3,6-DDTA-SBF). For ESI and crystallographic data in CIF or other electronic format see DOI: https://doi.org/10.1039/d4sc04979a |
|
This journal is © The Royal Society of Chemistry 2024 |